1.
Introduction
Wurtzite zinc oxide (ZnO) possesses fantastic and unique properties like direct wide band gap (3.4 eV), low toxicity, thermodynamically stable phase and high exciton binding energy[1]. The unique properties of ZnO make it an attractive material for versatile applications such as transparent electronics, UV light emitting devices, piezoelectric devices, spin electronics, and chemical sensors[2–5]. To tune the physical properties of the wide band gap semiconductor materials, doping is effective and often leads to dramatic changes in these properties. Doping with transition metals and their oxides enhance electrical and optical properties, which increase UV and green emissions[6]. Vanadium is a transition metal having an electron configuration 3d34s2, which is expected to tune the structure and physical properties of ZnO. Much effort has been placed on the ferroelectric and ferromagnetic properties of vanadium doped ZnO nanoparticles, bulk materials and thin film[7–9]. Vanadium doping gives rise to distinctive absorption in the visible region and increases carrier lifetime[10]. A number of reports have shown that the vanadium doping in ZnO leads to a dramatic improvement in structural and optical properties of ZnO[11–16]. The luminescence properties of ZnO can be tuned by changing the vanadium doping contents in ZnO. The luminescence properties of V-doped ZnO thin films and nanostructures have been studied and investigated[10, 16]. However, the photo-luminescence of V doped ZnO has not received much attention in bulk form, which is favourable for technological applications. In this research article we focus our efforts on luminescence emission, and the vibrational and structural properties of the (ZnO)1?x(V2O5)x (x = 0, 3, 6 and 9 mol%) composite material. We elucidate and investigate more explicitly fine energy band gap tune-ability, average crystallite size, dislocation density and favorable luminescence mechanisms.
2.
Experimental details
ZnO (99.999%) and V2O5 (99.999%) powder were used to prepare (ZnO)1?x(V2O5)x (x = 0, 3, 6 and 9 mol%) composites. An appropriate ratio of ZnO (97, 94 and 91 mol%) and V2O5 (3, 6 and 9 mol%) were thoroughly mixed and ground with the help of a pestle and mortar for 2–3 h. These mixed powders were pressed to form cylindrical pellets (thickness = 2 mm and diameter 10 mm) and then sintered at 1000 °C for 6 h in air. X-ray diffraction analysis of the composites were carried out by using a Bruker D-8 X-ray diffractometer with Cu Kα radiation (λ = 1.541 86 ?) in the range of Bragg angles 2θ (20°–75°) with a scanning rate of 2°/min. Raman Spectroscopy is performed at room temperature in the region of 100–600 cm?1 with the help of a Dongwoo Optron MST-4000A Raman Spectrometer. Room temperature photo luminescence (PL) was performed with He–Cd laser source emitting at 325 nm (model MST-4000A). The signals were collected in scan mode through an air cooled (?60 °C) charged couple device (CCD) detector. The PL spectra was collected in the desired range from 350 to 650 nm using a triple grating DM320 monochromator and data was displayed by using ANDOR Solis CCD software.
3.
Results and discussion
3.1
XRD analysis
The crystal structure, phase purity and orientation of the ZnO and (ZnO)1?x(V2O5)x composites were analysed by using XRD as shown in Fig. 1. From the XRD pattern it is obvious that the samples with dopant concentration less than 9 mol% of V2O5 have a single phase and can be indexed to a hexagonal (wurtzite) ZnO (ICSD-06512). Peaks corresponding to the secondary phase of V2O5 and zinc oxide divanidium (ZnV2O6) are observed in composite with x = 9 mol%. To investigate the impact of dopant concentration on the lattice constants a and c the following expression for a hexagonal system[17, 18] was used.

