1.
Introduction
In recent years, the clusters of different materials have attracted a great interest and their study has become a hot topic of research in both physics and chemistry. Because of their very small size, the evolution of their physical and chemical properties became very important for many applications in different fields such as optoelectronic materials, catalyst, nanoelectronic and spintronic[1, 2]. Semiconductor clusters formed by the elements of group 14 have been also extensively investigated experimentally and theoretically in view of their fundamental understanding and technological applications. Germanium and silicon are the most important semiconductor elements with widespread applications. Previous studies have shown that the structure of small germanium and silicon clusters are very similar, while the larger ones are fundamentally different[3]. In this work, we studied germanium clusters. During the last four decades, pure and doped germanium clusters have been intensively studied experimentally[4–10] and theoretically[11–17]. Zhao and Wang[18] studied geometries, stabilities and electronics properties of FeGen (n = 9–16) clusters. They found that the encapsulation of one iron atom Fe in the pure Gen clusters contributes in strengthening the stabilities of the germanium framework and the HOMO-LUMO gaps of the FeGen clusters which are usually smaller than those of the corresponding pure germanium clusters. In their study concerned with the properties of aluminum-doped small germanium clusters, Shi et al.[19] showed that the lowest energy structures of the AlGen are similar to lowest energy structures of the pure corresponding germanium clusters. Li et al.[20] reported the effect of one atom of gold Au in anionic germanium clusters AuGen– (n = 1–13). They found that the clusters with n = 12 are the most stable ones compared to the other size. Electronic and magnetic properties of Mn-, Co- and Ni-doped small germanium clusters have been investigated using spin polarized density functional theory by Kapila et al.[21]. They found that the Ni-doped Gen clusters are the more stable when compared to Co- and Mn-doped Gen clusters. Tang et al.[22] studied the structure and stability of the 3d transition metal endohedral Ge12M (M = Sc–Ni) clusters. They found that all Ge12M clusters have the doping energies (DEs) and the energies gap (Egs) comparable to the isolated compounds, which indicates their remarkably kinetic stability and the possibility for isolation. Mahtout et al.[2] calculated the electronic and magnetic properties of medium-sized CrGen (15 ≤ n ≤ 29) clusters by using first principles DFT investigation. They showed that the structures of Gen+1 and CrGen become more compact and switch to near-spherical structure with one or more core atoms and the clusters with size 17, 19, and 22 exhibit high stability. Katircioglu[23] reported that the C–C bonds are privileged over Ge–C bonds and Ge–Ge binding for different values of n and m in GenCm–n clusters. Recently, small SnGen clusters have been studied in the range n < 5. Andzelm et al.[24] performed a DFT study on GeSn monomer, and they reported on the spectroscopic constants and electronic structure of the diatomic molecules GeSn in their low-lying electronic states. They showed that the local spin-density model potentials (LSD) used in their study form a good description of chemical bonding and spectroscopic properties of molecules. Schmude and Gingenich[25] presented an experimental study on the small germanium–tin clusters and they find the atomization enthalpies of the GeSn, GeSn2, Ge2Sn, Ge3Sn, Ge4Sn molecules. A DFT investigation of SnmGen (m + n ≤ 5) binary clusters has been performed by Samanta and Das[1]. They showed that the larger mixed tin and germanium clusters have higher binding energies and the larger HOMO–LUMO energy gaps, which implies their high stability. Hanet al.[26] presented theoretical investigation of very small SnGen (n = 1–4) clusters. They calculated equilibrium geometry, enthalpy of reaction and natural population and they found that natural populations of these clusters indicate a charge transfer from Sn to Ge atoms. In light of the previous studies, to the best of our knowledge, systematic and theoretical investigation on neutral and charged tin-doped germanium SnGen clusters with range of 5 to 17 atoms have not been reported so far. The main motivation behind the present work is to study the inclusion effect of one Sn on the electronic and magnetic properties of different isomers of Gen+1 clusters in the size range n = 1–17 atoms and their evolution as a function of the size by using the density functional theory calculations. The properties and the associated adiabatic energies of charged tin-doped germanium are also studied. Our investigation will provide noteworthy contribution for theoretical and experimental studies. The paper is organized as follows: we describe the computational details in Section 2, Section 3 is devoted to discuss the obtained results and in Section 4 we draw some conclusions.
2.
Method
In this study, ab initio molecular orbital and density functional theory (DFT) calculations are performed by using the SIESTA software package[27–29] (the Spanish Initiative for Electronic Simulation with Thousands of Atoms). The density functional is treated by generalized gradient approximation (GGA) with exchange correlation potential developed by Perdew and Zanger[30] and Perdew, Burke and Ernserhof (PBE)[31]. Self-consistent field procedures are carried out with a convergence (SZ) basis set for Sn and Ge atoms. The ionic core criterion of 10–4 a.u. on the total energy and electron density. A big cubic supercell with dimension of 40 ? was used to create sufficient vacuum space to eliminate the image interactions. The k = 0 (Γ) point approximation was used in Brillouin zone sampling. During simulation, volume of the system was kept constant and a single ? potential were Bylander form[32]. The energy cut-off of 150 Ry was represented by norm-conserving Troullier-Martins[33] and non local pseudopotentiels factorized in the Kleinman and PAO. EnergyShift is taken equal to 50 meV. Conjugate gradient method within Hellmann Feynman forces was used and all the forces are less than 10–2 eV/?. In order to find the global minimum structures of SnGen clusters, at first we have optimized several isomers of pure germanium clusters with size of 2–18 atoms. Second, a great number of isomers for doped SnGen were considered. We have initially relaxed different possible isomers of neutral SnGen clusters. Then, the best obtained structures have been considered in their anionic and cationic configurations and relaxed. After conducting a simulation process, a comparative study between the properties of neutral and charged clusters was performed. In the discussion, we consider only the lowest energy isomers determined in our optimizations. In order to test the method used in geometry optimization, with respect to the exchange-correlation functional to the size and to the cutoff radii of basis sets, we performed calculations on Ge2 and Ge3 clusters. Our results shown in Table 1 are in good agreement with theoretical and experimental results of the literature.
Cluster | Our works | Theoretical values | Experimental values | |||||||||||
a | Eb | AEA | AIP | a | Eb | AEA | AIP | a | Eb | AEA | AIP | |||
Ge2 | 2.625 | 1.28 | 2.060 | 7.753 | 2.548d | 1.3e | 2.10i | 7.9j | / | 1.32g | 2.035g | 7.58–7.76j | ||
2.54b | 1.31f | 1.41m | 2.074h | |||||||||||
2.413c | 1.32j | |||||||||||||
2.41a | 1.230l | |||||||||||||
2.61k | 1.812k | |||||||||||||
Ge3 | 2.476 | 1.86 | 1.907 | 8.126 | 2.428i | 1.92e | 2.09i | 7.92j | / | 2.24n | 2.23g | 7.97–8.09j | ||
2.400l | 1.98d | 2.15m | 2.23h | |||||||||||
2.378d | 2a | |||||||||||||
2.296i | 2.04c | |||||||||||||
a Ref. [34]. b Ref. [35]. c Ref. [19]. d Ref. [36]. e Ref. [37]. f Ref. [38]. g Ref. [4]. h Ref.[5]. I Ref.[11]. j Ref.[39]. k Ref. [2]. l Ref. [12]. m Ref. [40]. n Ref. [41]. |
Table1.
Average bond length a (?), binding energy Eb (eV), adiabatic electronic affinity (AEA) (eV) and adiabatic ionisation potential (AIP) (eV) for Ge2 and Ge3 clusters.
Table options
-->
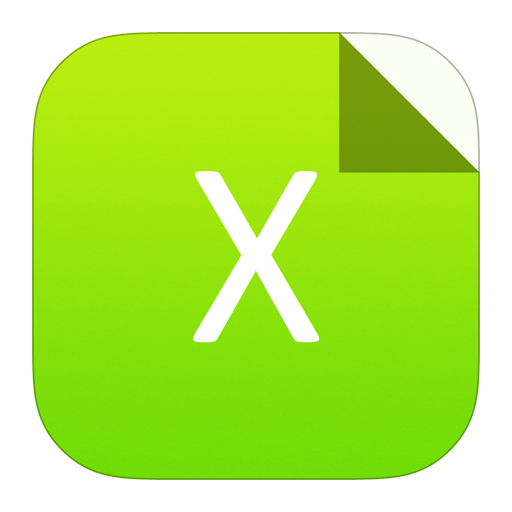
Download as CSV
Cluster | Our works | Theoretical values | Experimental values | |||||||||||
a | Eb | AEA | AIP | a | Eb | AEA | AIP | a | Eb | AEA | AIP | |||
Ge2 | 2.625 | 1.28 | 2.060 | 7.753 | 2.548d | 1.3e | 2.10i | 7.9j | / | 1.32g | 2.035g | 7.58–7.76j | ||
2.54b | 1.31f | 1.41m | 2.074h | |||||||||||
2.413c | 1.32j | |||||||||||||
2.41a | 1.230l | |||||||||||||
2.61k | 1.812k | |||||||||||||
Ge3 | 2.476 | 1.86 | 1.907 | 8.126 | 2.428i | 1.92e | 2.09i | 7.92j | / | 2.24n | 2.23g | 7.97–8.09j | ||
2.400l | 1.98d | 2.15m | 2.23h | |||||||||||
2.378d | 2a | |||||||||||||
2.296i | 2.04c | |||||||||||||
a Ref. [34]. b Ref. [35]. c Ref. [19]. d Ref. [36]. e Ref. [37]. f Ref. [38]. g Ref. [4]. h Ref.[5]. I Ref.[11]. j Ref.[39]. k Ref. [2]. l Ref. [12]. m Ref. [40]. n Ref. [41]. |
3.
