1.
Introduction
The researches of semiconductor materials and device physics[1–9] have largely supported the fast development of information society. Each technology therein depends crucially on the availability of specific suitable semiconductors. Silicon, an elemental semiconductor capable to be made ultra pure in large size and to be bipolarly doped controllably, sustains the required extreme low failure rate of each single unit in the integrated circuits[8]. III–V zinc blende semiconductors that can be continuously alloyed to achieve accurately designed band gaps of both well and barrier layers in the optoelectronic devices, are the crucial materials for semiconductor lasers, light-emitting diodes, photon detectors, etc.[9]. These elemental/binary diamond-like semiconductors and their alloys are the dominant materials used in information industry.
However, the number of diamond-type/zinc blende semiconductors is rather limited, as well as their diversity of materials properties such as band gaps, effective masses, and optical absorption. Although thermodynamically unstable semiconductor alloys offer the tunable properties, in many cases, suitable thermo-dynamically stable semiconductor compounds would be preferred if they are available. It was noticed in 1958[10] that series of new semiconductors could be derived from the elemental/binary diamond-like semiconductors by replacing one type of elements by pairs of elements from other groups in the periodic table while keeping the valence electron per atom unchanged. In 1960, the concept of super-cell structure of semiconductors was proposed[11]. The zinc blende structure can be treated as a super-cell structure of diamond, followed by further derivations of the zinc blende to chalcopyrite (CuFeS2-type) structure, etc. The super-cell structure could also contain ordered vacancies if the new species has excess ionic charge and needs a zero ionic charge species (vacancy) to compensate. These classes of new inorganic semiconductors as well as other classes we will discuss below provide a wide range of materials properties. Going from elemental and binary semiconductors to ternary or higher multinary materials, the number of possible compounds grows quickly.
Although the multinary diamond-like semiconductors are much more plenty than the elemental/binary semiconductors, they are much less used in electronic devices, especially for microelectronics; however, they are potential semiconducting electronic materials that are rigid in terms of the framework but are flexible in terms of internal electronic properties. The rigidity is needed to maintain the basic function of the electronic circuits. The flexibility is needed to realize passible self-learning electronic systems. In this sense, we will discuss the plenty of multinary semiconductors derived from existing materials using different design approaches.
2.
Iso-formula elemental substitution
The iso-formula elemental substitution approach was used in the pioneer discoveries of III–V semiconductors, e.g. from GaAs[12] to GaN[13], exploring all the ~28 members[9]. The development of new informatics technologies, such as artificial intelligence, which relies crucially on the available applicable semiconductors for a large number of rather different situations, would need a high diversity of the families of semiconductor compounds. The current compilations of previously documented inorganic compounds reveal that there are many multinary compounds (see Fig. 1 for a few popular multinary crystal structures) that have semiconducting band gaps[14, 15]. These compounds may not have the suitable semiconducting properties (e.g. low effective masses, dopability, etc.) for electronic devices, whereas the other members in the same families could have. Thus it is interesting to study the physical properties of the compounds in these types of materials families.