class="figure_img" id="Figure1"/>
Download
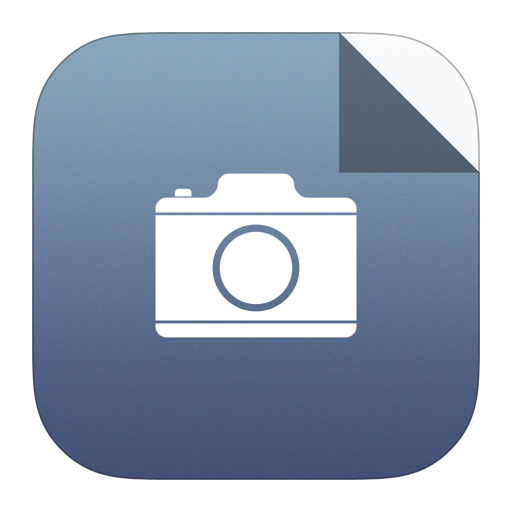
Larger image
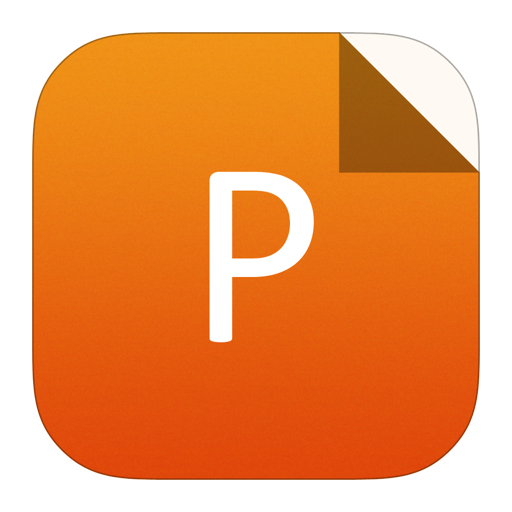
PowerPoint slide
Figure1.
(Color online) X-ray diffraction (XRD) patterns of (ZnO)1?x(V2O5)x composite.
$$frac{1}{{{d^2}}} = frac{4}{3}left( {frac{{{h^2} + hk + {k^2})}}{{{a^2}}}} ight) + frac{{{l^2}}}{{{c^2}}}.$$ ![]() | (1) |
The lattice parameters a and c for different samples calculated are shown in Table 1. The increase in a and c is due to the substitution of larger V+2 (0.93 ?) on the Zn2+ (0.74 ?) lattice site in tetrahedral coordination of ZnO wurtzite structure[19]. By using the Sherrer relation the average crystallite size D is calculated from XRD data[20].
$$D = frac{{0.9lambda }}{{{ m{FWHM}}cos theta }},$$ ![]() | (2) |
where λ is X-ray wavelength, FWHM is full width at half maxima and θ is Bragg’s angle in radian. It is found that the crystallite size increased with increase in doping contents up to 6 mol% and then decrease as shown in Table 1. The dislocation density of the preferential orientation was calculated by using the relation[21].
$$delta = frac{1}{{{D^2}}},$$ ![]() | (3) |
where D is the crystallite size in nm. From Table 1 it can be seen that the dislocation density decrease with increase in doping contents up to 6 mol% and then increases for 9 mol% of doping concentration. These results indicate that the V2O5 doping in ZnO improves the defective structure of ZnO by removing the intrinsic defects up to certain limits of doping contents. As the doping contents in ZnO increased (x = 9 mol%) extra phases related to impurities appear, which manifest that the solubility limit of V2O5 in ZnO is up to 6 mol%.
S. No | V2O5 composition (mol%) | Lattice constant c (nm) | Lattice constant a (nm) | Grain size (nm) | Dislocation density δ (10?3 nm?2) |
1 | 0 | 0.5219822 | 0.3262389 | 24.8756 | 1.6 |
2 | 3 | 0.5230075 | 0.3268797 | 26.15097 | 1.4 |
3 | 6 | 0.5249813 | 0.3281133 | 31.2996 | 1.021 |
4 | 9 | 0.523047 | 0.3269044 | 22.2252 | 2.024 |
Table1.
Lattice parameters for different samples.