Results and discussions
3.1
Structural properties
In cluster physics, one of the most important things in studying the properties of clusters is to determine their ground states geometries. The most obtained stable structure and their corresponding isomers for pure and tin-doped germanium clusters are shown in Figs. 1 and 2, respectively. In these figures, (a) isomer is the most stable structure. The energetic ordering of isomers for Gen+1 and SnGen (n = 1–17) clusters are given in Tables 2 and 3, respectively. In these tables, the parameters of the most stable structures for each size are reported in bold character. In Table 4, we give the average Ge–Ge and Sn–Ge bond lengths of the lowest energy structures of SnGen(0, ±1) clusters. Fig. 3 presents the most stable structures of anionic and cationic SnGen(±1) clusters and their parameters are described in Table 5. In the case of clusters with two atoms, we obtained a SnGe monomer with bond length of 2.805 ?, which is in good agreement with the theoretical value[33] of 2.753 ?. The binding energy equals 1.19 eV/atom, which is smaller than that of Ge2 monomer (1.28 eV/atom). For charged monomer, the bond length is 2.910 and 2.495 ? for SnGe+ and SnGe–, respectively, while the binding energy is 1.285 and 1.819 eV/atom, respectively. The cationic monomer is more stable than the neutral and anionic monomers. The triangular structure is the lowest energy structure for Ge3 with symmetry C2v and average bond length of Ge–Ge of 2.476 ?. For SnGe2 cluster, which has a triangular structure, with Cs symmetry, the average bond lengths Ge–Ge and Sn–Ge are 2.472 and 2.658 ?, respectively. The geometric structure of the anion cluster of SnGe2 is also triangular, whereas its cation cluster is angular with Cs symmetry. For Ge4, the most stable structure is the rhombus (D2h) with the bond length of 2.714 ? and the binding energy Eb = 2.25 eV/atom. The same structure is obtained by Wang et al.[12] in their theoretical study and Li et al.[39] in their experimental study on the Gen systems. In the case of SnGe3 cluster, the ground state structure showed a C2v symmetry with Sn–Ge and Ge–Ge bond distance of 2.857 and 2.741 ?, respectively. Rhombus structure is also found to be the most stable structure for charged SnGe3 cluster. The ground state of Ge5 cluster is a trigonal bipyramid with D3h symmetry and average bond length of 2.668 ?. Our calculations indicate that the SnGe4 cluster has also trigonal bipyramid with C2v structure. Their Ge–Ge bond length increases to 2.665 ? and Sn–Ge bond length is found to be 2.852 ?.

class="figure_img" id="Figure3"/>
Download
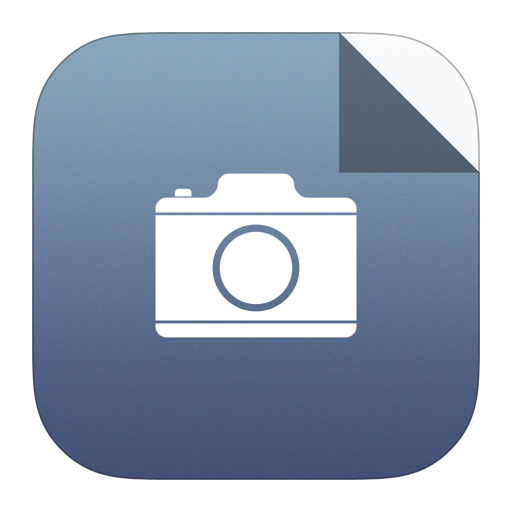
Larger image
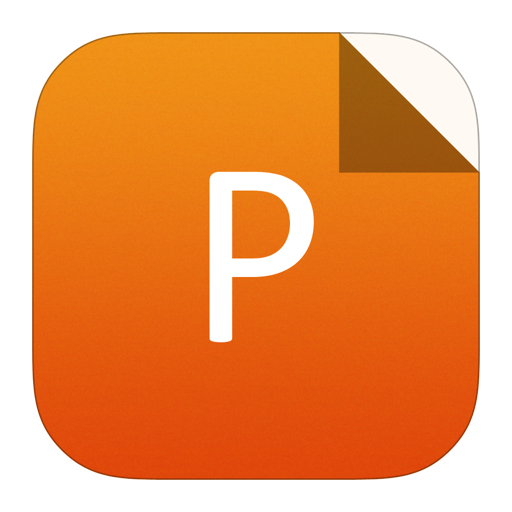
PowerPoint slide
Figure3.
(Color online) Ground state structures of cationic and anionic SnGen(±1) (n = 1–17) clusters.
Size (n+1) | Symmetry group | Eb (eV/atm) | ΔE (eV) | a (?) |
1 | (a) D∞h | 1.28 | 0.367 | 2.625 |
2 | (a) C2v | 1.86 | 1.368 | 2.476 |
3 | (a) D2h | 2.25 | 1.152 | 2.714 |
4 | (a) D3h | 2.44 | 1.815 | 2.668 |
5 | (a) C2v | 2.55 | 1.650 | 2.823 |
(b) Cs | 2.49 | 1.321 | 2.811 | |
6 | (a) D5h | 2.66 | 1.557 | 2.900 |
(b) C2v | 2.59 | 2.111 | 2.722 | |
7 | (a) Cs | 2.64 | 1.179 | 2.796 |
(b) C2 | 2.61 | 1.143 | 2.837 | |
(c) C2h | 2.61 | 1.153 | 2.836 | |
8 | (a) C2v | 2.70 | 1.588 | 3.000 |
(b) C1 | 2.64 | 1.306 | 2.854 | |
9 | (a) C3v | 2.80 | 1.609 | 2.857 |
(b) Cs | 2.73 | 1.415 | 2.854 | |
(c) C2v | 2.60 | 0.817 | 2.849 | |
10 | (a) Cs | 2.76 | 1.148 | 2.857 |
(b) C1 | 2.76 | 1.140 | 2.857 | |
(c) C2 | 2.73 | 1.143 | 2.944 | |
11 | (a) C2v | 2.76 | 1.536 | 2.884 |
(b) C1 | 2.75 | 1.113 | 2.940 | |
(c) Cs | 2.71 | 1.299 | 2.855 | |
12 | (a) C1 | 2.77 | 0.982 | 2.943 |
(b) C2 | 2.75 | 1.235 | 2.836 | |
(c) Cs | 2.75 | 1.096 | 2.788 | |
13 | (a) Cs | 2.83 | 1.372 | 2.867 |
(b) C1 | 2.80 | 1.335 | 2.888 | |
(c) C2 | 2.76 | 1.014 | 2.871 | |
14 | (a) C2v | 2.82 | 0.864 | 2.925 |
(b) C1 | 2.78 | 1.116 | 2.768 | |
(c) Cs | 2.77 | 1.163 | 2.862 | |
15 | (a) C2h | 2.83 | 1.164 | 2.893 |
(b) C1 | 2.78 | 0.978 | 2.852 | |
(c) C1 | 2.77 | 1.172 | 2.825 | |
(d) C1 | 2.77 | 1.138 | 2.875 | |
16 | (a) Cs | 2.82 | 0.884 | 2.893 |
(b) C1 | 2.82 | 0.908 | 2.861 | |
(c) C1 | 2.82 | 1.116 | 2.836 | |
17 | (a) C1 | 2.81 | 1.322 | 2.822 |
(b) C1 | 2.79 | 0.626 | 2.845 |
Table2.
Symmetry group, binding energy per atom Eb, HOMO-LUMO gap ΔE and average bond length for pure Gen+1 (n = 1–17) clusters.
Table options
-->
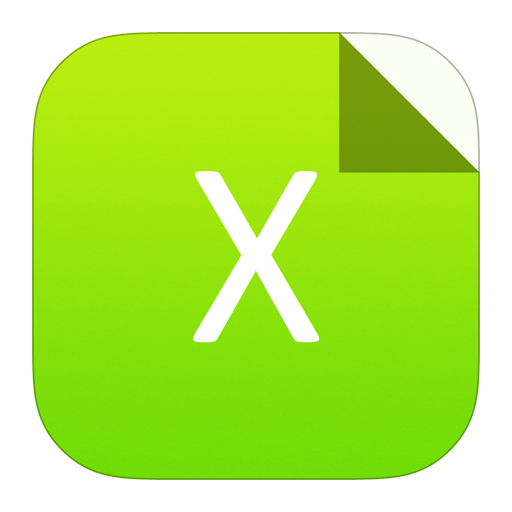
Download as CSV
Size (n+1) | Symmetry group | Eb (eV/atm) | ΔE (eV) | a (?) |
1 | (a) D∞h | 1.28 | 0.367 | 2.625 |
2 | (a) C2v | 1.86 | 1.368 | 2.476 |
3 | (a) D2h | 2.25 | 1.152 | 2.714 |
4 | (a) D3h | 2.44 | 1.815 | 2.668 |
5 | (a) C2v | 2.55 | 1.650 | 2.823 |
(b) Cs | 2.49 | 1.321 | 2.811 | |
6 | (a) D5h | 2.66 | 1.557 | 2.900 |
(b) C2v | 2.59 | 2.111 | 2.722 | |
7 | (a) Cs | 2.64 | 1.179 | 2.796 |
(b) C2 | 2.61 | 1.143 | 2.837 | |
(c) C2h | 2.61 | 1.153 | 2.836 | |
8 | (a) C2v | 2.70 | 1.588 | 3.000 |
(b) C1 | 2.64 | 1.306 | 2.854 | |
9 | (a) C3v | 2.80 | 1.609 | 2.857 |
(b) Cs | 2.73 | 1.415 | 2.854 | |
(c) C2v | 2.60 | 0.817 | 2.849 | |
10 | (a) Cs | 2.76 | 1.148 | 2.857 |
(b) C1 | 2.76 | 1.140 | 2.857 | |
(c) C2 | 2.73 | 1.143 | 2.944 | |
11 | (a) C2v | 2.76 | 1.536 | 2.884 |
(b) C1 | 2.75 | 1.113 | 2.940 | |
(c) Cs | 2.71 | 1.299 | 2.855 | |
12 | (a) C1 | 2.77 | 0.982 | 2.943 |
(b) C2 | 2.75 | 1.235 | 2.836 | |
