1.
Introduction
Semiconducting oxides such as ZnO, SnO2 and In2O3 have been proved to be an important class of transparent conducting oxides (TCO) for applications in solar cells[1], gas sensors[2], thin film transistor (TFT)[3] and flat panel display[4]. Among all these semiconducting oxides, SnO2 is one of the suitable candidates for these applications due to its wide band gap (3.6 eV) and electrically semiconducting nature[5]. SnO2 is the only oxide of group IV which exhibits the TCOs properties i.e. transparency in visible range and good conductivity[6]. The coexistence of these two properties in SnO2 is mainly due to the presence of defects[7], the main defects arise due to oxygen vacancy and Sn interstitial atoms. Furthermore, it is known that the formation of defects depends upon the formation (enthalpy) energy which results in the creation of free charge carriers[6] in the matrix of the material. Moreover, defects in pure semiconducting oxides play the key role in the overall electrical and optical properties as the number of defects inside the material decides the value of carrier concentration (n), electrical mobility (μ)[8] and optical band gap of the material. The electrical conductivity, carrier concentration and mobility in TCO thin films can be controlled (e.g. in case of TCOs range of carrier concentration is 1016–1020 cm?3[9, 10]) along with the desired level of optical transmission in the UV, visible and infrared region of the electromagnetic spectrum. This can be done by considering different deposition parameters in different deposition techniques[11].
In the literature, SnO2 thin films have been deposited by using different deposition techniques viz. RF sputtering[5, 12], electron beam evaporation[13, 14], spin coating[15], molecular beam epitaxy[16] etc. It is observed that there is a significant change in the physical properties of the films deposited with different deposition methods[17]. As in the case of electron beam evaporation method, it is observed that the deposition pressure in the chamber affects the crystallinity as well as the composition of the film[18, 19]. Prathyusha et al.[20] reported that the SnO2 thin films deposited by DC magnetron sputtering at different bias voltage exhibit a change in the composition of the films. Minami et al.[21] deposited the SnO2 thin films by RF magnetron sputtering with variation in the pressure and obtained the lowest resistivity at a gas pressure of 0.399 to 0.666 Pa. Another research group[3] deposited a very thin channel layer film of SnO2 by RF magnetron sputtering and annealed the films at 600 °C to obtain significant enhancement in carrier mobility from 0.8 to 2.0 cm2/(V·s) for various thicknesses of the films. Deposition of SnO2 thin films by pulsed laser deposition and a variation of band gap with different film thicknesses is also reported in a literature report[22]. Khan et al.[14] calculated the band gap of 4.22 eV for the 200 nm thick crystalline SnO2 thin film deposited by electron beam evaporation at the deposition rate of 0.45 nm/s. He et al.[23] deposited SnO2 thin film by metal organic chemical vapor deposition (MOCVD) method at different substrate temperatures and observed that along with the better mobility (21 cm2/(V·s)), the optical transmission in the visible range was ~ 93%. It is understood that every deposition technique is distinguished according to its specific method of thin film deposition and can be optimized in order to obtain the desired properties of thin films. So it is worth mentioning here that the desired properties of the deposited thin films may be tuned by altering different deposition parameters during the deposition of the thin films. One more important aspect in case of semiconducting oxides is that these oxides show the Mott transition due to which at a certain value of carrier concentration, these oxides give the best compromise between optical and electrical properties[8] for transparent electrode applications. In the wake of such optimized value of electrical and optical properties, we deposited SnO2 thin films by electron beam evaporation method for three different deposition powers which is considered to change the deposition rate and formation energy of defects inside the films. It is observed that these defects result in different concentrations of oxygen vacancies, carriers and mobility in the films. Furthermore, the change in deposition power leads to different activation energies which are used to understand the relation between electrical properties and intrinsic defects, and in turn with other properties for transparent electrode applications. To the best of our knowledge, there has been limited discussion of the variation in the properties of SnO2 thin films with different deposition rate due to different deposition powers.
2.
Experimental details
The target of 10 mm diameter for deposition was prepared with 99.99% pure SnO2 powder (Alfa Aesar) followed by sintering at 1000 °C for 10 h. All the substrates were thoroughly cleaned by acetone, propanol and followed by ultrasonic cleaning for 15 min. The SnO2 thin films were deposited by electron-beam deposition method three times with variation in deposition powers to change the deposition rate while all other parameters were kept the same. The deposition powers were taken to be 50, 75, and 100 W, which are obtained by keeping voltage as constant (4.75 kV) and varying the current during the deposition. The distance between the target and substrate holder was ~20 cm during the deposition. The films deposited at 50, 75, and 100 W are named as SnO-50, SnO-75, and SnO-100 respectively.
