全文HTML
--> --> -->钙钛矿太阳能电池的光电转换过程主要包括电荷载流子的产生、分离、输运和复合等物理过程. 主要研究方法是通过测量器件的电流-电压响应、光谱学等间接方式来分析和推测电荷载流子的动力学行为, 仍然缺少对器件内部的直接观测和分析研究. 直接测量分析太阳能电池器件内部的空间电势, 并阐明不同光照、外加电场等条件下空间电势与电荷载流子微观过程的内在联系, 可以深入揭示钙钛矿太阳能电池的光电转换规律, 从而为该领域的进一步发展提供必要的科学研究基础. 开尔文探针力显微镜技术(图1[19])是无损表征器件空间电势分布的重要方法, 它并不直接接触样品表面, 测试时也可以在真空环境下进行, 可以方便地测试样品截面的空间电势情况, 成为揭示钙钛矿太阳能电池工作机理的有力工具[20].
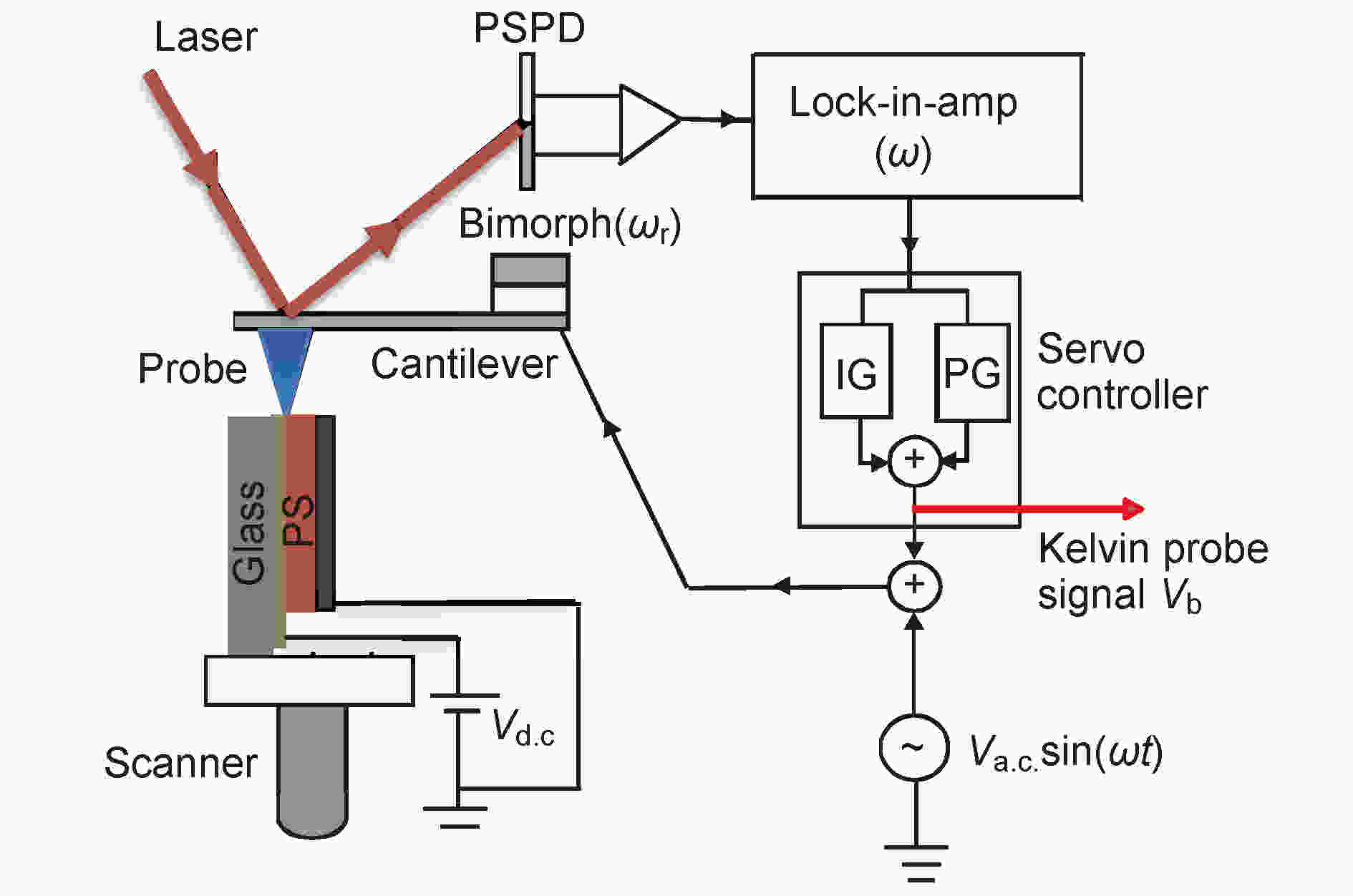
Figure1. Illustration of Kelvin probe force microscopy[19].