(c) Cs | 2.75 | 1.096 | 2.788 | |
13 | (a) Cs | 2.83 | 1.372 | 2.867 |
(b) C1 | 2.80 | 1.335 | 2.888 | |
(c) C2 | 2.76 | 1.014 | 2.871 | |
14 | (a) C2v | 2.82 | 0.864 | 2.925 |
(b) C1 | 2.78 | 1.116 | 2.768 | |
(c) Cs | 2.77 | 1.163 | 2.862 | |
15 | (a) C2h | 2.83 | 1.164 | 2.893 |
(b) C1 | 2.78 | 0.978 | 2.852 | |
(c) C1 | 2.77 | 1.172 | 2.825 | |
(d) C1 | 2.77 | 1.138 | 2.875 | |
16 | (a) Cs | 2.82 | 0.884 | 2.893 |
(b) C1 | 2.82 | 0.908 | 2.861 | |
(c) C1 | 2.82 | 1.116 | 2.836 | |
17 | (a) C1 | 2.81 | 1.322 | 2.822 |
(b) C1 | 2.79 | 0.626 | 2.845 |
Size (n) | Symmetry group | Eb (eV/atom) | ΔE (eV) | VEA (eV) | VIP (eV) | η (eV) | μ (μb) |
1 | (a) C∞v | 1.19 | 0.344 | 1.199 | 7.383 | 6.184 | 2.000 |
2 | (a) Cs | 1.81 | 1.311 | 1.441 | 7.982 | 6.541 | 0.000 |
(b) C∞v | 1.71 | 1.643 | 1.264 | 8.719 | 7.455 | 0.000 | |
(c) C2v | 1.80 | 0.984 | 1.702 | 7.936 | 6.234 | 0.000 | |
3 | (a) C2v | 2.20 | 0.227 | 1.715 | 8.039 | 6.324 | 0.000 |
(b) C2v | 1.79 | 1.132 | 2.386 | 7.072 | 4.686 | 0.000 | |
4 | (a) C2v | 2.40 | 1.739 | 1.649 | 8.912 | 7.263 | 0.000 |
(b) C3v | 2.35 | 1.556 | 1.255 | 8.447 | 7.192 | 0.000 | |
(c) Cs | 2.19 | 0.499 | 2.687 | 7.367 | 4.680 | 2.000 | |
(d) C2v | 2.14 | 0.889 | 2.959 | 6.840 | 3.881 | 2.000 | |
5 | (a) Cs | 2.53 | 1.663 | 1.845 | 8.022 | 6.177 | 0.000 |
(b) Cs | 2.48 | 1.450 | 2.056 | 7.798 | 8.742 | 0.000 | |
(c) Cs | 2.44 | 1.228 | 1.873 | 7.786 | 5.913 | 0.000 | |
6 | (a) C1 | 2.63 | 1.853 | 2.064 | 8.241 | 6.177 | 0.000 |
(b) C1 | 2.61 | 1.512 | 2.357 | 8.153 | 5.796 | 0.000 | |
(c) C2v | 2.53 | 1.451 | 1.590 | 7.856 | 6.266 | 0.000 | |
7 | (a) C1 | 2.62 | 1.197 | 2.059 | 7.538 | 5.479 | 0.000 |
(b) C1 | 2.59 | 1.149 | 2.076 | 7.416 | 5.340 | 0.000 | |
(c) Cs | 2.58 | 0.947 | 2.047 | 7.179 | 5.132 | 0.000 | |
8 | (a) C1 | 2.68 | 1.486 | 1.749 | 7.745 | 5.996 | 0.000 |
(b) C1 | 2.68 | 1.515 | 1.978 | 7.425 | 5.447 | 0.000 | |
9 | (a) Cs | 2.77 | 1.551 | 2.306 | 7.714 | 5.408 | 0.000 |
(b) C1 | 2.69 | 1.434 | 2.197 | 7.497 | 5.300 | 0.000 | |
10 | (a) Cs | 2.73 | 1.202 | 2.291 | 7.204 | 4.913 | 0.000 |
(b) C1 | 2.72 | 1.031 | 2.378 | 7.133 | 4.755 | 0.000 | |
(c) C1 | 2.71 | 1.121 | 2.511 | 7.324 | 4.813 | 0.000 | |
11 | (a) C1 | 2.74 | 1.529 | 2.225 | 7.321 | 5.096 | 0.000 |
(b) C5v | 2.70 | 1.155 | 2.583 | 7.388 | 4.805 | 0.000 | |
(c) Cs | 2.67 | 1.104 | 2.667 | 7.359 | 4.692 | 0.000 | |
(d) C1 | 2.64 | 1.027 | 3.628 | 6.242 | 2.614 | 0.000 | |
12 | (a) C1 | 2.73 | 0.992 | 2.230 | 7.207 | 4.977 | 0.000 |
(b) C2v | 2.70 | 1.014 | 2.822 | 7.421 | 4.599 | 0.000 | |
(c) D3d | 2.62 | 1.046 | 2.754 | 6.804 | 4.050 | 0.000 | |
13 | (a) C1 | 2.81 | 1.353 | 2.556 | 7.310 | 4.754 | 0.000 |
(b) C1 | 2.78 | 1.310 | 2.585 | 7.329 | 4.744 | 0.000 | |
(c) C1 | 2.60 | 0.554 | 2.913 | 6.951 | 4.038 | 0.000 | |
(d) C6v | 2.72 | 0.517 | 3.115 | 7.122 | 4.007 | 0.000 | |
14 | (a) Cs | 2.79 | 0.950 | 2.889 | 6.396 | 3.507 | 0.000 |
(b) C1 | 2.75 | 1.078 | 2.721 | 7.125 | 4.404 | 0.000 | |
(c) D6h | 2.58 | 0.010 | 2.916 | 6.358 | 3.442 | 0.002 | |
(d) C1 | 2.73 | 1.097 | 2.690 | 7.070 | 4.380 | 0.000 | |
15 | (a) C2 | 2.80 | 1.031 | 3.022 | 7.357 | 4.335 | 0.000 |
(b) C1 | 2.77 | 0.997 | 2.758 | 7.026 | 4.268 | 0.000 | |
(c) Cs | 2.69 | 0.591 | 2.975 | 6.816 | 3.841 | 0.000 | |
(d) C2v | 2.64 | 0.520 | 2.758 | 6.424 | 3.666 | 0.000 | |
16 | (a) C1 | 2.81 | 0.899 | 2.780 | 6.939 | 4.159 | 0.000 |
(b) C1 | 2.80 | 0.994 | 2.953 | 7.069 | 4.116 | 0.000 | |
(c) C2h | 2.74 | 0.537 | 2.571 | 6.348 | 3.777 | 0.000 | |
17 | (a) C1 | 2.80 | 1.332 | 2.664 | 7.136 | 4.472 | 0.000 |
(b) Cs | 2.71 | 1.018 | 2.747 | 6.863 | 4.116 | 0.000 | |
(c) C2v | 2.69 | 1.015 | 2.219 | 6.826 | 4.607 | 0.000 |
Table3.
Symmetry group, binding energy per atom Eb, HOMO-LUMO gap ΔE, vertical electronic affinity (VEA), vertical ionisation potential (VIP), chemical hardness η and total spin magnetic moments μ for SnGen (n = 1–17) clusters.
Table options
-->
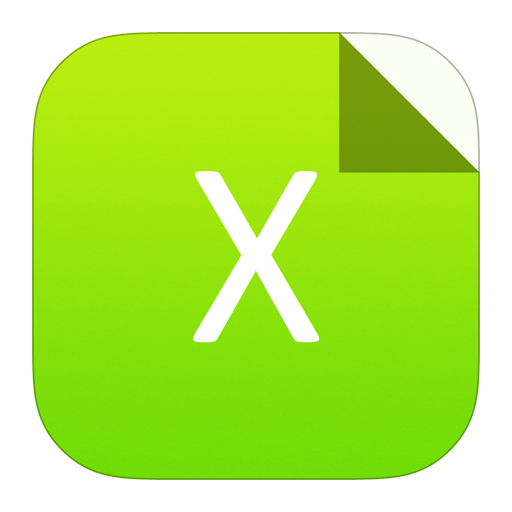
Download as CSV
Size (n) | Symmetry group | Eb (eV/atom) | ΔE (eV) | VEA (eV) | VIP (eV) | η (eV) | μ (μb) |
1 | (a) C∞v | 1.19 | 0.344 | 1.199 | 7.383 | 6.184 | 2.000 |
2 | (a) Cs | 1.81 | 1.311 | 1.441 | 7.982 | 6.541 | 0.000 |
(b) C∞v | 1.71 | 1.643 | 1.264 | 8.719 | 7.455 | 0.000 | |
(c) C2v | 1.80 | 0.984 | 1.702 | 7.936 | 6.234 | 0.000 | |
3 | (a) C2v | 2.20 | 0.227 | 1.715 | 8.039 | 6.324 | 0.000 |
(b) C2v | 1.79 | 1.132 | 2.386 | 7.072 | 4.686 | 0.000 | |
4 | (a) C2v | 2.40 | 1.739 | 1.649 | 8.912 | 7.263 | 0.000 |
(b) C3v | 2.35 | 1.556 | 1.255 | 8.447 | 7.192 | 0.000 | |
(c) Cs | 2.19 | 0.499 | 2.687 | 7.367 | 4.680 | 2.000 | |
(d) C2v | 2.14 | 0.889 | 2.959 | 6.840 | 3.881 | 2.000 | |
5 | (a) Cs | 2.53 | 1.663 | 1.845 | 8.022 | 6.177 | 0.000 |
(b) Cs | 2.48 | 1.450 | 2.056 | 7.798 | 8.742 | 0.000 | |
(c) Cs | 2.44 | 1.228 | 1.873 | 7.786 | 5.913 | 0.000 | |
6 | (a) C1 | 2.63 | 1.853 | 2.064 | 8.241 | 6.177 | 0.000 |
(b) C1 | 2.61 | 1.512 | 2.357 | 8.153 | 5.796 | 0.000 | |
(c) C2v | 2.53 | 1.451 | 1.590 | 7.856 | 6.266 | 0.000 | |
7 | (a) C1 | 2.62 | 1.197 | 2.059 | 7.538 | 5.479 | 0.000 |
(b) C1 | 2.59 | 1.149 | 2.076 | 7.416 | 5.340 | 0.000 | |
(c) Cs | 2.58 | 0.947 | 2.047 | 7.179 | 5.132 | 0.000 | |
8 | (a) C1 | 2.68 | 1.486 | 1.749 | 7.745 | 5.996 | 0.000 |
(b) C1 | 2.68 | 1.515 | 1.978 | 7.425 | 5.447 | 0.000 | |
9 | (a) Cs | 2.77 | 1.551 | 2.306 | 7.714 | 5.408 | 0.000 |
(b) C1 | 2.69 | 1.434 | 2.197 | 7.497 | 5.300 | 0.000 | |