The as-deposited films were found to be amorphous in nature; therefore, all the films were annealed at 600 °C for 5 h[7, 24]. The crystalline structure of the films was confirmed by X-ray diffraction (XRD, Bruker D8-X-ray diffractometer) using CuKα radiation with wavelength, λ = 1.54 ?. The surface morphology of the films is studied by scanning electron microscopy (SEM, JEOL), which is operated at a voltage of 30 kV. In order to find the thickness of SnO2 thin film and the concentration of Sn and O ions, the Rutherford back scattering (RBS) available at IUAC, New Delhi with 2 MeV He+ was used. The optical absorption of the SnO2 films is studied by using the UV–Vis spectrophotometer (Hithachi, U-3300) in the wavelength range of 200–900 nm. The Hall measurements in the films are done by using the ECOPIA Hall measurement system.
3.
Results and discussion
3.1
Structural properties
The XRD patterns of the SnO2 thin films are shown in Fig. 1. These are recorded at a glancing angle of 3° and the diffraction angle range is taken from 20° to 55°. The diffraction peaks are indexed with JCPDS Card No. 41-1445 and indicate the formation of crystalline thin films of tetragonal SnO2 thin films with (110) peak having the highest intensity, which is consistent with its bulk counterpart. The XRD results also reveal that the deposited SnO2 thin films are polycrystalline in nature. Furthermore, it can be elucidated that the growth of thin film has taken place according to the grain model. Moreover, the orientation of the grains is not present along any fixed direction and the phases are formed in a different direction, which confirms the granular formation of films. It is also observed that the intensity and sharpness of the diffraction peaks increase as the deposition rate increases in SnO-75 and SnO-100 as compared to SnO-50. The average crystalline size (D) is calculated for the (110) peak using the following Debye-Scherrer formula,

class="figure_img" id="Figure1"/>
Download
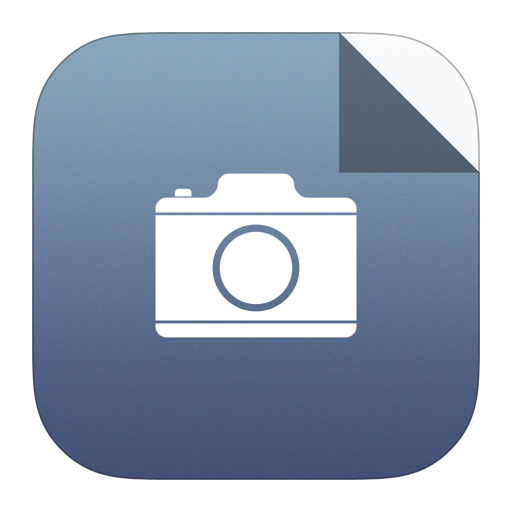
Larger image
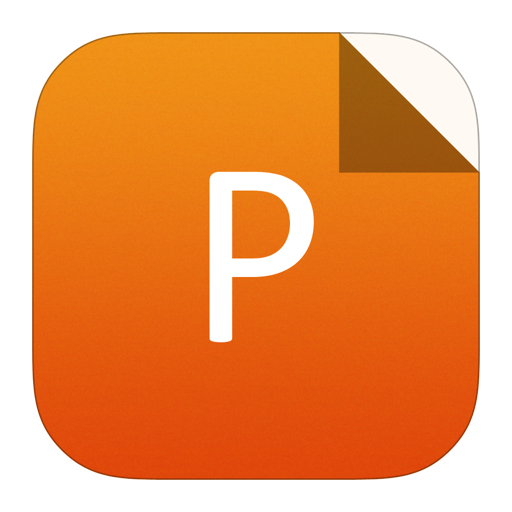
PowerPoint slide
Figure1.
(Color online) XRD pattern of films deposited at 50 W (SnO-50), 75 W (SnO-75), and 100 W (SnO-100).
$${{D}} = frac{{0.9{ m{lambda }}}}{{{ m{beta costheta }}}},$$ ![]() | (1) |
where λ is the wavelength of X-rays, β is full width at half maximum (FWHM) and θ is the half of diffraction angle for this peak. The crystallite sizes are calculated as 21, 19, and 15 nm for SnO-50, SnO-75 and SnO-100 respectively, which decreases as the sharpness of the films increases from SnO-50 to SnO-100. Moreover, the lattice constants for SnO2 thin films are also calculated and presented in Table 1.
Sample | a = b (?) | c (?) | Crystallite size (nm) |
SnO-50 | 4.744 | 3.187 | 21 |
SnO-75 | 4.741 | 3.188 | 19 |
SnO-100 | 4.747 | 3.190 | 15 |
Table1.
The change in lattice constants is attributed to the lattice strain produced by the variation in deposition power. This also results in change in particle size and formation of oxygen vacancies[25] in the matrix of the films.