开尔文探针力显微镜技术将测试接触电势差的开尔文方法与现代扫描探针显微镜技术相结合, 对器件内部电势进行表征, 具有纳米级分辨率, 无损测试等优点. 在开尔文方法中[21], 两个间距很小的导体, 形成平行板电容器, 通过施加交流电压的方式, 形成周期振动, 并产生电流. 此时, 施加一个额外的反向电压使电流为零, 此时的反向电压数值上便等于此时的接触电势差. 这一方法对测量某一点的接触电势差具有较高的分辨率, 但无法对样品表面进行空间上的观测. 20世纪90年代以后, 扫描探针技术逐渐成熟. 1991年, 扫描探针显微镜与开尔文方法被结合到一起[22], 通过光束偏转法检测悬臂振动情况, 振动情况将被反馈至频率检测器, 从而以固有频率控制悬臂振动. 悬臂振动导致样品与探针间静电力的变化, 通过施加直流电压, 平衡此静电力变化, 从而获得样品与探针间的接触电势差. 一般情况下, 通过已知的稳定功函数的金属, 如金、铂等, 可以标定探针表面电势, 从而通过测得的接触电势差, 获得样品真正的电势分布情况.
半导体器件空间电势分布的研究对理解新型钙钛矿太阳能电池的工作机理起到至关重要的作用. 比如在开路条件下, 由空间电势分布可以获得器件内部电场和空间电荷区的强度和宽度信息, 并根据电势升高或降低判断能带弯曲方向; 在短路情况下, 则可以获取电荷载流子的产生和输运信息. 本文综述了利用开尔文探针力显微镜直接测量和分析钙钛矿太阳能电池内部的空间电势的研究进展, 讨论了其在加深和扩展器件工作机理认知中的应用, 并对其在未来研究中存在的问题和挑战做了进一步的展望, 希望对钙钛矿太阳能电池的研究起到一定的推动作用.
2
2.1.空间电势的光致变化研究