10 | (a) Cs | 2.73 | 1.202 | 2.291 | 7.204 | 4.913 | 0.000 |
(b) C1 | 2.72 | 1.031 | 2.378 | 7.133 | 4.755 | 0.000 | |
(c) C1 | 2.71 | 1.121 | 2.511 | 7.324 | 4.813 | 0.000 | |
11 | (a) C1 | 2.74 | 1.529 | 2.225 | 7.321 | 5.096 | 0.000 |
(b) C5v | 2.70 | 1.155 | 2.583 | 7.388 | 4.805 | 0.000 | |
(c) Cs | 2.67 | 1.104 | 2.667 | 7.359 | 4.692 | 0.000 | |
(d) C1 | 2.64 | 1.027 | 3.628 | 6.242 | 2.614 | 0.000 | |
12 | (a) C1 | 2.73 | 0.992 | 2.230 | 7.207 | 4.977 | 0.000 |
(b) C2v | 2.70 | 1.014 | 2.822 | 7.421 | 4.599 | 0.000 | |
(c) D3d | 2.62 | 1.046 | 2.754 | 6.804 | 4.050 | 0.000 | |
13 | (a) C1 | 2.81 | 1.353 | 2.556 | 7.310 | 4.754 | 0.000 |
(b) C1 | 2.78 | 1.310 | 2.585 | 7.329 | 4.744 | 0.000 | |
(c) C1 | 2.60 | 0.554 | 2.913 | 6.951 | 4.038 | 0.000 | |
(d) C6v | 2.72 | 0.517 | 3.115 | 7.122 | 4.007 | 0.000 | |
14 | (a) Cs | 2.79 | 0.950 | 2.889 | 6.396 | 3.507 | 0.000 |
(b) C1 | 2.75 | 1.078 | 2.721 | 7.125 | 4.404 | 0.000 | |
(c) D6h | 2.58 | 0.010 | 2.916 | 6.358 | 3.442 | 0.002 | |
(d) C1 | 2.73 | 1.097 | 2.690 | 7.070 | 4.380 | 0.000 | |
15 | (a) C2 | 2.80 | 1.031 | 3.022 | 7.357 | 4.335 | 0.000 |
(b) C1 | 2.77 | 0.997 | 2.758 | 7.026 | 4.268 | 0.000 | |
(c) Cs | 2.69 | 0.591 | 2.975 | 6.816 | 3.841 | 0.000 | |
(d) C2v | 2.64 | 0.520 | 2.758 | 6.424 | 3.666 | 0.000 | |
16 | (a) C1 | 2.81 | 0.899 | 2.780 | 6.939 | 4.159 | 0.000 |
(b) C1 | 2.80 | 0.994 | 2.953 | 7.069 | 4.116 | 0.000 | |
(c) C2h | 2.74 | 0.537 | 2.571 | 6.348 | 3.777 | 0.000 | |
17 | (a) C1 | 2.80 | 1.332 | 2.664 | 7.136 | 4.472 | 0.000 |
(b) Cs | 2.71 | 1.018 | 2.747 | 6.863 | 4.116 | 0.000 | |
(c) C2v | 2.69 | 1.015 | 2.219 | 6.826 | 4.607 | 0.000 |
Cluster size (n) | SnGen | SnGen+ | SnGen– | |||||
aGe-Ge | aSn-Ge | aGe-Ge | aSn-Ge | aGe-Ge | aSn-Ge | |||
1 | / | 2.805 | / | 2.910 | / | 2.495 | ||
2 | 2.472 | 2.658 | 2.626 | 2.833 | 2.551 | 2.900 | ||
3 | 2.741 | 2.857 | 2.656 | 2.884 | 2.689 | 2.844 | ||
4 | 2.665 | 2.852 | 2.691 | 3.026 | 2.683 | 2.861 | ||
5 | 2.850 | 2.911 | 2.867 | 2.974 | 2.856 | 3.034 | ||
6 | 2.714 | 2.927 | 2.912 | 3.010 | 2.452 | 3.047 | ||
7 | 2.581 | 3.130 | 2.892 | 3.154 | 2.862 | 3.042 | ||
8 | 2.939 | 3.062 | 2.978 | 3.107 | 2.908 | 2.999 | ||
9 | 2.851 | 3.059 | 2.498 | 3.008 | 3.022 | 2.976 | ||
10 | 2.846 | 3.088 | 3.033 | 3.071 | 2.848 | 3.002 | ||
11 | 2.866 | 3.052 | 2.873 | 3.086 | 2.641 | 2.996 | ||
12 | 2.904 | 3.059 | 2.931 | 3.078 | 2.901 | 3.029 | ||
13 | 2.860 | 3.083 | 2.787 | 3.049 | 2.850 | 3.057 | ||
14 | 2.980 | 3.038 | 2.855 | 3.054 | 2.917 | 2.969 | ||
15 | 2.906 | 2.907 | 2.925 | 2.929 | 2.882 | 2.931 | ||
16 | 2.895 | 3.037 | 3.081 | 3.029 | 2.897 | 3.021 | ||
17 | 2.865 | 2.996 | 2.837 | 2.986 | 2.854 | 3.002 |
Table4.
Average bond length aGe-Ge and aSn-Ge for neutral, cationic and anionic SnGen(0 ± 1) (n = 1–17) clusters.
Table options
-->
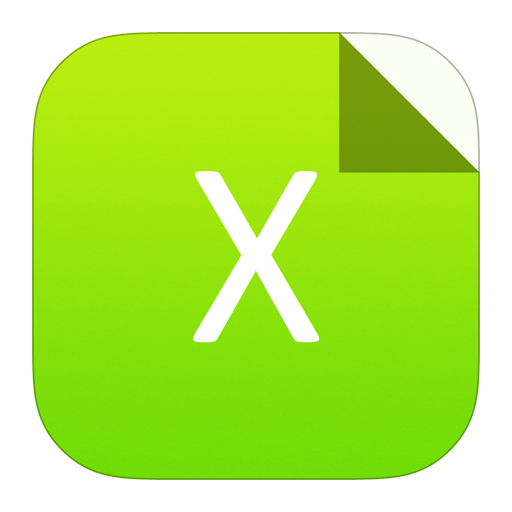
Download as CSV
Cluster size (n) | SnGen | SnGen+ | SnGen– | |||||
aGe-Ge | aSn-Ge | aGe-Ge | aSn-Ge | aGe-Ge | aSn-Ge | |||
1 | / | 2.805 | / | 2.910 | / | 2.495 | ||
2 | 2.472 | 2.658 | 2.626 | 2.833 | 2.551 | 2.900 | ||
3 | 2.741 | 2.857 | 2.656 | 2.884 | 2.689 | 2.844 | ||
4 | 2.665 | 2.852 | 2.691 | 3.026 | 2.683 | 2.861 | ||
5 | 2.850 | 2.911 | 2.867 | 2.974 | 2.856 | 3.034 | ||
6 | 2.714 | 2.927 | 2.912 | 3.010 | 2.452 | 3.047 | ||
7 | 2.581 | 3.130 | 2.892 | 3.154 | 2.862 | 3.042 | ||
8 | 2.939 | 3.062 | 2.978 | 3.107 | 2.908 | 2.999 | ||
9 | 2.851 | 3.059 | 2.498 | 3.008 | 3.022 | 2.976 | ||
10 | 2.846 | 3.088 | 3.033 | 3.071 | 2.848 | 3.002 | ||
11 | 2.866 | 3.052 | 2.873 | 3.086 | 2.641 | 2.996 | ||
12 | 2.904 | 3.059 | 2.931 | 3.078 | 2.901 | 3.029 | ||
13 | 2.860 | 3.083 | 2.787 | 3.049 | 2.850 | 3.057 | ||
14 | 2.980 | 3.038 | 2.855 | 3.054 | 2.917 | 2.969 | ||
15 | 2.906 | 2.907 | 2.925 | 2.929 | 2.882 | 2.931 | ||
16 | 2.895 | 3.037 | 3.081 | 3.029 | 2.897 | 3.021 | ||
17 | 2.865 | 2.996 | 2.837 | 2.986 | 2.854 | 3.002 |
(A) SnGen+ | (B) SnGen- | ||||||||||
Cluster size (n) | Symmetry group | Eb (eV/atm) | ΔE (eV) | μ(μb) | AIP (eV) | Symmetry group | Eb (eV/atm) | ΔE (eV) | μ (μb) | AEA (eV) | |
1 | C∞v | 1.285 | 1.476 | 3.000 | 7.360 | C∞v | 1.819 | 0.247 | 1.000 | 1.451 | |
2 | Cs | 1.761 | 0.410 | 1.000 | 7.706 | Cs | 2.365 | 0.601 | 1.000 | 1.850 | |
3 | C2v | 2.156 | 0.674 | 1.000 | 7.729 | C2v | 2.640 | 0.990 | 1.000 | 1.948 | |
4 | C2v | 2.316 | 0.405 | 1.000 | 7.971 | C2v | 2.833 | 0.930 | 1.000 | 2.357 | |
5 | C2v | 2.515 | 1.027 | 1.000 | 7.624 | C2v | 2.878 | 0.892 | 1.000 | 2.300 | |
6 | C2v | 2.597 | 0.530 | 1.000 | 7.796 | C2v | 2.956 | 1.284 | 0.996 | 2.460 | |
7 | C1 | 2.655 | 0.894 | 0.999 | 7.252 | C1 | 2.909 | 0.827 | 1.000 | 2.525 | |
8 | C1 | 2.711 | 0.420 | 0.998 | 7.277 | C1 | 2.975 | 0.430 | 0.998 | 2.844 | |
9 | Cs | 2.760 | 0.372 | 0.997 | 7.597 | Cs | 3.007 | 0.669 | 0.999 | 2.611 | |
10 | C1 | 2.775 | 0.944 | 0.997 | 7.012 | Cs | 2.945 | 0.376 | 0.999 | 2.596 | |
11 | C1 | 2.772 | 0.430 | 0.992 | 7.158 | C1 | 2.934 | 0.376 | 0.994 | 2.521 | |
12 | C1 | 2.771 | 0.435 | 0.995 | 7.086 | C1 | 2.958 | 0.061 | 0.997 | 3.100 | |
13 | C1 | 2.836 | 0.535 | 0.992 | 7.139 | C1 | 2.985 | 0.174 | 0.976 | 2.682 | |
14 | Cs | 2.828 | 0.650 | 0.999 | 6.939 | Cs | 2.992 | 0.426 | 0.985 | 3.244 | |
15 | C2 | 2.829 | 0.635 | 0.994 | 7.046 | C2 | 2.991 | 0.446 | 0.945 | 3.278 | |
16 | C1 | 2.854 | 0.343 | 0.991 | 6.824 | C1 | 2.978 | 0.151 | 0.966 | 3.022 | |
17 | C1 | 2.834 | 0.194 | 0.969 | 6.960 | C1 | 2.963 | 0.122 | 0.976 | 3.110 |
Table5.