Table options
-->
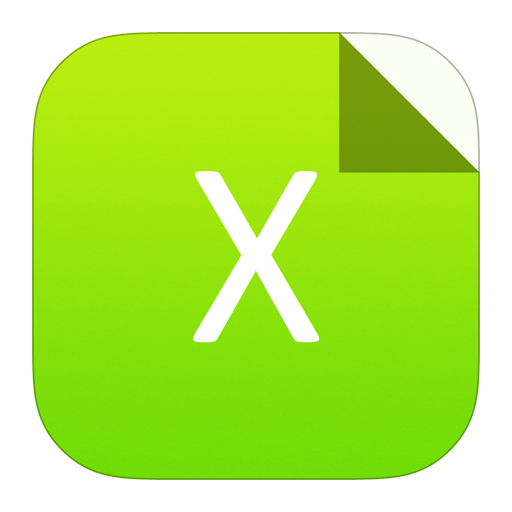
Download as CSV
Sample | a = b (?) | c (?) | Crystallite size (nm) |
SnO-50 | 4.744 | 3.187 | 21 |
SnO-75 | 4.741 | 3.188 | 19 |
SnO-100 | 4.747 | 3.190 | 15 |
3.2
Surface morphological study
The SEM images of SnO2 thin films shown in Fig. 2 exhibit the clusters composed of grains. The morphology of SnO-50, SnO-75 and SnO-100 are shown in Figs. 2(a)–2(c) respectively. It is seen that the sizes of grains are not homogeneous which in turn suggest that the growth of grains is in the form of islands. Furthermore, film SnO-50 also has inhomogeneous grain growth, which becomes a little homogenous by increasing the deposition power to 75 W in the case of film SnO-75. It is seen from Fig. 2(c) that there is an agglomeration of grains for the film SnO-100. The surface morphological results support that with high deposition power, the thickness as well as the grain growth increases in the films. It is also understood that the change in defects thermodynamic (i.e. the defects formation energy) for all the thin films, results in the creation of different grain sizes[26]. The sample SnO-100 has the lowest grains of size (~30 nm), which is in accordance with the result reported in Ref. [27].

class="figure_img" id="Figure2"/>
Download
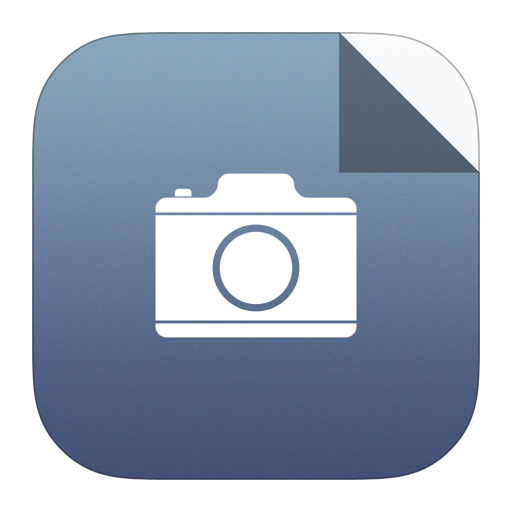
Larger image
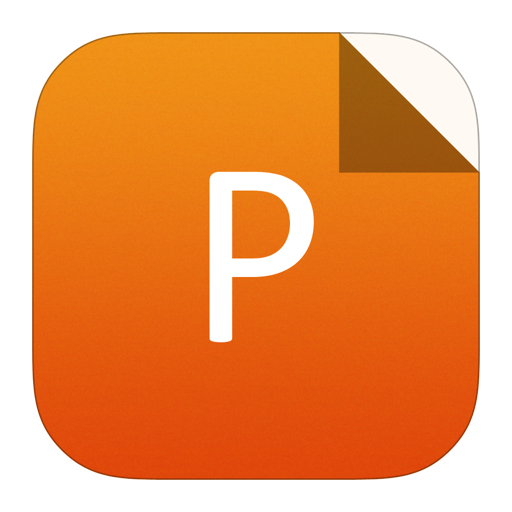
PowerPoint slide
Figure2.
SEM images of thin films. (a) SnO-50. (b) SnO-75. (c) SnO-100.
3.3
RBS spectra
The RBS spectra provide the details about the depth profile and elemental concentration in the films and are shown in Fig. 3(a) for SnO-50, SnO-75, and SnO-100. This is also used to find the thickness of the films by fitting the spectra with the help of simulation software (X-RUMP). The fitted spectra of the film SnO-100 are shown in Fig. 3(b). The simulation results which calculate the thickness and elemental concentration in films SnO-50, SnO-75, and SnO-100 are presented in Table 2. It is elucidated from the RBS spectra of the films that there is diffusion of SnO2 into the Si substrate, which is known to be a normal process during the thin film deposition[28].