如图2(a)所示, 在基于正式介孔二氧化钛的器件中, 其空间电势通过开尔文探针力显微镜技术获得. 结果表明, 暗态条件下器件中的整体电势较为均匀; 加光照后, 钙钛矿光吸收层中的电势明显升高. 这些结果说明钙钛矿覆盖层中存在空穴积累, 而相应的电子被很好地传输至电子传输层中. 当条件由光照转至暗态后, 该研究发现介孔层中出现了被捕获的空穴, 钙钛矿层中出现被捕获的电子; 表明这些难以移动载流子是被缺陷中心捕获. 该研究认为这些被捕获的载流子可能是导致器件迟滞现象的原因. 另外, 过去研究认为正式介孔结构器件中的光吸收主要发生在介孔层. 然而, 空间电势光致变化结果表明, 介孔层部分的被捕获的空穴很少; 即使改变钙钛矿覆盖层的厚度, 空穴仍然集中在介孔层以上的钙钛矿覆盖层中(图2(b)). 因此, 该研究认为正式介孔结构钙钛矿太阳能电池的钙钛矿覆盖层是产生电荷载流子的重要区域[23]. 上述空间电势的直接观测研究给出了电荷载流子的产生、分离、传输的关键信息, 指出载流子的非平衡提取和陷阱积聚的空间位置, 为进一步提高器件性能的研究提供了重要的指导.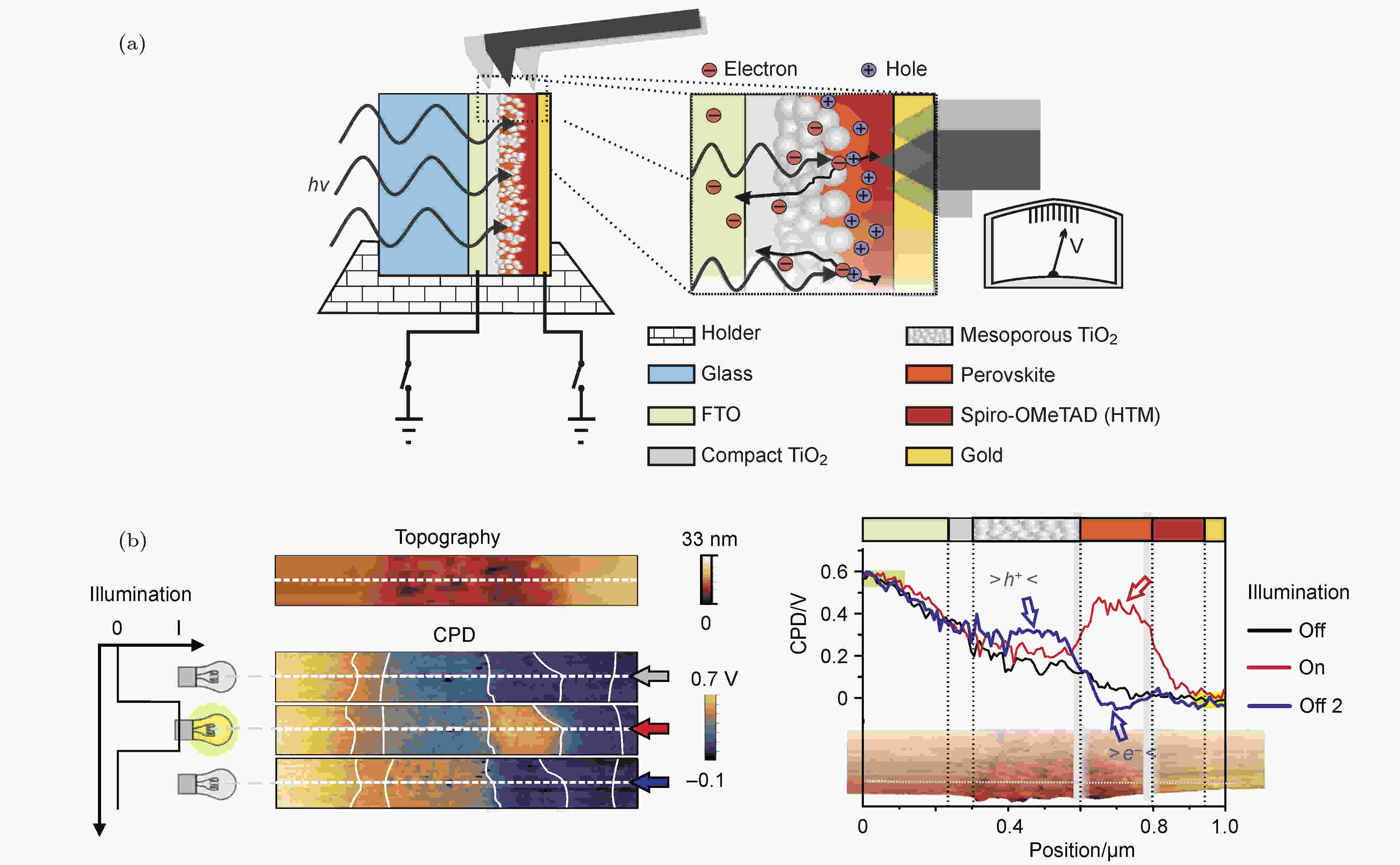
Figure2. (a) Potential of mesoporous perovskite solar cells using Kelvin probe force microscopy (FTO, fluorine-doped tin oxide; HTM, hole-transport material); (b) space potential changes of perovskite solar cells under illumination (CPD, contact potential difference)[23].