(A) Symmetry group, binding energy per atom Eb (eV/atom), HOMO-LUMO gap ΔE (eV), total spin magnetic moments μ(μB), adiabatic ionisation potential (AIP) (eV) for cationic SnGen+ (n = 1?17) clusters. (B) Symmetry group, binding energy per atom Eb, HOMO-LUMO gap ΔE, total spin magnetic moments μ, adiabatic electron affinity (AEA) for anionic SnGen– (n = 1?17) clusters.
Table options
-->
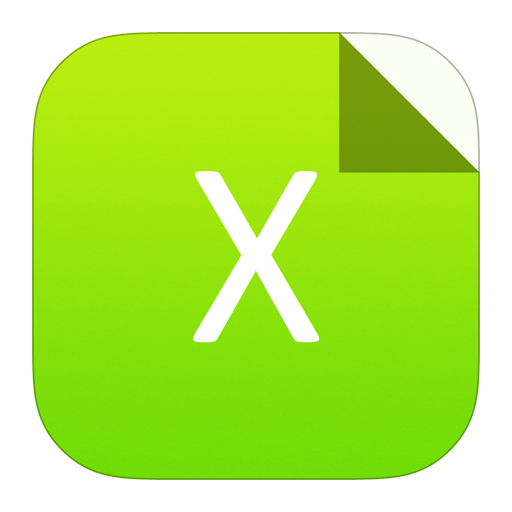
Download as CSV
(A) SnGen+ | (B) SnGen- | ||||||||||
Cluster size (n) | Symmetry group | Eb (eV/atm) | ΔE (eV) | μ(μb) | AIP (eV) | Symmetry group | Eb (eV/atm) | ΔE (eV) | μ (μb) | AEA (eV) | |
1 | C∞v | 1.285 | 1.476 | 3.000 | 7.360 | C∞v | 1.819 | 0.247 | 1.000 | 1.451 | |
2 | Cs | 1.761 | 0.410 | 1.000 | 7.706 | Cs | 2.365 | 0.601 | 1.000 | 1.850 | |
3 | C2v | 2.156 | 0.674 | 1.000 | 7.729 | C2v | 2.640 | 0.990 | 1.000 | 1.948 | |
4 | C2v | 2.316 | 0.405 | 1.000 | 7.971 | C2v | 2.833 | 0.930 | 1.000 | 2.357 | |
5 | C2v | 2.515 | 1.027 | 1.000 | 7.624 | C2v | 2.878 | 0.892 | 1.000 | 2.300 | |
6 | C2v | 2.597 | 0.530 | 1.000 | 7.796 | C2v | 2.956 | 1.284 | 0.996 | 2.460 | |
7 | C1 | 2.655 | 0.894 | 0.999 | 7.252 | C1 | 2.909 | 0.827 | 1.000 | 2.525 | |
8 | C1 | 2.711 | 0.420 | 0.998 | 7.277 | C1 | 2.975 | 0.430 | 0.998 | 2.844 | |
9 | Cs | 2.760 | 0.372 | 0.997 | 7.597 | Cs | 3.007 | 0.669 | 0.999 | 2.611 | |
10 | C1 | 2.775 | 0.944 | 0.997 | 7.012 | Cs | 2.945 | 0.376 | 0.999 | 2.596 | |
11 | C1 | 2.772 | 0.430 | 0.992 | 7.158 | C1 | 2.934 | 0.376 | 0.994 | 2.521 | |
12 | C1 | 2.771 | 0.435 | 0.995 | 7.086 | C1 | 2.958 | 0.061 | 0.997 | 3.100 | |
13 | C1 | 2.836 | 0.535 | 0.992 | 7.139 | C1 | 2.985 | 0.174 | 0.976 | 2.682 | |
14 | Cs | 2.828 | 0.650 | 0.999 | 6.939 | Cs | 2.992 | 0.426 | 0.985 | 3.244 | |
15 | C2 | 2.829 | 0.635 | 0.994 | 7.046 | C2 | 2.991 | 0.446 | 0.945 | 3.278 | |
16 | C1 | 2.854 | 0.343 | 0.991 | 6.824 | C1 | 2.978 | 0.151 | 0.966 | 3.022 | |
17 | C1 | 2.834 | 0.194 | 0.969 | 6.960 | C1 | 2.963 | 0.122 | 0.976 | 3.110 |
This structure is in agreement with Samanta et al.[1] results. For the charged cluster, the capped tetrahedron structure is the most stable structure with C2v symmetry. The energy binding of SnGe4+ is 2.316 eV/atom, which is smaller than that of SnGe4– (2.833 eV/atom). The capped trigonal bipyramid with (C2v) symmetry is obtained for Ge6 cluster, in agreement with the result of Li et al.[39] For n = 5, SnGe5 (a) is the most stable isomer with Cs symmetry. Their corresponding Ge–Ge and Sn–Ge bonds length are 2.850 and 2.911 ?, respectively. The same form is obtained by Wang and Han[42]. The capped trigonal bipyramid configuration with C2v is also stable for SnGe5+. However, the anion SnGe5– is a distorted octahedron with (C2v) symmetry. For Ge7, the pentagonal bipyramid with D5h symmetry and the lowest binding energy of 2.66 eV/atom is suggested. Our results are in good agreement with previous theoretical studies[3, 12, 39]. Three different isomers are found for SnGe6. The most stable one is SnGe6 (a) with Cs symmetry, bond lengths Ge–Ge and Sn–Ge of 2.714 and 2.927 ?, respectively. The pentagonal bipyramid is the most stable structure for SnGe6– and SnGe6+. For n = 8, the face-capped pentagonal bipyramid is obtained for pure Ge8 cluster with Cs symmetry, binding energy of 2.64 eV/atom and average bond length of 2.796 ?. The same structure is reported by Wang et al.[12] A similar structure as Ge8 is obtained in the case of SnGe7 and SnGe7± with C1 symmetry. The Ge–Ge bond length is much larger in the case of SnGe7+ and SnGe7– (2.892 and 2.862 ?) clusters compared to the corresponding neutral SnGe7 cluster (2.581 ?). For Ge9, the bicapped pentagonal bipyramid Ge9 (a) with C2v symmetry is the most stable structure with binding energy of 2.70 eV/atom and average bond length Ge–Ge of 3?. In the corresponding doped cluster, the most stable structure is SnGe8 (a) with Cs symmetry. Its Ge–Ge and Sn–Ge average distances are 2.939 and 3.062 ?, respectively. The same structure and symmetry is found for cationic SnGe8+. However, the anionic SnGe8– cluster presents a different structure with C1 symmetry. The lowest energy structure, among the three best structures observed in the case of Ge10 cluster, is Ge10 (a) with C3v symmetry, which is in good agreement with Wang et al.[12] results. In the doped clusters case, the ground state structure is SnGe9 (a) with Cs symmetry. The Sn atom occupies a peripheral position. The average Ge–Ge and Sn–Ge distances are 2.851 and 3.059 ?, respectively. The results indicate that the SnGe9+ and SnGe9– structure are completely different to the neutral SnGe9 structure. The average Ge–Ge distance of cationic cluster 0.353 ? is smaller than that in the neutral SnGe9. For Ge11, the most stable structure is Ge11 (a) with Cs symmetry. Substituting Ge by Sn leads to a somewhat similar structure. The ground state structure of SnGe10 (a) is given in Fig. 2 with Cs symmetry. Its calculated binding energy is 2.73 eV/atom, while the bond lengths Ge–Ge and Sn–Ge are 2.846 and 3.088 ?, respectively. We observe that the obtained results suggest that the majority lowest energy clusters of pure germanium are layered structure beginning n = 12. In Fig. 1, we show that the ground state structure of Ge12 has stacked structure with C2v symmetry. Substituting Ge by Sn does not perturb significantly the structure because the structure of SnGe11 is very similar to that of Ge12. The binding energy of SnGe11 is only 0.02 eV/atom, which is less than that of Ge12. The stacked structure is also stable for SnGe11+ species with C1 symmetry. The layered structure has been found also for Ge13. It consists in a triangle Ge3 and bicapped square antiprism Ge10, in 1-5-4-3 layers. Similar layered structure Ge14 (a) is obtained for Ge14 with 1-5-4-4 layered. In this case, the Ge3 unit of the best isomer Ge13 is replaced by a rhombus Ge4. The lowest energy structures of Ge15, Ge16, and Ge17 are stacked structures with 1-5-3-5-1, 1-5-4-5-1 and 1-5-5-5-1 layers, respectively. These layered structures have also been found by Wang et al.[12]. Two isomers with spherical configuration and one Ge core atom are found for Ge16 and Ge18, as shown in Fig. 1. The most stable structure Ge18 (a) of Ge18 consists in three connected pentagonal parties Ge7, dimer Ge2 and capped tetragonal prism Ge9 but this isomer is not a layered structure. In all the SnGen isomers clusters, with n < 12 size, the Sn atom occupies a peripheral position. In n > 12 case, the Sn atom can occupy a core position. For example in SnGe 12, Sn occupies a peripheral position in SnGe12 (a) structure and occupies a core position in SnGe12 (b) and SnGe12 (c) structures. Compared to other isomers, SnGe12 (a) structure has the highest stability. For charged SnGe12±, we obtained the same structures with the same symmetry C1 and Sn–Ge bond length for cation and anion clusters of 3.078 and 3.029 ?, respectively. Two stacked structures (a) and (b) and hexagonal prism (c) are optimized for SnGe13. The calculated results show that the SnGe13 (a) isomer is the most stable geometry and have the same form with pure germanium Ge14. The stacked structure is also stable for SnGe13±. SnGe14 (a) structure, where the Sn atom is located at the surface, is similar to Ge15 structure. Two other isomers (b) and (c), with Sn atom at the center of the structure, are also found for SnGe14. The same structure with Cs symmetry, have been obtained for SnGe14+ and SnGe14–. SnGe15 have four stable structures. The most stable one is the SnGe15 (a) with Cs symmetry, which is obtained by substitution of Ge atom by Sn atom in the Ge16 cluster. The other isomers SnGe15 (b, c, d) have spherical structures with Sn atom in surface for (b) and in a core position for (c, d). The SnGe15 (a) structure is conserved for the cationic SnGe15+ cluster. SnGe16 and SnGe16± have the same stable structure Ge17 (a) with C1 symmetry, SnGe16 has two other isomers. Three SnGe17 isomers are shown in Fig. 2. The SnGe17 (a) isomer has the same form of Ge18 and it is the most stable structure. The SnGe17 (b) cluster is similar spherical structure and SnGe17 (c) cluster is spherical structure, Sn atom is located in the centre position in both structures. The same structure as SnGe17 (a) is found for cationic SnGe17+ with C1 symmetry. Different forms of anionic SnGe17– cluster are obtained.