class="figure_img" id="Figure3"/>
Download
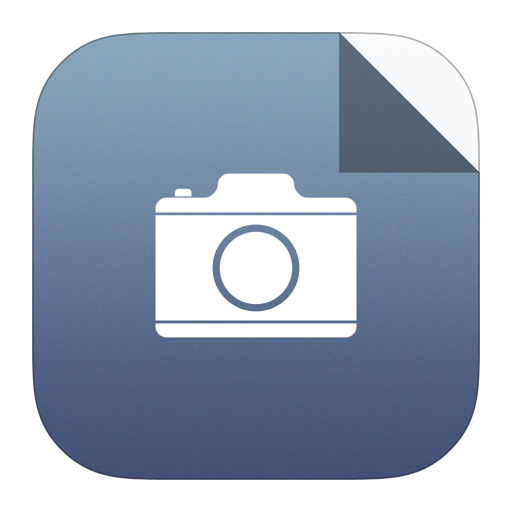
Larger image
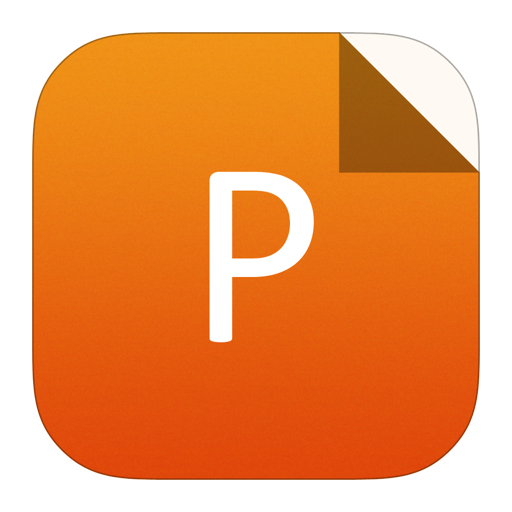
PowerPoint slide
Figure3.
(Color online) (a) RBS spectra of SnO-50, SnO-75, and SnO-100 thin films. (b) Simulated RBS spectrum of film SnO-100 using X-Rump software. The fitted curve is shown in red.
Sample | SnO-50 | SnO-75 | SnO-100 |
Thickness (nm) | 200 | 250 | 400 |
Concentration (Sn) | 1 | 1 | 1 |
Concentration (O) | 1.7 | 1.9 | 2.0 |
Table2.
Simulated results of RBS spectra for SnO-50, SnO-75, and SnO-100 films.
Table options
-->
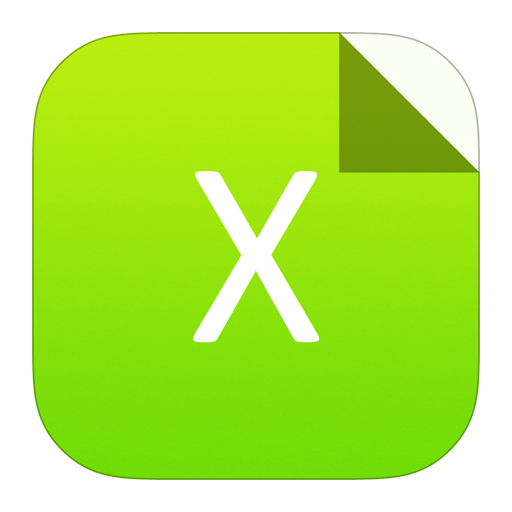
Download as CSV
Sample | SnO-50 | SnO-75 | SnO-100 |
Thickness (nm) | 200 | 250 | 400 |
Concentration (Sn) | 1 | 1 | 1 |
Concentration (O) | 1.7 | 1.9 | 2.0 |
Table 2 depicts that there is an increase in film thickness with increasing the deposition power in the films from SnO-50 to SnO-100, which is understood to be due to the increase in the deposition rate with deposition power during the thin film deposition. Furthermore, the elemental concentration of tin (Sn) atoms is found to be the same in all samples, however the elemental concentration of oxygen (O) atoms increases with the increase in deposition rate. It is elucidated that in the case of the lowest deposition power i.e. in SnO-50, the growth of film is composed of adatoms leaving the oxygen vacancies, whereas at the highest deposition power (in SnO-100) the growth of film is composed of an island with no oxygen vacancy in this film. This can also be correlated with the calculated difference in crystallite and grain size as studied from XRD and SEM results.
3.4
Optical properties
The optical absorption spectra of SnO2 thin films is taken by UV–Vis spectroscopy in the wavelength range of 200–900 nm and is shown in Fig. 4. It is observed that the absorption of all the films is found to be very much less in the visible region, which means that the films were highly transparent in the visible region. There was found to be a variation in the transition wavelength for the films. This is used to calculate the variation in the band gap of the films. In order to determine the band gap, a plot of (αhν)2 versus hν (Tauc’s plot) is used. The following relation is used to find the band gap by plotting the corresponding Tauc plot, which is shown in Fig. 5.
$${(alpha hv)^2} = hv - {E_{ m g}},$$ ![]() | (2) |
where Eg is the band gap of material, hv is the energy of photons and α is the absorption coefficient.
It is known that the fundamental absorption corresponding to the optical transition of the electrons from the valence band to the conduction band can be used to determine the nature and value of the band gap of films. Furthermore, it is reported in the literature that the band gap in SnO2 generally ranges from 3.7 to 3.9 eV, which increases if the films are annealed at high temperature[29]. The values of the band gaps in SnO-50, SnO-75, and SnO-100 are found to be in the reported range.