通过测量空间电势的变化, 还可以估算空间电荷区宽度, 从而对比不同电荷传输材料从钙钛矿中提取载流子的能力. 通过对比研究钙钛矿与二氧化钛界面以及钙钛矿与三氧化二铝的界面, 报道发现在暗环境下, 两者的空间电荷层几乎一致; 但在光照时, 钙钛矿与二氧化钛界面的空间电荷区达到45 nm, 而相应的钙钛矿与三氧化二铝的空间电荷区只有10 nm[24]. 该研究认为较宽的耗尽区有助于提高光生电荷载流子的界面分离效率.
在对比正式介孔结构和正式平面结构这两种钙钛矿电池的研究中, 空间电势的光致变化为深入的对比研究提供了直接观测结果. 通过开尔文探针力显微镜进行观测, 结果表明介孔结构器件中的空间电势变化主要发生在介孔二氧化钛和钙钛矿的界面处, 而且这一空间电势区的电势分布不受钙钛矿组分变化的影响, 在碘化铅过量亦或是碘甲胺过量的条件下基本保持不变. 该研究还发现, 正式介孔结构器件效率以及理想因子也几乎不受钙钛矿组分的影响(图3(a)和图3(b)). 然而在正式平面结构的电池中, 在钙钛矿层与电荷传输材料的上下界面处均存在空间电势的明显变化; 这一结果表明器件中存在更多电荷复合界面, 导致器件效率的降低. 基于此, 该工作通过改变钙钛矿组分来调节平面结构器件内空间电势的分布; 在碘化铅过量时, 电势突变主要发生在空穴传输层与钙钛矿层之间; 而碘甲胺过量时, 电势变化主要发生在电子传输层与钙钛矿层之间. 经过器件性能比较, 碘化铅过量的器件效率明显高于碘甲胺过量的器件, 且随碘化铅比例的增加而提高, 器件的理想因子也相应地逐渐降低. 这一结果说明器件中光生电荷载流子的复合逐渐降低, 相应的正式平面结构钙钛矿电池效率从16%提升至20%以上[25].
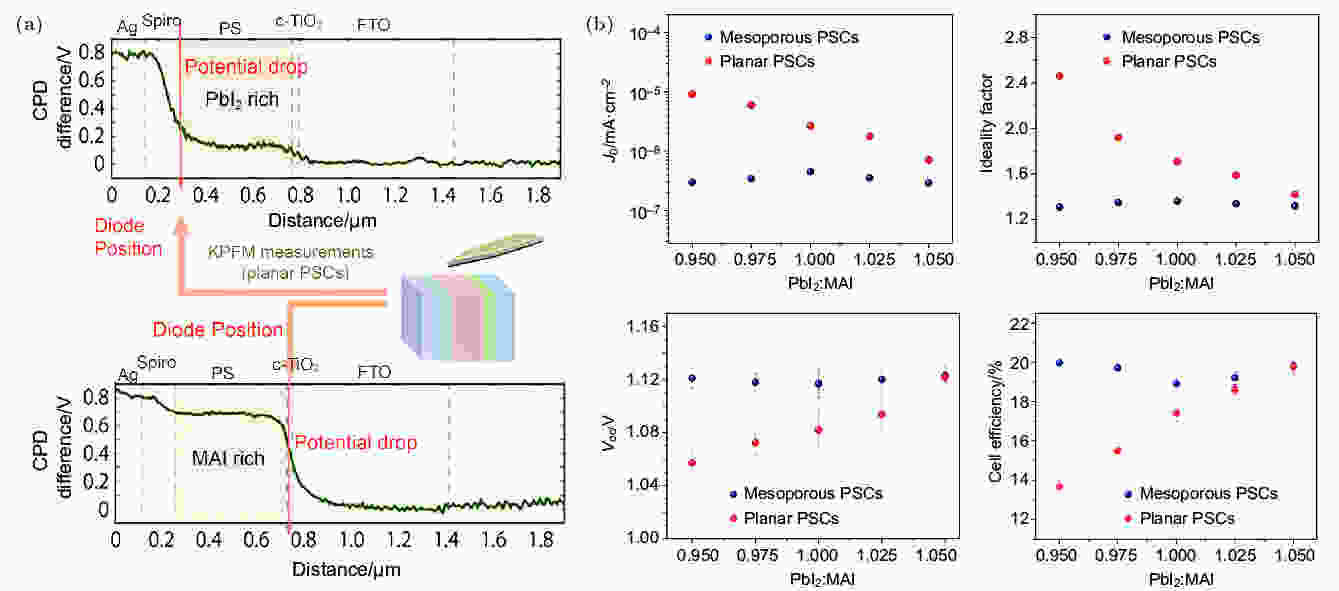
Figure3. (a) Kelvin probe force microscopy characterizations of perovskite solar cells for the mesoporous structures using MAI- and PbI2-Rich precursors; (b) photovoltaic performance of mesoporous and planar perovskite solar cells and ideality factor on PbI2/CH3NH3I(MAI) mole ratio[25].