class="figure_img" id="Figure1"/>
Download
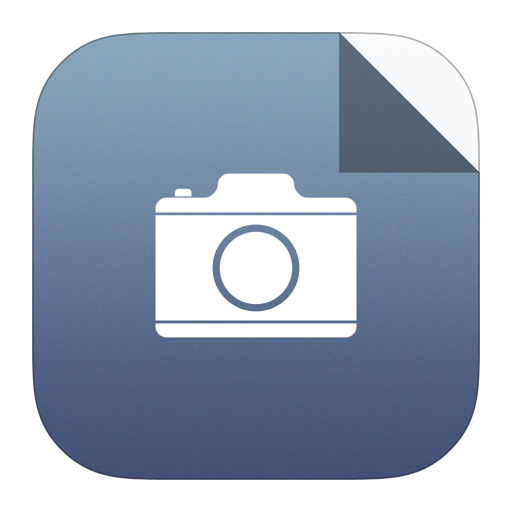
Larger image
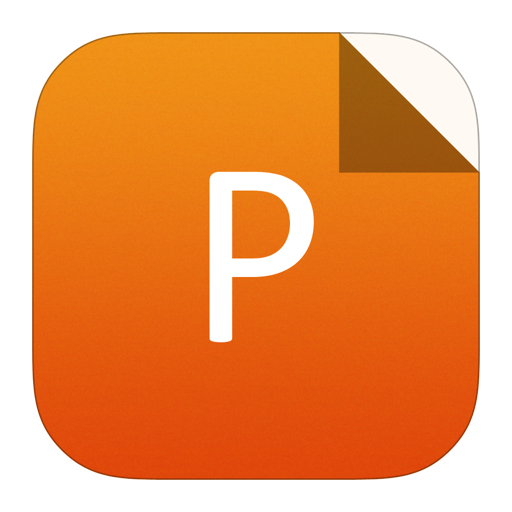
PowerPoint slide
Figure1.
(Color online) Crystal structures of typical ternary semiconductors: (a) ABX Half-Heusler structure (space group: F-43m), (b) ABX2 chalcopyrite structure (I-42d), (c) A2BX4 spinel structure (Fd-3m), (d) ABX3 perovskite structure (Pm-3m).
The cubic ternary ABX structure (LiAlSi-type, F-43 m, Half-Heusler) with the same space group as zinc blende compounds has attracted many attentions[16–21]. A few hundreds of ABX compounds with 8 valence electrons per formula unit in the cubic structure were studied theoretically, proposing many candidate optoelectronic materials including NaMgN, NaCaN, NaCuS etc.[16]. In the meantime, many cubic ABX materials were found to be topological semimetals[17–20] analogous to HgTe, including ScPtBi (with 18 valence electrons per formula unit) that is not synthesized yet. A recent fast screening of thousands of hypothetical cubic ABX structures with arbitrary numbers of valence electrons, for the design of semiconductors with low thermal conductivity, resulted in a small pool of cubic structures with either 8 or 18 valence electrons[21], reflecting the electron counting rule for semiconductors in this special crystal structure mimicking s2p6 (8) or s2p6d10 (18) electronic shells. A2BX4 spinel structure (Al2MgO4-type, space group Fd-3m) is another cubic structure that is closely related to zinc blende structure, in the sense that it contains tetrahedral local environments BX4. Several spinel compounds with high ion mobility were predicted[22] including Sc2ZnSe4 that is not experimentally reported yet.
The above application of simple iso-formula elemental substitution could diverge from the original design principles (i.e. the elemental substitution should not change the electronic structure or chemical bonding significantly). The proposed promising compound (e.g. NaCaN or Sc2ZnSe4) could be rather different than the prototype compound (e.g. LiAlSi or Al2MgO4) and thus could adopt another crystal structure or even become thermodynamically unstable. Therefore, it is necessary to first predict the crystal structure of the target compound and then evaluate its stability relative to its competing phases[23–25].
2.1
Prediction of crystal structures of unknown semiconducting compounds
Prediction of the crystal structure of an unknown compound without prior knowledge is typically based on optimization of the thermodynamic potential in the space of atomic coordinates, using algorithms such as simulated annealing[26], genetic algorithm[27–29], random search[30], and particle-swarm optimization[31], which are capable of going beyond the kinetic barriers around a local minima. These coordination-based optimization methods are successful for a number of focused cases of structure prediction[27–31]. However, for high-throughput predictions involving more than a handful of candidate materials, these methods without the help of prior knowledge are too computationally costly.