It is seen from Fig. 4(a) that the absorption edge is found to be shifted towards the longer wavelength region (red shift) when films SnO-50 and SnO-100 are considered. However, this shift is found to be the maximum in film SnO-75, which is inconsistent with the variation in other films. This is primarily attributed to comparatively lower absorption of photons in the case of films SnO-75 and SnO-100 in comparison to film SnO-50. The reason behind this lower absorption is the larger thickness of the SnO-75 and SnO-100 films as compared to film SnO-50. Another reason is given in terms of the number of oxygen vacancies in the matrix of the films. As seen in the RBS spectral analysis, the oxygen vacancies are the maximum in film SnO-50, which consecutively decreases in SnO-75 and SnO-100 films. This is further correlated with the energy states in the conduction bands, which are filled by the electrons provided by the oxygen vacancies. This results in the maximum optical band gap of film SnO-50 and in turn results in the lowest transition wavelength in the absorption spectra. However, when the films SnO-75 and SnO-100 are compared, the optical band gap is found to be more in SnO-100 as compared to SnO-75 [Fig. 4(b)], however the oxygen vacancies are more in SnO-75 than SnO-100. This discrepancy in the absorption behavior in case of SnO-75 may be attributed to the microstructural nature of film SnO-75, which basically affects the band structure of the film and in turn affects the overall optical properties of the film. This behavior is also consistent with the electrical property variation, which is discussed in the subsequent sections. Therefore, it can be concluded that the defect density as well as the microstructure of the films are responsible for the variation of optical properties of the films. Moreover, in case of sample SnO-100 with thickness 400 nm, the amount of absorption is lower as there are no free electrons due to the oxygen vacancies and the absorption is primarily due to the large thickness in this film. However, in case of SnO-75 the film thickness is 250 nm hence the contribution in absorption due to the thickness is lower as compared to that due to free charge carriers. This fact can further be explained with the help of skin depth calculations, which are calculated and represented in Fig. 6. The detailed analysis of these calculations is discussed in the last section. It is concluded that the higher skin depth in film SnO-75 results in excess loss of energy. In case of SnO-50, there is a deficiency of oxygen resulting in a large number of free charge carriers as concluded from the RBS spectra analysis. These free carriers are populated at the conduction band minimum, which results in the highest band gap of this film[30]. However, it is found that the intensity of the absorption spectrum at higher wavelength is in order. For the application as a transparent electrode for different applications, there is a requirement of higher transmission, hence in order to get the desired transmission, the deposition rate in terms of change in deposition power during thin film deposition may play a great role.

class="figure_img" id="Figure4"/>
Download
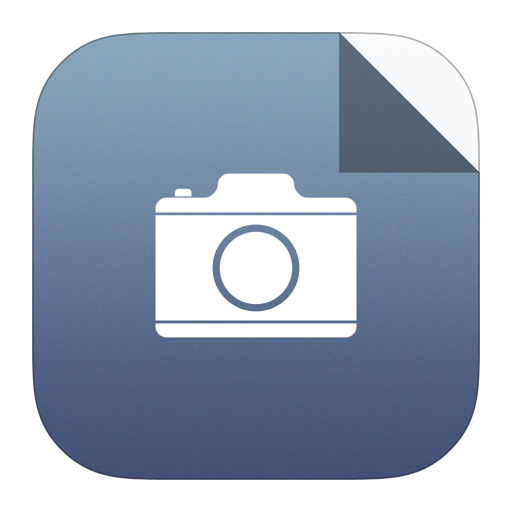
Larger image
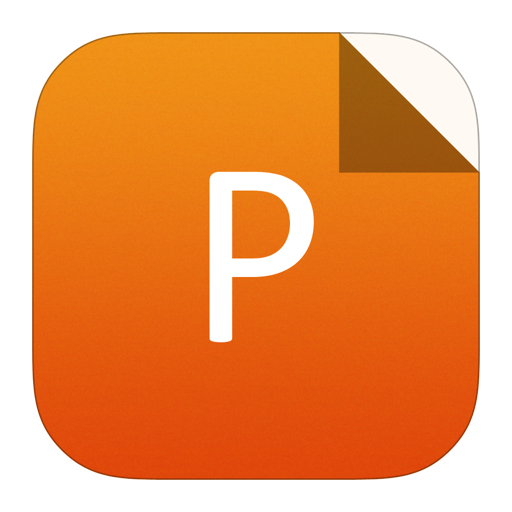
PowerPoint slide
Figure4.
(Color online) (a) Absorption spectra of SnO2 thin films. (b) Tauc plots for SnO-50, SnO-75, and SnO-100 for band gap calculation.