Table options
-->
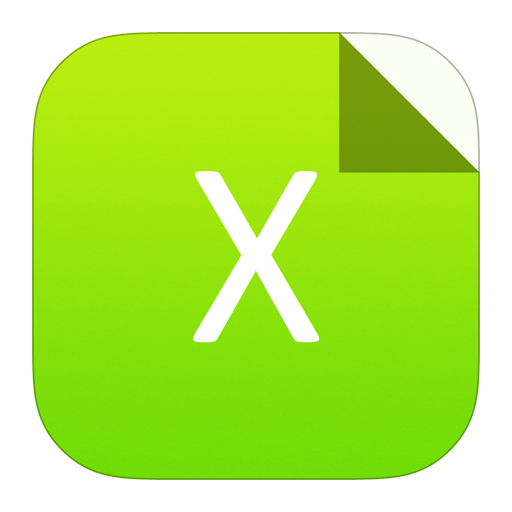
Download as CSV
S. No | V2O5 composition (mol%) | Lattice constant c (nm) | Lattice constant a (nm) | Grain size (nm) | Dislocation density δ (10?3 nm?2) |
1 | 0 | 0.5219822 | 0.3262389 | 24.8756 | 1.6 |
2 | 3 | 0.5230075 | 0.3268797 | 26.15097 | 1.4 |
3 | 6 | 0.5249813 | 0.3281133 | 31.2996 | 1.021 |
4 | 9 | 0.523047 | 0.3269044 | 22.2252 | 2.024 |
3.2
Raman spectroscopy
Raman spectroscopy is a non-destructive technique used to study the structural disorder and defects in the target materials. The ZnO wurtzite structure belongs to P63mc space group with six Raman active optical phonon modes at the centre of the Brillouin zone such as[22, 23],
$${varGamma _{{ m{opt}}}} = {A_1} + 2{B_1} + {E_1} + 2{E_2},$$ ![]() |
where A1 and E1 are polar modes and grouped into longitudinal optical (LO) and transverse optical (TO) modes, all are Raman and infrared active. E2 (low and high) modes are non-polar and Raman active. The effect of doping on the behavior of these modes in (ZnO)1?x(V2O5)x (x = 0, 3, 6, 9 mol%) was investigated by using high-resolution Laser Raman spectroscopy. Fig. 2 illustrates Raman Spectra of ZnO and (ZnO)1?x(V2O5)x composite with the peaks located at 436, 378 and 330 cm?1. These peaks are reported in the literature for wurtzite ZnO[24, 25]. It can be seen from Fig. 2 that the peak appearing at about 436 cm?1 is due to the vibration of Zn and O lattice in wurtzite ZnO structure and is designated as E2 (high)[26]. The effect of doping on the crystal quality of ZnO can be seen from the change in intensity of E2 (high) mode. The intensity of E2 (high) increased with V2O5 concentration up to 6 mol% and then decreased, indicating the improved ZnO crystal quality then deteriorate with further addition of V2O5 due to the precipitation of Vanadium metallic clusters. This is in agreement with our XRD results where the dislocation density decreased with Vanadium incorporation. A weak peak located at 380 cm?1 corresponds to E1 (TO) of ZnO[13]. The E1 (TO) become prominent and shift towards the lower wave number region (376 cm?1) after doping. The peak position at 330 cm?1 in ZnO spectra shift towards lower frequency in the (ZnO)1?x(V2O5)x composites, which corresponds to the second order transverse optical (TO) phonons mode ofA1 (TO)[27]. A small Raman mode at 580 cm?1 in pure ZnO can be observed, which disappears after doping of Vanadium contents. The phonon mode located at 580 cm?1 is the longitudinal optical (LO) phonon mode of ZnO, which has E1 symmetry and clearly reflects the existence of defects (the defects attributed due to oxygen vacancies and Zn interstitials) in ZnO materials[26]. The peaks observed in the range of 100–330 cm?1 in V doped ZnO composites correspond to the oxides of vanadium modes and arises due the lattice and bending modes of V–O. The A1 (TO) mode observed in Raman spectra of ZnO disappears in doped samples. It can be observed by careful analysis that the A1 (TO) mode is overlapped by some other mode appearing at 315 cm?1 in doped samples. The peak at 315 cm?1 is broad and consists of more than one peak, and is attributed to the triply coordinated oxygen (V3–O) bond and stretching band[28]. When doping contents in ZnO reach to 9 mol% two additional peaks positioned at 187 and 253 cm?1 appeared, which corresponds to the stretching mode of chain translation (V2O2)n and the V–O–V bending mode[29, 30].