An efficient approach using prior knowledge is adopted in high-throughput predictions of the crystal structures of unknown compounds[32, 33], as described below. One can define a tensor for the experimental information on the known crystal structures of given compositions (xi) and sets of elemental constituents (ej), S(xi, ej). If for a given composition, e.g. ABX, and a given set of elemental constituents, e.g. NaMgN, the compound has not been reported yet, thus has no structure information, then S(xi, ej) = “null”. A probability density P(S(xi, ej)) can be defined based on the tensor S, which can be used to suggest the most likely crystal structures of unknown compounds. This approach can be illustrated by the diagrammatic separation of different crystal structures of a given family of compounds, such as A2BX4[34]. In Ref. [34], a diagrammatic separation of the 44 different crystal structures of 688 A2BX4 compounds was described by plotting the atomic orbital radii[35] of A versus B elements for many A2BX4 materials with various structures and seeking heuristically simple, straight boundaries that best separate the domains of different structure types in the structure field maps[35]. A success rate of 98% was achieved in such diagrammatic separation of structure types[34]. The structure field maps offer a quick guess of the crystal structure of an unknown compound by placing atomic orbital-radii coordination’s on such maps and reading off its most likely structure type.
The structure field diagrammatic separation of known A2BX4 compounds was then tested by using the theoretical data of the new A2BX4 compounds predicted from exhausting first-principles calculations[24] without using the structure field maps. The orbital-radii coordination’s of the new compounds were put on the A2BX4 structure field maps to suggest a structure type and to check whether the suggested structure type agrees with that from exhausting first-principles calculations. Unfortunately, the success rate decreases from 98% for the original diagrammatic separation[34] to 88% for the newly added data[24] (see e.g. Fig. 2). The exhausting first-principles calculations in Ref. [24] are also based on prior knowledge, but instead of relying on structure probability density, an expanded iso-formula substitution approach was applied, in which a large number of iso-formula substitutions were performed from the prototype compound of each structure type to the target compound, making sure that as much as possible prior knowledge on crystal structures are utilized. Another step conducted in Ref. [24] for predicting new compounds is the evaluation of the stability of unknown compounds relative to their competing phases, which will be described in details below.
2.2
Prediction ?of ?thermodynamic ?stability ?of ?unknown semiconducting?compounds

class="figure_img" id="Figure2"/>
Download
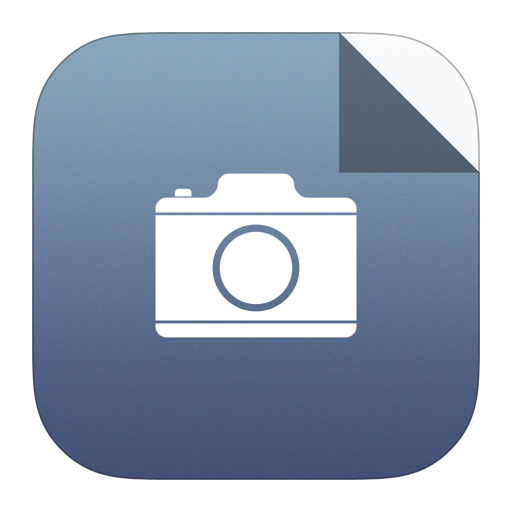
Larger image
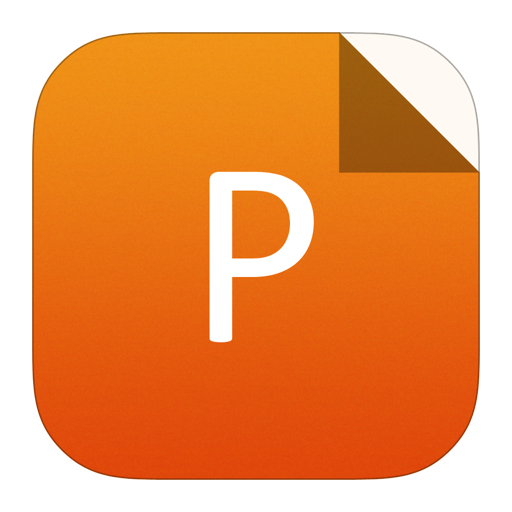
PowerPoint slide
Figure2.