3.5
Electrical properties
3.5.1
Hall effect measurements
The Hall effect measurements in SnO-50, SnO-75, and SnO-100 were carried out by using the Ecopia Hall Measurement system and the results show the n-type semiconducting behavior of all the films. It is observed that the carrier concentrations are found in decreasing order from SnO-50 to SnO-100 and consequently the resistivity of the films increases. The values of carrier concentrations, mobility and resistivity are presented in Table 3. However, there is unusual behavior in the case of electron mobility in SnO-75 film, which is less as compared to SnO-50 while it is also less as compared to SnO-100 film. This behavior is understood to be due to the microstructure of the film as seen in the case of SEM results. Furthermore, there may be any other parameter that is responsible for this behavior as the mobility depends upon many physical quantities, which needs further investigations in future. It is also observed that the mobility in film SnO-100 is higher than in film SnO-50, which is understood by the fact that the higher concentration of charges leads to lower mobility in SnO-50[12] and microstructure will also affect the value when the two films are compared.
This may further be explained with the help of electron Bloch wave function that describes the motion of an electron inside a lattice. According to the theory, if the concentration of carriers i.e. electrons are higher, the mean free path in the lattice will be small and the electron will have low relaxation time resulting in low mobility, which is given by
$$mu = frac{{etau }}{{{m^*}}},$$ ![]() | (3) |
where m* is the effective mass of electron, and τ is the relaxation time.
The film SnO-100 is characterized with a less populated conduction band minimum in comparison to film SnO-50. Therefore, to further understand the transport mechanism, the electron mean free path is calculated by free electron gas theory[31] considering the fact that electron scattering by grain boundary affects the resistivity in polycrystalline materials. For comparison purposes, the SnO2 material is considered as a degenerate semiconductor for the calculations. It is assumed that the Fermi velocity is a constant and the Fermi surface is spherical in nature. The Fermi energy Ef is given by,
$${E_{ m{f}}} = frac{{{h^2}}}{{8m{pi ^2}}}{left( {3N{{{pi }}^2}} ight)^{2/3}},$$ ![]() | (4) |
where m is the mass of electron and N is the electron density.
The Fermi velocity can be calculated from the expression given below,
$${V_{ m f}} = sqrt {frac{{2{E_{ m f}}}}{m}} .$$ ![]() | (5) |
The electron mean free path l is given by,
$$l = {V_{ m f}} tau,$$ ![]() | (6) |
where τ is the relaxation time.
The electron mean free paths in SnO-50, SnO-75, and SnO-100 are calculated using Eqs. (3)–(6) above, while the value of mobility and carrier concentration is taken from Hall effect measurements (Table 3). The values of Fermi energy, Fermi velocity and electron mean free path for films SnO-50, SnO-75, and SnO-100 are presented in Table 3.
As the grain sizes (revealed from XRD and SEM) are decreasing from SnO-50 to SnO-100, accordingly the electronic mean free path is decreasing due to the decrease in the relaxation time. Therefore, it can be concluded that there is a size effect on electrical conductivity and hence the scattering is dominated by lattice scattering in the case of SnO-100 but in the other two cases the scattering is dominating equally by lattice as well as by defects.
3.5.2
Resistance versus temperature
The electrical behavior with respect to the temperature is recorded with the help of the two probes method and the results are shown in Fig. 5. All the films are found to be semiconducting in nature. It is observed that the resistance in the case of SnO-50 film is the lowest and it is the highest in the case of SnO-100. The reason for the same is understood to be due to the variation of oxygen vacancies in the films. Moreover, the thicknesses of the films are also increasing with deposition rate as seen by the simulation of RBS spectra of the films[32]. The values of electrical resistivity are calculated and are given in Table 3.

class="figure_img" id="Figure5"/>
Download
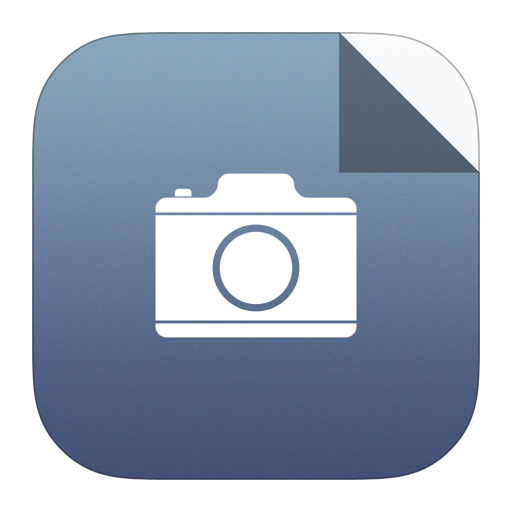
Larger image
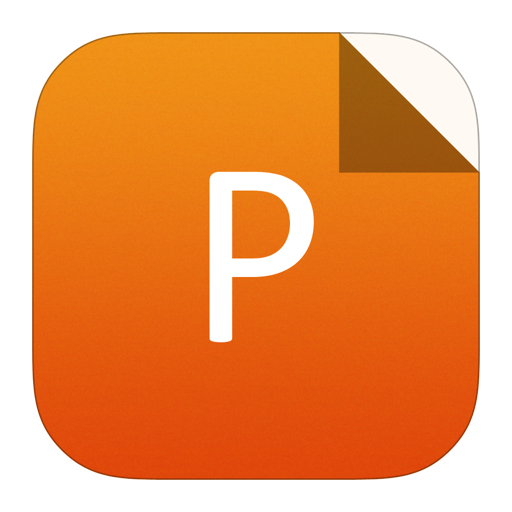
PowerPoint slide
Figure5.