class="figure_img" id="Figure2"/>
Download
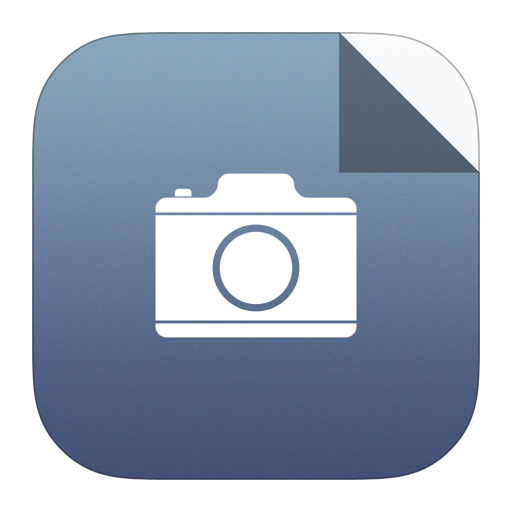
Larger image
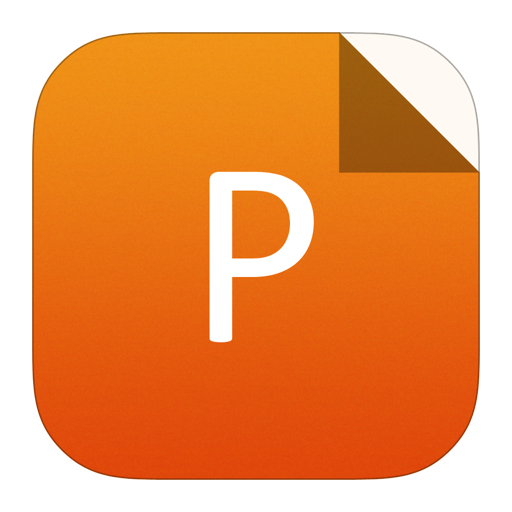
PowerPoint slide
Figure2.
(Color online) Raman spectra for (ZnO)1?x(V2O5)x composite.
3.3
Photo luminescence spectroscopy
To examine the optical luminescent behaviour of ZnO and (ZnO)1?x(V2O5)x composites, room temperature photo luminescence spectra was recorded under 325 nm excitation wavelength and the results are shown in Fig. 3. From Fig. 3 one can see that the PL spectrum consists of near band edge (NBE) and broad deep level emission. The NBE peak position changes with the increase in doping contents and shifts towards the lower wavelength. The NBE peak of ZnO centered at ~383 nm, while for doped samples it shifts to 374 nm. Such a blue shift is due to the increase in band gap energy as observed previously in Mg:ZnO films[31]. The blue shift in NBE arises due to the contribution of electrons in the conduction band by the doping element and the Fermi level shifts up towards the conduction band[32]. Another interesting feature observed from Fig. 3 is the enhancement in intensity of the NBE with the increase in doping contents of V2O5 (x = 6 mol%) in ZnO and then decrease with further increase in V2O5 concentration. As observed in our XRD results that the dislocation density decrease with increase in V2O5 concentration, so we can infer on the base of our XRD findings that the incorporation of vanadium in ZnO lattice results in the reduction of Zn vacancies and hence an improved NBE emission arise. The intensity of the NBE remarkably decreased i.e. quenched for x = 9 mol%, which indicates a non radiative recombination process. The non radiative recombination process depends on doping contents and lattice defects in wide band gap semiconductor materials[33]. When V2O5 concentration is high enough (x > 6 mol%), the excess vanadium concentration appears as a vanadium cluster, and the defects due to the doping element in ZnO structure. These defects decrease the radiative recombination efficiency in ZnO and quench the NBE emission.