class="figure_img" id="Figure2"/>
Download
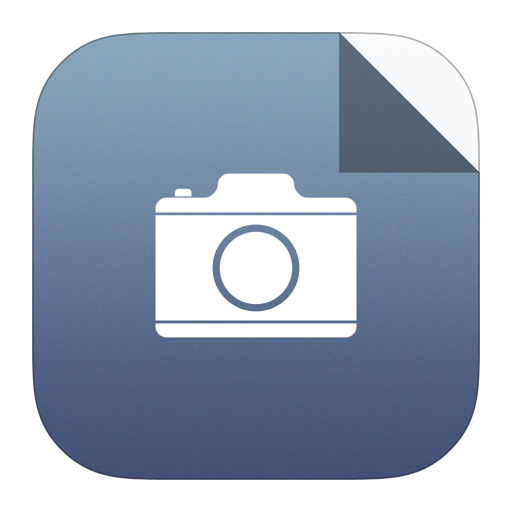
Larger image
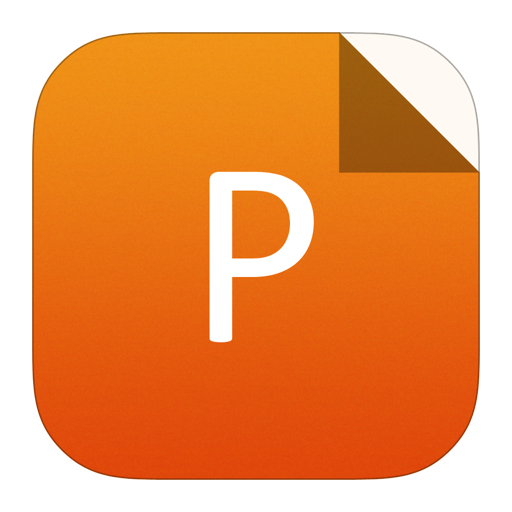
PowerPoint slide
Figure2.
(Color online) Ground state structures and their corresponding isomers for SnGen (n = 1–17) clusters.

class="figure_img" id="Figure1"/>
Download
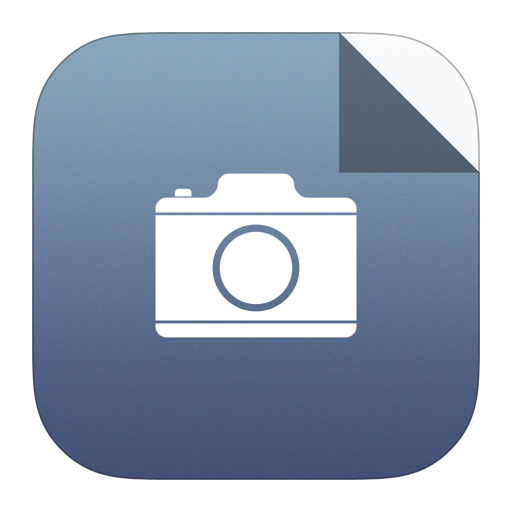
Larger image
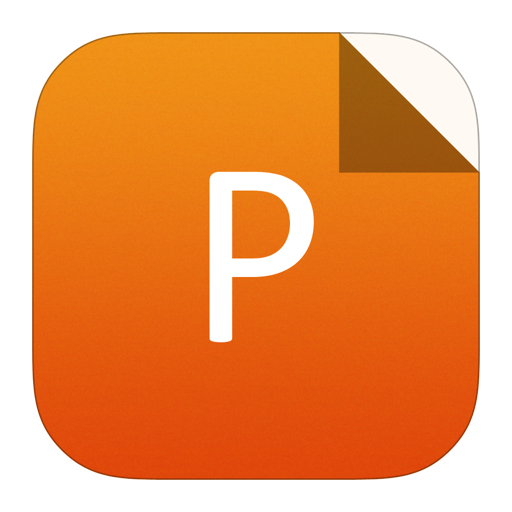
PowerPoint slide
Figure1.
(Color online) Ground state structures and their isomers for Gen+1 (n = 1–17) clusters.
3.2
Electronic properties
In order to explore the relative stability of different species, we calculate the binding energy per atom for the Gen+1 and SnGen(0±1) (n = 1–17) clusters. The binding energy per atom (Eb/atom) is considered, in cluster science, as a sensitive quantity that reflects the relative stability. The different values of Eb/atom for the lowest energy structures are calculated as:
$${E_{ m{b}}}left( {{ m{G}}{{ m{e}}_n}_{ + 1}} ight){ m{ }} = { m{ }}left[ {left( {n + 1} ight)Eleft( {{ m{Ge}}} ight) - Eleft( {{ m{G}}{{ m{e}}_n}_{ + 1}} ight)} ight]/left( {n + 1} ight),$$ ![]() | (1) |
$${E_{ m{b}}}left( {{ m{SnG}}{{ m{e}}_n}} ight){ m{ }} = { m{ }}[nEleft( {{ m{Ge}}} ight){ m{ }} + Eleft( {{ m{Sn}}} ight){ m{ }} - E({ m{SnG}}{{ m{e}}_n} )] /left( {n + 1} ight),$$ ![]() | (2) |
$$begin{split}{E_{ m{b}}}left( {{ m{SnG}}{{ m{e}}_n}^{ - 1}} ight) = &[left( {n - 1} ight)Eleft( {{ m{Ge}}} ight) + Eleft( {{ m{Sn}}} ight) &+ Eleft( {{ m{G}}{{ m{e}}^{ - 1}}} ight) - Eleft( {{ m{SnG}}{{ m{e}}_n}^{ - 1}} ight)]/left( {n + 1} ight),end{split}$$ ![]() | (3) |
$${E_{ m{b}}}left( {{ m{SnG}}{{ m{e}}_n}^{ + 1}} ight){ m{ }} =left[ { m{ }}nEleft( {{ m{Ge}}} ight){ m{ }} + Eleft( {{ m{S}}{{ m{n}}^{ + 1}}} ight){ m{ }} - Eleft( {{ m{SnG}}{{ m{e}}_n}^{ + 1}} ight) ight]/left( {n + 1} ight).$$ ![]() | (4) |
where E(Ge), E(Sn), E(Ge-1) and E(Sn+1) are the total energy of free Ge, Sn, Ge– and Sn+ atoms, respectively. E(Gen+1) is the total energy of Gen+1, E(SnGen) is the total energy of SnGen and E(SnGen±) is the total energy cationic and anionic SnGen± clusters. The obtained results of binding energies per atom for pure Ge and neutral and charged tin-doped germanium clusters are given in Tables 2, 3 and 5, respectively and their variations versus the cluster size are plotted in Fig. 4. For all species, the binding energy per atom increases with the cluster size. This behavior implies that the cluster stability is enhanced whenever the cluster size increased. The graphs show a thermodynamic instability of smaller clusters. In the case of SnGen clusters, the binding energy per atom increases significant increasing in the average binding energies of the clusters of small size range (n < 9), which is due to the rapidly from 1.19 eV/atom in SnGe cluster to 2.77 eV/atom in SnGe 9. For clusters of size n > 9, we can see a slow increasing in Eb to reach the saturation plateau at 2.8 eV/atom. From Fig. 4, we also observe that there is a high competition in the evolution of the binding energies between SnGen and its corresponding Gen+1 cluster. This means that the Sn atom haven’t an immediate and direct effect on the relative stability of pure germanium clusters.

class="figure_img" id="Figure4"/>
Download
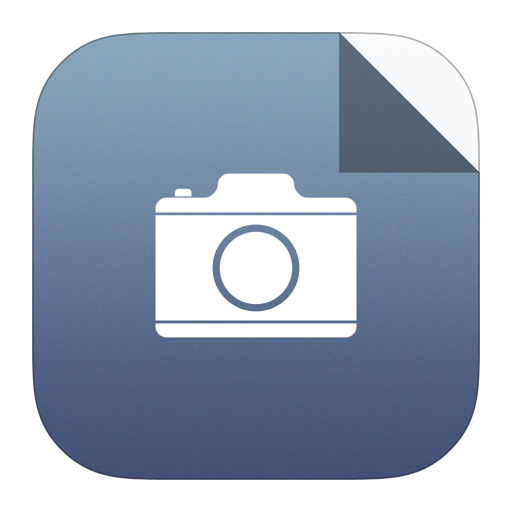
Larger image
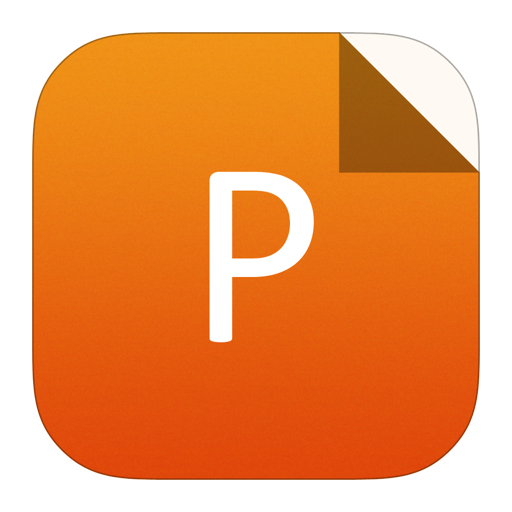
PowerPoint slide
Figure4.
(Color online) Size dependence of the binding energies of Gen+1 and SnGen(0, ±1) (n = 1–17) clusters.
The anionic clusters exhibit high stabilities compared to the others’ cationic and neutral ones. Local peaks are observed in the curve of binding energies of Gen+1 and SnGen for n = 6, n = 9 and n = 13. This means that the corresponding clusters are more stable than their neighbors.