(Color online) Temperature versus resistance plot of SnO2 thin films deposited at different power.
The semiconducting behavior of the films is further explained with the help of the mobility of the charge carriers. The expression for mobility is given by[33],
$$mu propto {mu _{ m b}}{left[ {1 + {m_{ m d}}exp left( {frac{{q{textit{Φ} _{ m f}}}}{{kT}}} ight)} ight]^{ - 1}},$$ ![]() | (7) |
where md is the defect density, q is the electronic charge, Φf is the potential at the stacking fault and μb is the bulk mobility. This equation predicts that there is a decrease in the mobility value with an increase in the defect density and temperature; therefore, the resistivity of SnO2 thin films also depends on crystal defects[34], which decrease with the improvement in the crystal structure of the film. In this study, the variation of the electrical properties of the films can be explained according to the above equation. As the density of charge carriers increases with oxygen vacancies, i.e. increase in the non-stoichiometry of the SnO2 phase, this results in a decrease in the electrical resistivity of the film. Furthermore, the activation energy is calculated by using the following equation
$$sigma = {sigma _0}{ m {exp}}left( {frac{{2{E_{ m a}}}}{{kT}}} ight),$$ ![]() | (8) |
where Ea is the activation energy and the values are calculated to be ~ 0.34, 0.29 and 0.04 eV for SnO-50, SnO-75 and SnO-100 films respectively. The different values of activation energies of SnO2 thin films allow the diffusion of oxide into the silicon substrate, as seen in RBS spectra as well, and decide the thickness of the SiO2 layer after the thermal oxidation.
4.
Skin depth calculations
To understand the correlation between the optical and electrical properties of SnO2 thin films, the skin depth is calculated using the free electron gas theory based on Lorentz Drude’s theory[8] since SnO2 is a degenerate semiconductor. It is known that the propagation vector of electromagnetic wave (light) is a complex quantity, while the wave interacts with conducting or semiconducting material and can be written as,
$${ k} = {k_1} - {text{i}}{k_2} = omega sqrt {varepsilon /c} ,$$ ![]() | (9) |
where ε is the dielectric function and is given as
$$varepsilon = {varepsilon _1} - i{varepsilon _2}.$$ ![]() | (10) |
The skin depth is given by,
$$delta = frac{1}{{{k_2}}}.$$ ![]() |
From Eqs. (5) and (6),
$${ m{delta }} = { m{lambda }}{left( {frac{2}{{sqrt {({ m{varepsilon }}_{1}^2} + { m{varepsilon }}_2^2) - {varepsilon _1}}}} ight)^{1/2}},$$ ![]() | (11) |
where ε1 and ε2 are given by
$$begin{split}& {varepsilon _1} = {varepsilon _infty } - frac{{omega _{ m N}^2{tau ^2}}}{{1 + {omega ^2}{tau ^2}}},& {{ m{varepsilon }}_2} = frac{{omega _{ m N}^2{tau ^2}}}{{1 + {omega ^2}{tau ^2}}}.end{split}$$ ![]() | (12) |
Using the above equations, the skin depth is calculated for wavelength 400 nm (which correspond to higher energy) for SnO2, ε = 3.9 and m* = 0.25 me[34, 35]. The relaxation time τ is calculated from the mobility equation, μ = eτ/m* whereas carrier concentration n and mobility μ are available from the Hall Effect measurements listed in Table 3. Fig. 6 shows the variation of conductivity and skin depth with deposition powers for films SnO-50, SnO-75, and SnO-100.