class="figure_img" id="Figure3"/>
Download
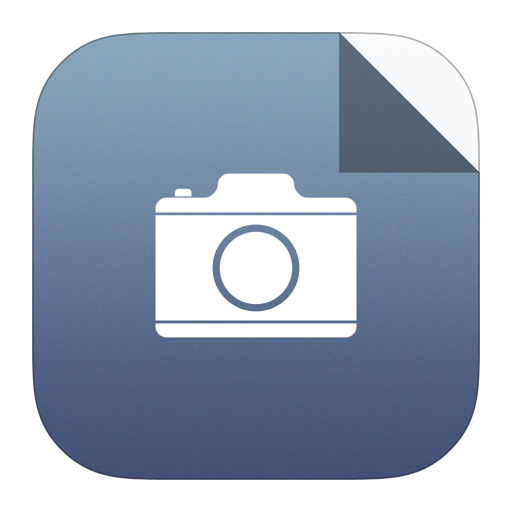
Larger image
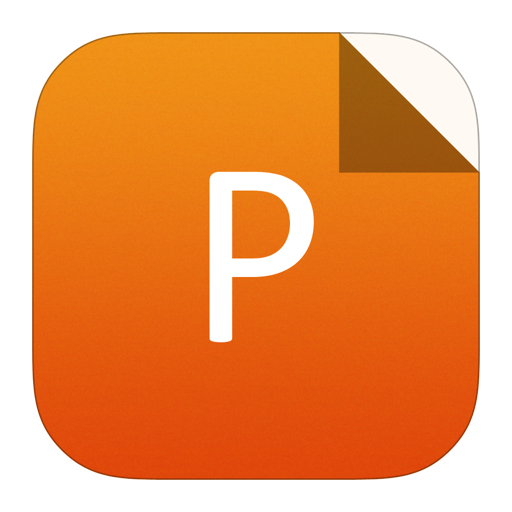
PowerPoint slide
Figure3.
(Color online) Room temperature PL spectra of (ZnO)1?x(V2O5)x composite.
On the other side, broad deep level emission can be seen from the PL spectrum shown in Fig. 3. The intensity of broad deep level emission decreases with increase in doping contents and approximately vanishes, when V2O5 concentration is 6 mol%. However, the deep level emission emerges again, when V2O5 concentration in ZnO is 9 mol%. The deep level emission arises due to extrinsic and intrinsic defects as reported in the literature[33, 34]. In our study the intrinsic defects such as VZn and VO may be responsible for deep level emission[35]. When the V2O5 concentration in ZnO is equal to or less than 6 mol%, the incorporation of doping contents suppress the intrinsic defects, which results in the decreased intensity of broad deep level emission. As the doping contents are further increased (x > 6 mol%), the defects due to doping element increase, as confirmed from the calculated value of dislocation density in XRD and additional modes observed in Raman spectra causing an increase in the intensity of the broad deep level emission.
4.
Conclusion
In summary, high quality (ZnO)1?x(V2O5)x (x = 0, 3, 6 and 9 mol%) composites with hexagonal structure were prepared using the solid state reaction technique. Different characterization techniques such as XRD, Raman and photo-luminescence spectroscopy were carried out to investigate structural, vibrational and photo-luminescence characteristics of the composites. XRD results indicate the hexagonal wurtzite structure of (ZnO)1?x(V2O5)x composite with proper substitution of V ions at Zn lattice sites. By using XRD data lattice constants, crystallite size and dislocation density were calculated, which indicate that the crystalline quality gets improved with vanadium doping (x = 6 mo%), and further increase in doping concentration results in the deterioration in the crystalline quality. Raman spectroscopy indicate the existence of the fundamental Raman modes of wurtzite ZnO. Room temperature photo-luminescence of the (ZnO)1?x(V2O5)x composite consist of two emission bands such as NBE and broad deep level emission. The intensity of NBE enhanced and the broad deep level emission reduced for x 6 mol%, which indicate the suppression of defects with doping. However, further increase in doping contents ( x > 6 mol%) results in defects which suppress the NBE and increase the broad deep level emission. A blue shift in the NBE emission peak appears with doping, which indicates that the optical band gap energy increases with doping.
Acknowledgments
The authors gratefully acknowledge the assistance from the National Institute of Laser and Optronics (NILOP) for providing research capacities.