电势的光致变化研究不仅可以用于测量器件截面的电势分布, 也可以在薄膜表面进行, 从而为器件的机理研究提供依据[26]. 通过表面电势的分布与强弱可以判断缺陷态密度[27,28]、能带弯曲情况[29]、相均匀性[30]、界面老化[31]等问题, 这里不再赘述.
2
2.2.空间电势的电致变化研究
与光照条件的影响不同, 改变器件的偏压条件可以研究器件在正向偏置或反向偏置下的空间电势变化, 从而获取更多的电荷载流子的分布和输运过程等电学特性信息. 在不加偏压的情况下, 以二氧化钛为电子传输层的正式平面结构器件的空间电势分布如图4(a)所示. 由于表面静电荷的存在, 其电势分布并不均匀, 因此, 不同偏压条件下电势变化是通过减去偏压为零时的结果来获得. 对空间电势进行一阶求导可以获得器件内部电场变化的空间信息. 在正式平面结构的钙钛矿太阳能电池中, 空间电势急剧变化均发生在二氧化钛电子传输层和钙钛矿界面处(图4(b)和图4(c)), 空间电荷区的耗尽宽度达到300 nm; 在正式介孔结构的器件中, 空间电势变化还发生在介孔层与钙钛矿覆盖层之间的界面处. 该研究认为在钙钛矿太阳能电池中, 影响载流子分离和传输的机制主要是p-n结结构和少数载流子的扩散与漂移过程, 并认为提高电池性能的方法是提高载流子的迁移率[22]. 上述研究结果与其他报道的光致变化结果有些差别; 内在原因包括材料组分的不同、光致变化与电致变化条件的不同. 另外, 器件整体光电转换效率的不同对于测试结果也会有一定影响.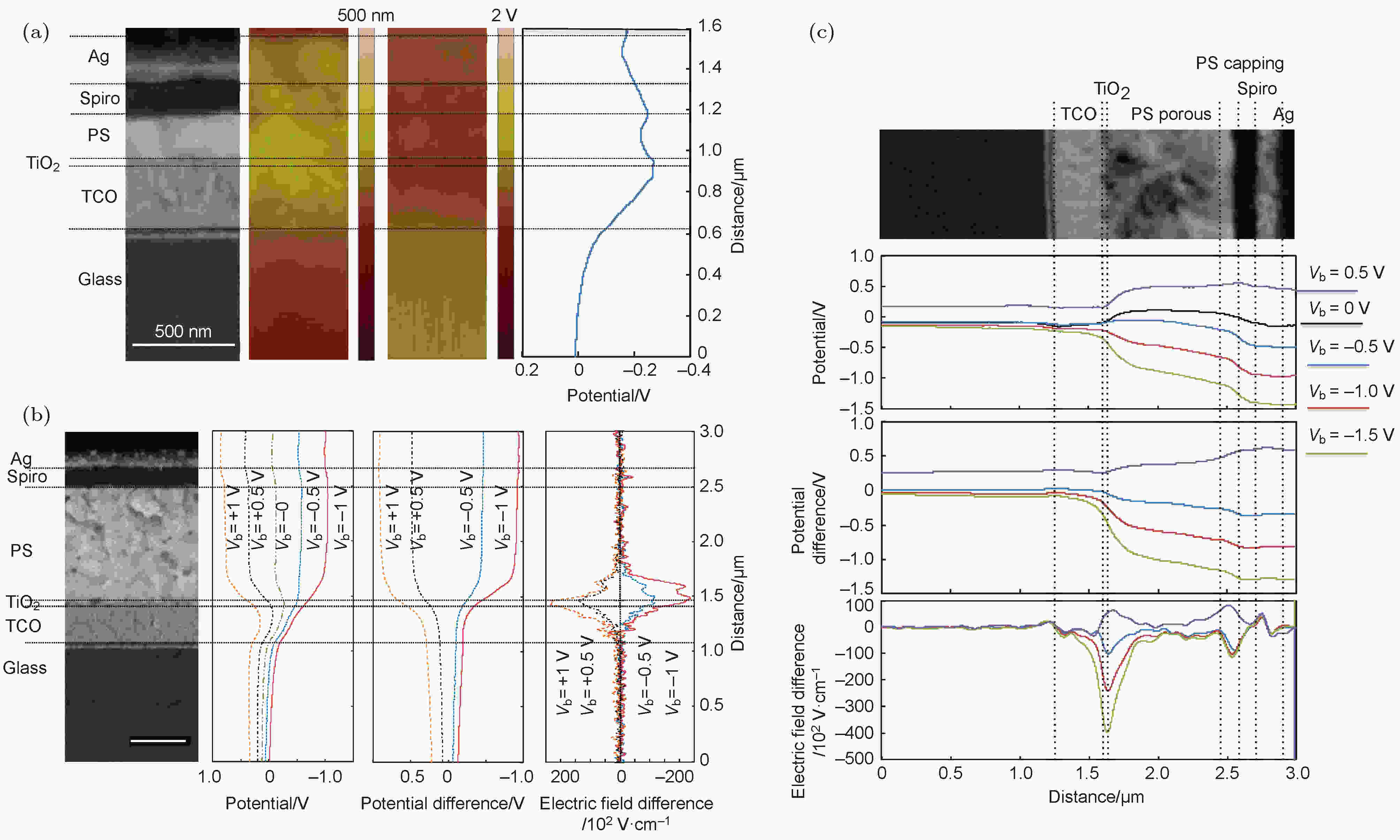