On the other hand, we calculated the fragmentation energy which is considered as a good criterion for predicting the relative stability of the clusters for spontaneous fragmentation. In this works, the size dependence of the fragmentation energies (EF) for Gen+1 and SnGen(0, ±1) clusters are studied. The fragmentation energy values for different clusters can be calculated by using the following formulas:
$${E_{ m{F}}}left( {{ m{G}}{{ m{e}}_n}_{ + 1}} ight){ m{ }} = Eleft( {{ m{G}}{{ m{e}}_n}} ight) + Eleft( {{ m{Ge}}} ight) - Eleft( {{ m{G}}{{ m{e}}_n}_{ + 1}} ight),$$ ![]() | (5) |
$$begin{split}{E_{ m{F}}}left( {{ m{SnG}}{{ m{e}}_n}^{(0 pm 1)}} ight) =& Eleft( {{ m{SnG}}{{ m{e}}_n}^{(0 pm 1)}} ight)& + Eleft( {{ m{Ge}}} ight) - Eleft( {{ m{SnG}}{{ m{e}}_n}^{(0 pm 1)}} ight).end{split}$$ ![]() | (6) |
where E(Ge), E(Gen+1) and E(SnGen(0±1)) are the total energy of free Ge atom, Gen+1 cluster and SnGen(0±1) cluster respectively. Based on the above formulas, the calculated fragmentation energy values and their evolution with the cluster size are shown in Fig. 5. We observe that there are oscillating behaviors with an odd–even decreasing tendency in the evolution of fragmentation energy for all the species. Two parts can be distinguished for Gen+1 and SnGen clusters. For n < 9, the clusters with n even values are more stable than n odd values. However, for n > 9, the clusters with n odd values are more stable than n even values. This confirms that the size n = 9 is the stability transition size of these two species. Local maxima of the fragmentation energy of Gen+1, SnGen, SnGen+ and SnGen? clusters appear at Ge4, 10, 14, SnGe3, 9, 13, 16, SnGe+3, 5, 13, 16 and SnGe?4, 6, 8, 13, respectively, which indicates that these clusters are more stable than their neighbors.

class="figure_img" id="Figure5"/>
Download
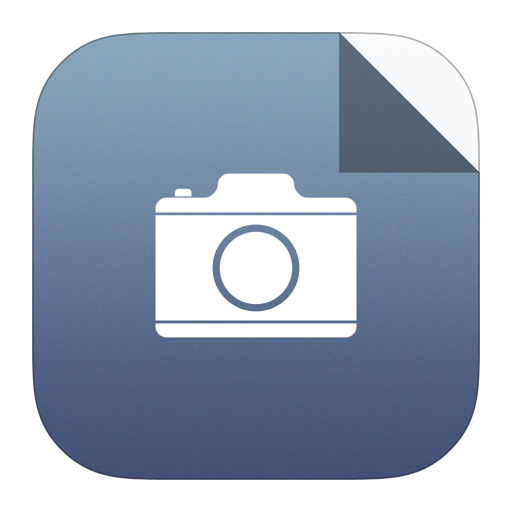
Larger image
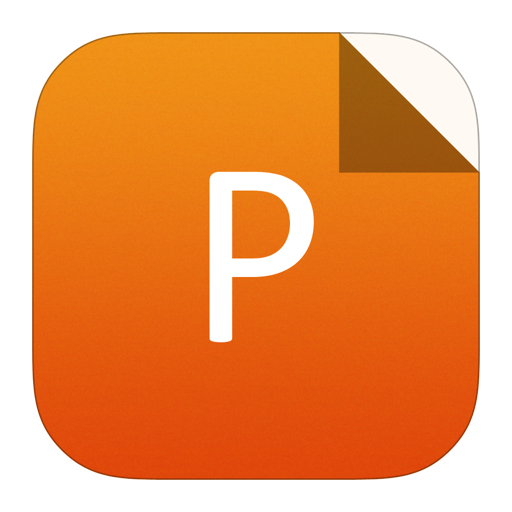
PowerPoint slide
Figure5.
(Color online) Size dependence of the fragmentation energy of Gen+1 and SnGen(0, ± 1) (n = 1–17) clusters.
In order to analyze the relative stability of Gen+1 and SnGen(0±1) clusters, we calculate second order difference of total energy (Δ2E). For the ground state structures the second difference energies for all species are defined by:
$${Delta _2}Eleft( {{ m{G}}{{ m{e}}_n}_{ + 1}} ight) = Eleft( {{ m{G}}{{ m{e}}_n}_{ + 2}} ight){ m{ }} + Eleft( {{ m{G}}{{ m{e}}_n}} ight){ m{ }} - { m{ }}2Eleft( {{ m{G}}{{ m{e}}_n}_{ + 1}} ight),$$ ![]() | (7) |
$$begin{split}{Delta _2}Eleft( {{ m{G}}{{ m{e}}_n}_{ + 1}} ight) =& Eleft( {{ m{SnG}}{{ m{e}}_{n{ m{ + }}1}}^{(0 pm 1)}} ight) &+ Eleft( {{ m{SnG}}{{ m{e}}_{n - 1}}^{(0 pm 1)}} ight) - 2Eleft( {{ m{SnG}}{{ m{e}}_n}^{(0 pm 1)}} ight),end{split}$$ ![]() | (8) |
where E is the total energy of corresponding cluster. It is very known in cluster physics that the small systems with positive value of Δ2E are more stable than the systems with negative value of Δ2E. The calculated values of Δ2E and their evolution as function of the n size for Gen+1 and SnGen(0±1) are shown in Fig. 6. From Fig. 6, the very pronounced peaks are observed at the sizes n = 1, 5, 7, 10, 12, 14 for pure Gen+1 and SnGen clusters, which demonstrate that the clusters corresponding for these sizes are more stable compared to their neighbors for both species. The explanation of this behavior can be given with a direct relationship between the stability of Sn-doped germanium and their corresponding pure Gen+1 with the same size. Except for the two size n = 7 and n = 10, we observe that the structure SnGen clusters kept unchanged after the encapsulation of Sn atom in pure germanium cage. We also observe that the local peaks at sizes n = 2, 4, 12 and n = 7, 10, 11 for SnGen+ and SnGen? clusters, respectively indicating that the corresponding clusters have a higher relative stability compared to neighboring clusters.

class="figure_img" id="Figure6"/>
Download
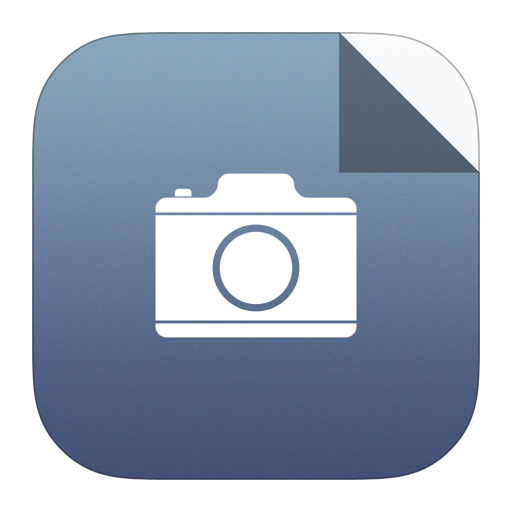
Larger image
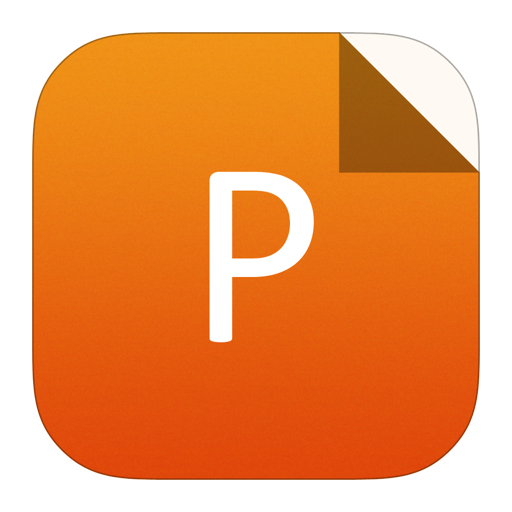
PowerPoint slide
Figure6.
(Color online) Second energy differences for Gen+1 and SnGen(0, ± 1) (n = 1–17) clusters.
In order to understand the chemical reactivity and kinetic stability of the clusters, we calculate the energy differences between the highest occupied and lowest unoccupied molecular orbital (HOMO-LUMO) of the Gen+1 and SnGen(0, ±1) clusters. In Fig. 7, we show the evolution of the HOMO-LUMO gaps as a function of the size for the ground states of different species studied in this piper. We observe a high oscillation of HOMO-LUMO gaps values for the very small size clusters (n < 4). From n = 4 this behavior decreases when the size increases. Many local maxima are observed at n = 2, 4, 8, 9, 11, 13, 15, 17 for both pure and doped germanium clusters, which indicates that these clusters are less reactive than their neighbors and have high chemical stability. The HOMO-LUMO gap of SnGe3 is lower than that of Ge4, which indicates that the doping Sn atom enhance the chemical activity of the cluster. We note that the cluster of tin-doped germanium at n = 6 have a large value of HOMO-LUMO gap, which indicates that SnGe6 is the most chemically stable structure and can be used as the building blocks in many new nanomaterials with specific properties. The ionization of SnGen leads to a considerable reduction of the HOMO-LUMO gaps compared to the case of neutral clusters. This indicates that the chemical activity of charged SnGen± clusters is higher than that of neutral SnGen clusters. Moreover, the clusters of Ge2, SnGe1, 3 and SnGe–12, 13, 16, 17 have small values of HOMO-LUMO gaps, which indicates that they have partially metallic character.

class="figure_img" id="Figure7"/>
Download
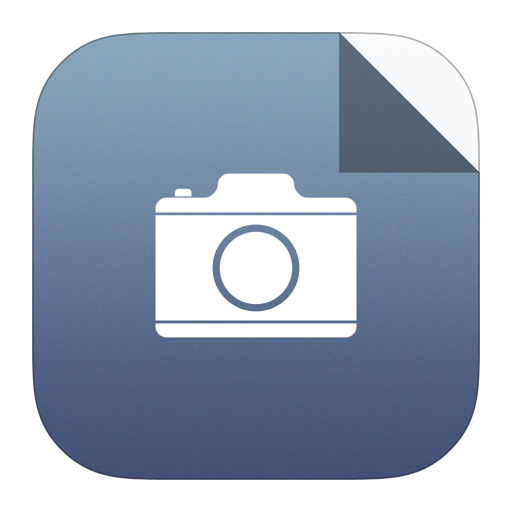
Larger image
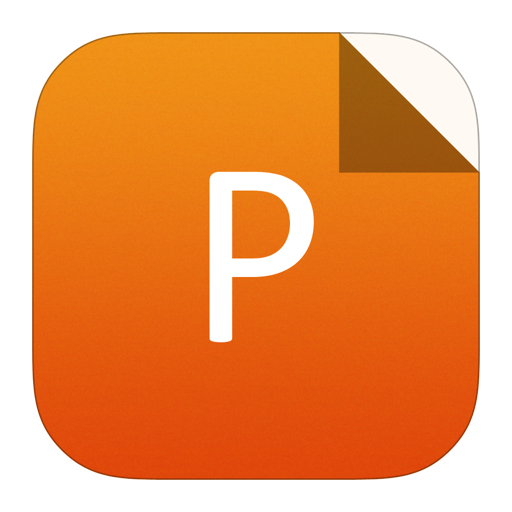
PowerPoint slide
Figure7.