(Color online) Structure field map of (a) A2BS4 and (b) A2BSe4 based on orbital radii. (Figure adapted from Ref. [24].)
Once the crystal structure of an unknown compound is determined, it is straightforward to calculate its total energy and compare to the total energies of its competing phases, which is equivalent to comparing their formation enthalpies by the analysis in the space of elemental chemical potentials (see e.g. Fig. 3)[24]. However, the current approximations such as generalized gradient approximation (GGA)[36] to density functional theory (DFT)[37] suffer from errors when predicting compounds’ formation enthalpies. To tackle this problem, a set of fitted elemental-phase reference energies (FERE) (see Fig. 4)[38] were obtained using an extensive set of 252 binary compounds with measured heat of formations, showing that after the fitting, the 252 formation enthalpies can be reproduced with the mean absolute error of 0.054 eV/atom instead of 0.250 eV/atom before fitting.

class="figure_img" id="Figure4"/>
Download
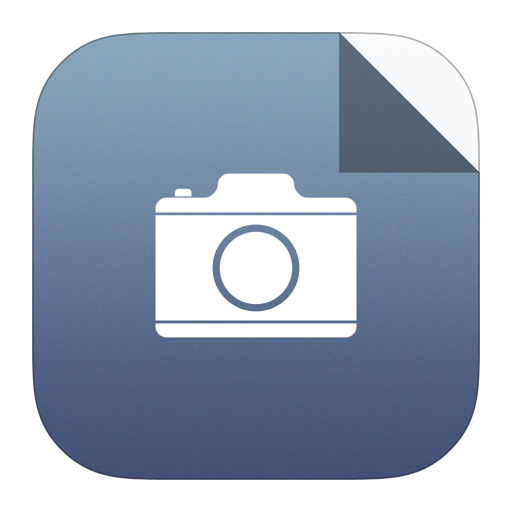
Larger image
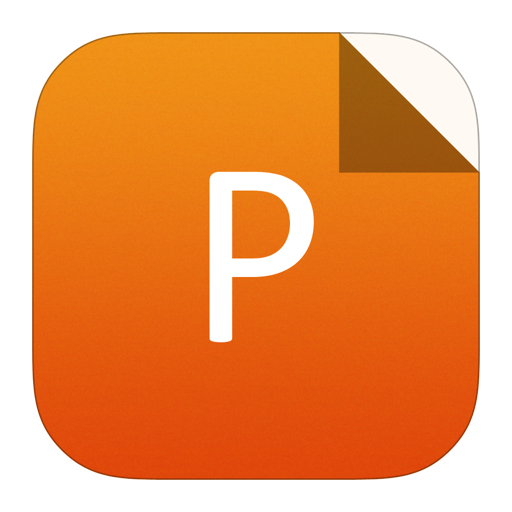
PowerPoint slide
Figure4.
(Color online) Part of the periodic table listing the FERE energies (in eV) for 50 chemical elements obtained by fitting using a set of 252 binary compounds with measured heat of formations. Colors denote the values of the Hubbard U parameter used in the calculations: U = 3 (light blue), 5 (orange), and 0 eV (light grey) (From Ref. [38]).
An unknown semiconductor could then be predicted by (i) predicting its crystal structure using expanded iso-formula substitution approach followed by (ii) testing its thermodynamic stability w.r.t. its competing phases using FERE energies. A few hundreds of new A2BX4 (see e.g. Fig. 5) and ABX (see e.g. Fig. 6) compounds were predicted recently[23–25] followed by experimental verifications[25, 39–42]. For many new compounds such as oxides, their growth conditions can also be predicted from the thermodynamic stability calculations. Fig. 7 shows the general relationship between growth temperature, oxygen partial pressure, and the predicted oxygen chemical potential (see e.g. the green area in Fig. 3).