Figure4. (a) Potential distribution of mesoporous perovskite solar cells under Vb = 0 (TCO, transparent conducting oxide; PS, perovskite); (b) electrical potential and field profiling results on the planar device under different biases; (c) electrical potential and field profiling results on the optimized mesoporous device under different biases[19].
在研究以二氧化锡为电子传输层的正式平面器件时, 曾发现其空间电势的突变位置有两处, 分别在电子传输层/钙钛矿和钙钛矿/空穴传输层界面处(图5(a)). 两处电势突变的相对大小与电子传输层的电荷载流子迁移率相关, 较高的电子迁移率导致较高的电子传输, 可以减少诸如电流-电压曲线回滞等电荷传输不平衡现象. 当二氧化锡电子传输层的导电性差, 其与钙钛矿界面处的电荷传输效率也相应降低; 在加外电压的情况下, 漏电流过大会引起空间电势较大的变化. 从开尔文探针力显微镜的结果来看, 钙钛矿/空穴传输层界面处的电势突变越小, 表明器件漏电流小, 对应的电子传输层/钙钛矿界面的异质结质量更好. 因此, 通过对电子传输层进行简单退火后处理(图5(b)—(d)), 可以提高氧化锡基电子传输层的导电率、降低漏电流和界面载流子的非辐射复合, 器件内部电荷传输更加平衡, 而钙钛矿与空穴传输材料之间的电势降被基本消除, 相应器件性能提升至20%以上[32]. 通过对比以导电玻璃为基底的器件、以二氧化锡及二氧化锡/C60-SAM为基底的器件, 结果表明直接以导电玻璃为基底的器件在与钙钛矿接触的界面处呈现最小的电势降, 而以C60-SAM修饰过的二氧化锡器件呈现最大的电势降, 对应的器件效率也得到了大幅提升[33].