(Color online) Size dependence of the HOMO-LUMO gaps of Gen+1 and SnGen(0, ± 1) (n = 1–17) clusters.
In cluster physics, the ionization potential and electron affinity are considered as suitable parameters to determine the stability of clusters and reflecting the variation of the electronic structure with the size. In order to determine the required energies to add or remove an electron from the lowest energy structures of neutral SnGen clusters, we have calculated the adiabatic electron affinity and adiabatic ionization potential, taking in the account the structural relaxation. The vertical ionization potential and vertical electron affinity are respectively the energy required to remove or add an electron on the neutral clusters without structural relaxation.
The ionization potential (IP) is an important character in understanding electronic properties of clusters and giving more information about clusters metallic character. From Fig. 8(a), we can see that VIP and AIP values decrease as the cluster size the increase. It is well known that when the IP is small, the cluster will be closer to a metallic character. This means that the clusters of SnGen with size more than 9 atoms exhibit a high metallic character. The smallest VIP and AIP values are observed in SnGe14, 16 clusters, indicating that these clusters are more readily ionized than the others. The highest value of SnGe4 VIP can be explained by its related symmetry. The calculated AEA and VEA of SnGen clusters are presented in Fig. 8(b). We can see that the electron affinity increases whenever the cluster size increases. Consequently, the SnGen clusters with large sizes will liberate more energy when they capture one electron. We also observe some maximal local peaks in this graph at n = 8, 12, 14 for AEA and n = 7, 9, 15 for VEA, which means that these clusters are energetically less stable than their neighbors.

class="figure_img" id="Figure8"/>
Download
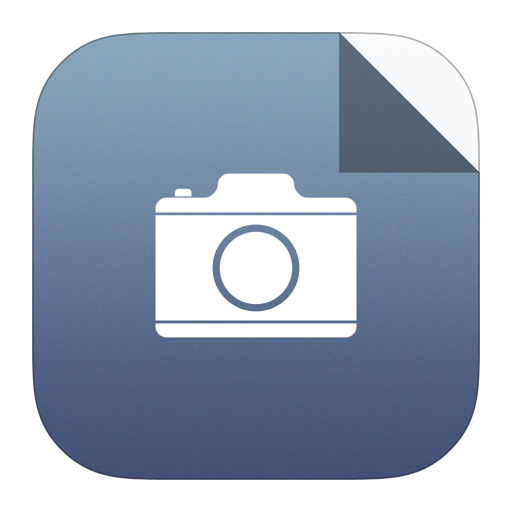
Larger image
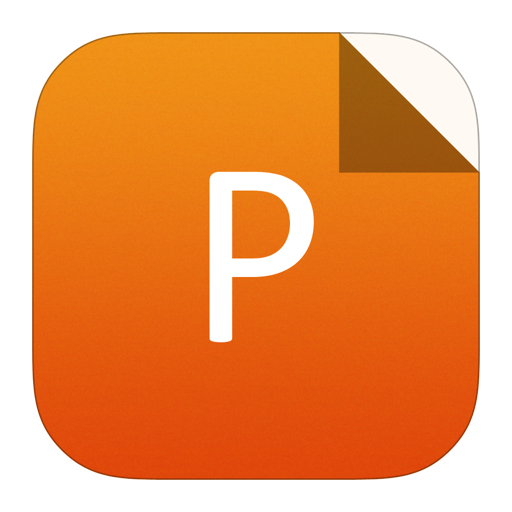
PowerPoint slide
Figure8.
(Color online) (a) Vartical (VIP) and adiabatic (AIP) ionization potential; (b) Vartical (VEA) and adiabatic (AEA) electron affinity, (c) chemical hardness for SnGen (n = 1 – 17) clusters.
On the other hand, the chemical hardness (η) of cluster can be examined in order to understand its chemical stability. A large value of η indicates that corresponding clusters are less reactive[43, 44]. For the ground state structures, the chemical hardness is evaluated by using the following formula:
$${ m{eta = VIP - VEA}}.$$ ![]() | (9) |
The obtained results for the most stable structures of SnGen clusters are listed in Table 3 and plotted in Fig. 8(c) as a function of the size n. We observe that the chemical hardness of SnGen clusters decreases when the size increases. This means that clusters with large size are less reactive and more stable than clusters with small size. We can also see from graph that the SnGe4 cluster has the highest chemical hardness value, which indicates that this cluster is more stable than its neighbors. In addition, other local peaks at n = 2, 6 and 8 for SnGen are less reactive than the other clusters.
3.3
Magnetic properties
One of the most important properties that make a special characteristic of these small clusters is their magnetic behavior. Indeed, we can observe very small clusters with very specific magnetic response that can have many important applications in nanotechnologies.
In our case, the magnetic properties are evaluated by the assessment of the total spin magnetic moment for each cluster size. It is defined as the difference between the total Mullikan charge populations for electrons with spin up and electrons with spin down. The calculated total spin magnetic moment values of SnGen clusters are reported in Table 3. We observe that all the ground states of SnGen clusters are generally nonmagnetic structures, except the case of SnGe1, which have a total spin magnetic moment of 2μb. In order to explore this specific case we plot the total and the partial densities of states for SnGe1 cluster (DOS and PDOS) (Fig. 9). We observe, from the figure, that the total spin magnetic moment in SnGe monomer is mainly due to the 4p and 5p orbital of Ge and Sn atoms, respectively. In the case of the anionic and cationic SnGen clusters, the obtained total spin magnetic moments have been reported in Table 4. We observe that their total spin magnetic moments of ionized structures are different compared to those obtained in neutral structures and they are generally equal to 1μb. This means that the charge of the systems can affect the magnetic response of the tin doped germanium clusters.

class="figure_img" id="Figure9"/>
Download
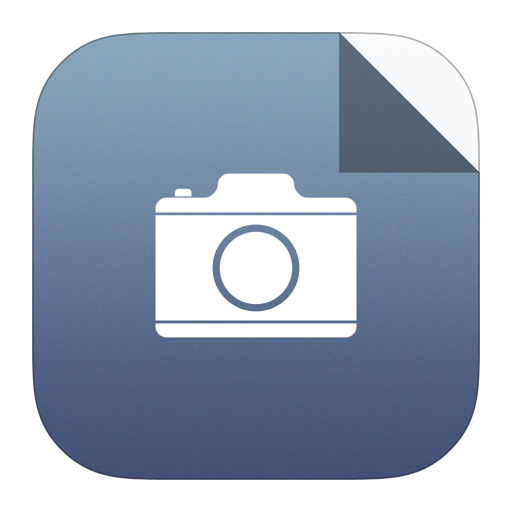
Larger image
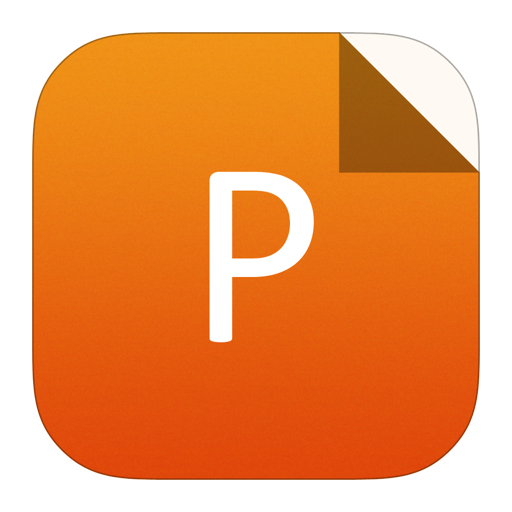
PowerPoint slide
Figure9.
(Color online) The total density of states (DOS) for SnGe monomer and the projected density of states (PDOS) for Sn and Ge atoms in SnGe.
4.
Conclusion
In this work, the geometry, stability, electronic and magnetic properties of the neutral and charged SnGen clusters have been systematically investigated by the first principles DFT calculations. All the Gen+1 and SnGen(0, ±1) clusters adopt planar structures for very small size and near spherical and spherical compact structures as the size increase. In SnGen(0, ±1) clusters, the Sn atom prefers the peripheral position when n < 12 and occupies a core position for n > 12. The structure SnGe n clusters kept unchanged after the encapsulation of Sn atom in pure germanium cage. For Gen+1 and SnGen(0, ±1) clusters, the binding energy per atom increases with the increasing of the size. The strong increasing in stability of the small clusters is related to the thermodynamic instability of smaller systems. The fragmentation energy calculations indicates that the clusters Ge4, 10, 14 and SnGe3, 9, 13, 16 and SnGe+3, 5, 13, 16 are more stable than their neighbors. The second energy difference analysis shows that the clusters of Gen+1 and SnGen clusters at n = 1, 5, 7, 10, 12, 14 are more stable. The HOMO-LUMO gaps of all systems showed a decreasing behavior with the increasing of the size from n = 4 and the charged clusters showed a considerable reduction of the HOMO-LUMO gaps compared to the case of neutral ones. The ionization potentials and electron affinity calculations showed that the clusters of SnGen with a size more than 9 atoms exhibit high metallic character and will liberate more energy when they capture one electron. However, the chemical hardness of SnGen showed that the clusters with large size are less reactive and more stable. All of the ground states of SnGen clusters are nonmagnetic structures, except the case of SnGe1. Their total spin magnetic moment is mainly due to the 4p and 5p orbital of Ge and Sn atoms, respectively. All these specific properties make the SnGen clusters good potential candidates for many eventual applications in nanotechnologies. We have to mention that it is very interesting for the future to calculate the frequencies of vibration in order to obtain the best and more stable isomers.
Acknowledgements
The authors are thankful to the SIESTA group for providing their computational code and greatly acknowledge for LENREZA Laboratory, Ouargla University, Algeria.