class="figure_img" id="Figure6"/>
Download
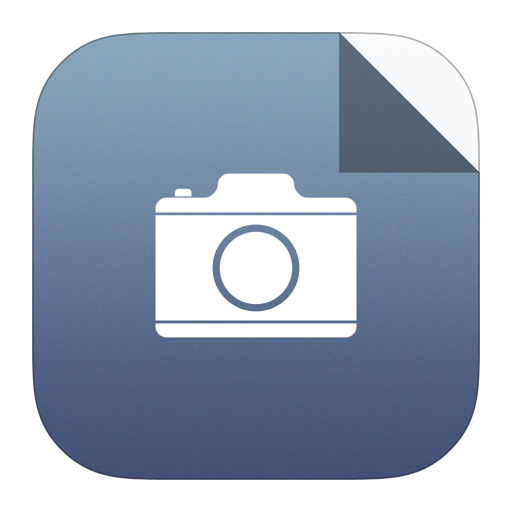
Larger image
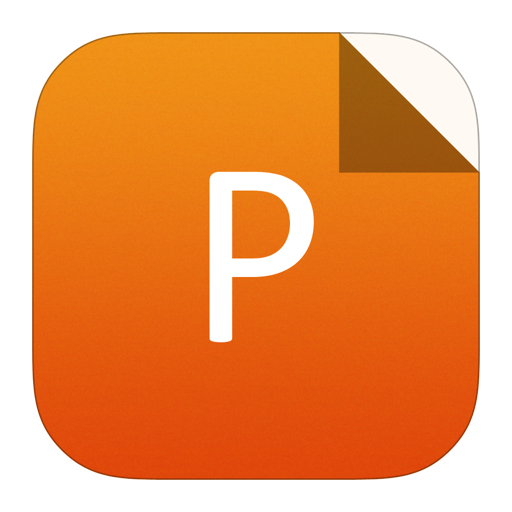
PowerPoint slide
Figure6.
(Color online) The variation of conductivity and skin depth with the deposition power.
Physical quantity | SnO-50 | SnO-75 | SnO-100 |
Carrier concentration (1018 cm?3) | 4.615 | 4.346 | 1.018 |
Mobility (cm2/(V·s)) | 4.848 | 3.972 | 5.700 |
Resistivity (Ω·cm) | 0.279 | 0.3616 | 1.076 |
Fermi energy (eV) | 0.0162 | 0.0155 | 0.006 |
Fermi velocity (104 m/s) | 6 | 5 | 0.04 |
Mean free path (nm) | 16.2 | 12.76 | 0.12 |
Table3.
The results of Hall Effect, resistance measurements and calculated values of Fermi energy, Fermi velocities and electron mean free path for SnO-50, SnO-75, and SnO-100.
Table options
-->
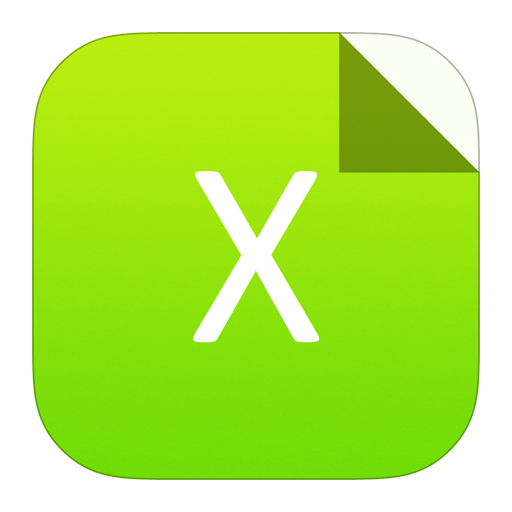
Download as CSV
Physical quantity | SnO-50 | SnO-75 | SnO-100 |
Carrier concentration (1018 cm?3) | 4.615 | 4.346 | 1.018 |
Mobility (cm2/(V·s)) | 4.848 | 3.972 | 5.700 |
Resistivity (Ω·cm) | 0.279 | 0.3616 | 1.076 |
Fermi energy (eV) | 0.0162 | 0.0155 | 0.006 |
Fermi velocity (104 m/s) | 6 | 5 | 0.04 |
Mean free path (nm) | 16.2 | 12.76 | 0.12 |
This variation provides a powerful tool to examine a meaningful comparison of electrical and the optical properties and can explore the further possibility to optimize the physical quantities affecting the required properties for any TCOs like carrier concentration, mobility etc. As in the present case SnO2 in pure phase have the value of n above the critical carrier concentration for Mott transition (nc = 9.5 × 1017 for SnO2)[17], which is due to oxygen vacancies, the skin depth is higher, hence the further increment in carrier concentration n to increase the electrical conductivity will not affect the desired optical properties.
5.
Conclusion
In this work, thin films of SnO2 are deposited by electron beam evaporation for different deposition powers for changing the deposition rate of the films. The structural properties are found to be improved with increasing the deposition power. The microstructural property of the film deposited for highest power is found to be the best. The concentration of defect i.e. oxygen vacancies is found to decrease and the thickness of the films is found to increase with the increase in the deposition rate as revealed by RBS spectral analysis of the films. Films are found to be optically transparent with a small variation in the optical band gap. The variation is correlated with the defects and microstructure using the skin depth calculations. Electrical resistivity, carrier concentration and mobility in the films are found to change with the deposition rate, which is also correlated with the defect density and microstructure of the films. Furthermore, to understand the variation in the electrical properties and also the transport mechanism, the free electron gas theory is used considering the thin film as a degenerate semiconductor. It is concluded that at higher deposition power the structural and morphological properties of the film were found to be better. On the other hand the better electrical and optical properties were obtained at a lower deposition rate.
Acknowledgements
One of the author (N. Kumar) gratefully acknowledges the support from IUAC, New Delhi.