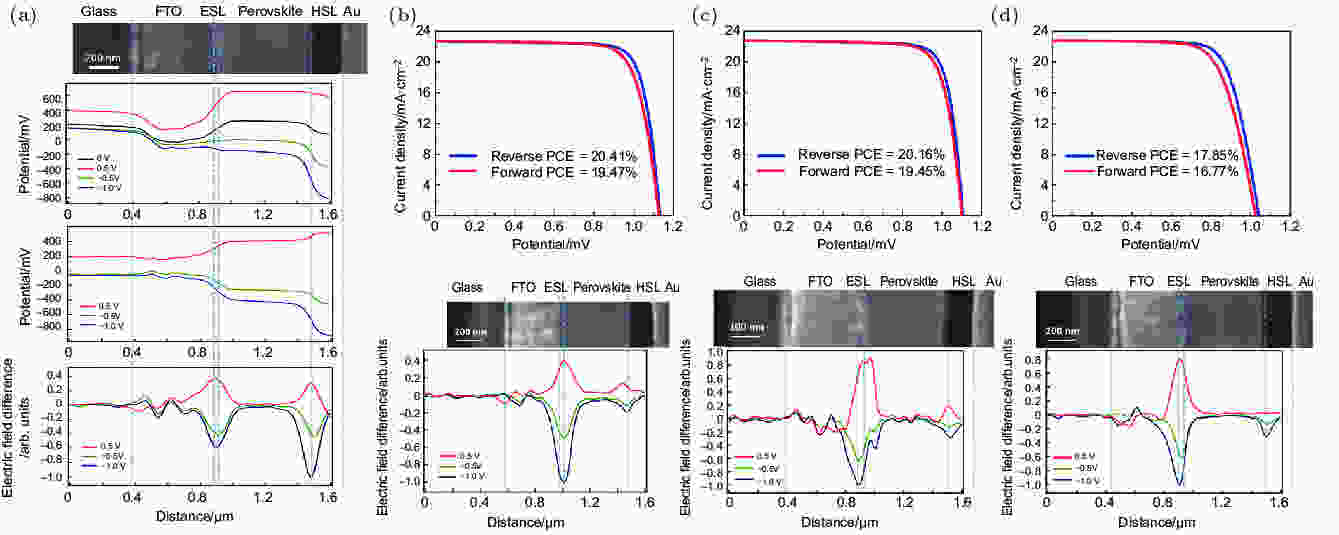
Figure5. (a) Potential difference of planar device based on SnO2 electron transfer layer, under different biases (fluorine-dopled SnO2, FTO; electron selective layer, ESL; hole selective layer, HSL); (b) 100, (c) 150, (d) 200 ℃ electrical potential and field profiling results of the device based on low-temperature thermal annealing of SnO2 electron transfer layer[32].
反式结构钙钛矿太阳能电池的空间电势分布与正式结构器件有所不同(图6(a)和图6(b)). 文献[34]报道, 反式结构器件的钙钛矿两端界面处都存在明显的电势变化, 表明两个界面的电荷载流子复合都较为严重, 这可能是目前反式器件电压较低的原因; 且电荷传输受到整个器件内空间电势的影响, 具有p-i-n结构的特征. 该研究表明在钙钛矿/金属氧化物界面处出现电荷注入势垒不利于电荷载流子的分离, 需要优化界面接触来进一步提高正式和反式平面结构钙钛矿太阳能电池的性能.
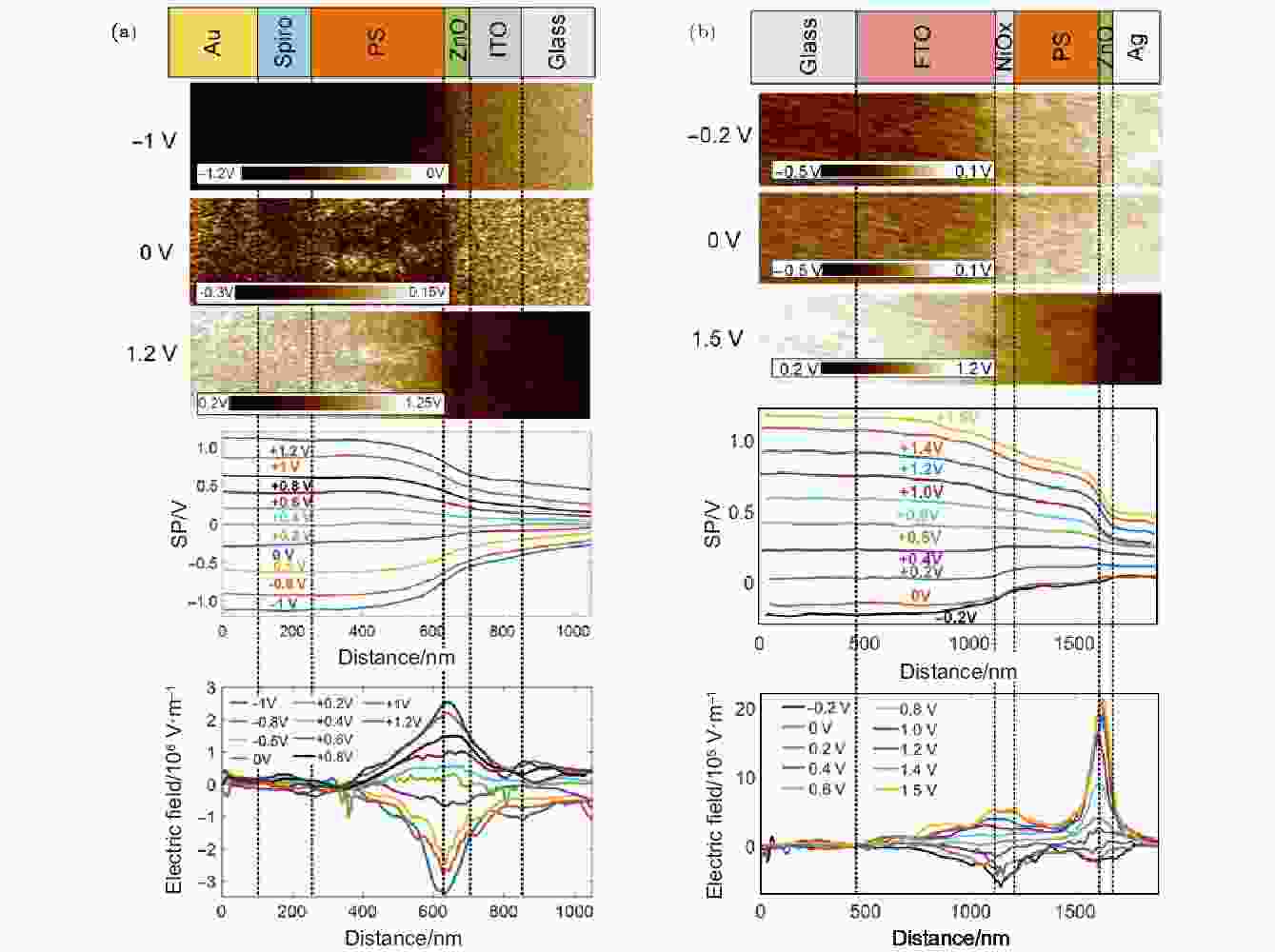
Figure6. (a) Potential distribution of regular perovskite solar cells under different biases; (b) potential distribution of inverted perovskite solar cells under different biases[34].
1)在快速发展的钙钛矿太阳能电池研究中, 通过直接观测器件内部空间电势来揭示器件光电转换机理的研究仍然偏少, 尤其是对于应用新型二维钙钛矿、纯无机钙钛矿、少铅或无铅钙钛矿以及新型无机或有机电子/空穴传输层等材料的电池器件, 相关机理研究严重滞后; 直接观测新型钙钛矿太阳能电池器件中空间电势的研究, 将为该领域的基础和应用研究提供重要科学依据.
2)钙钛矿太阳能电池的材料特性以及制备过程是影响光电转换过程的重要因素, 因此在较低效率的器件中, 影响空间电势分布的作用机制比较复杂; 而且, 在制备器件截面样品以进行空间电势探测的过程中, 器件被损坏的情况难以避免. 因此, 较高效率器件的空间电势分析结果的可靠性更高.
3) 开尔文探针力显微镜测试空间电势的过程中, 由于探针与样品间距离只有纳米级别, 表面粗糙样品会对探针产生损伤, 降低测试结果的准确性; 样品若较为柔软, 测试中容易在探针的作用下发生位移. 因此, 较低粗糙度的样品更适用于基于该类测量方法的研究.
4) 钙钛矿太阳能电池的组成材料稳定性不高, 如钙钛矿材料容易受水、氧的侵蚀而分解. 一般情况下, 为了提高开尔文探针力显微镜测试结果的信噪比, 需要较长时间积累数据信号, 因此在惰性环境中进行测试有利于获得更加可靠的结果.