class="figure_img" id="Figure5"/>
Download
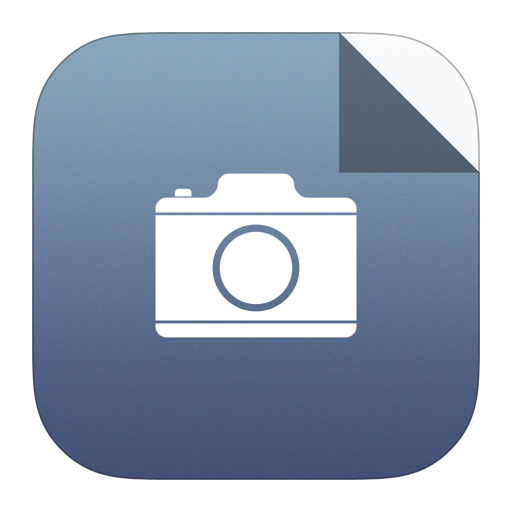
Larger image
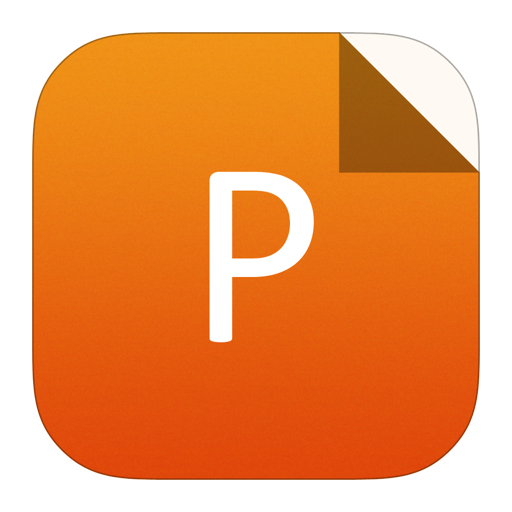
PowerPoint slide
Figure5.
(Color online) Reported (check-marks) and unreported (UR) A2BX4 (X = O and S) compounds. (Figure adapted from Ref. [24].)

class="figure_img" id="Figure6"/>
Download
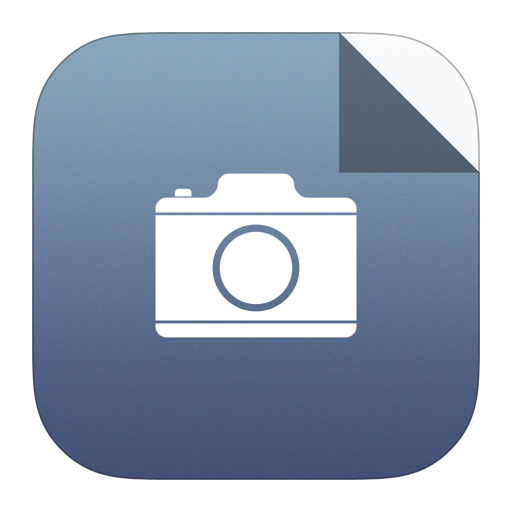
Larger image
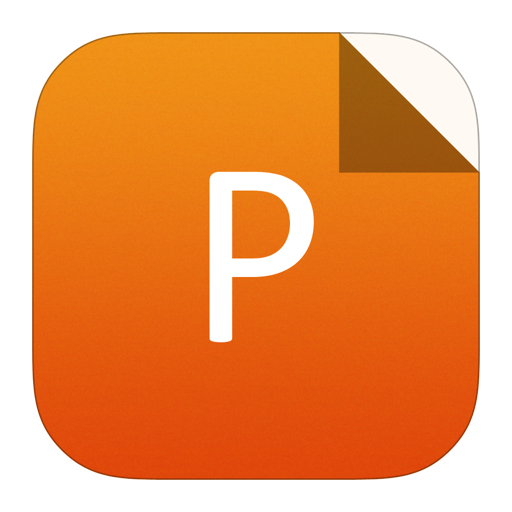
PowerPoint slide
Figure6.
(Color online) Four of the existing chemical groups of the ABX ternary materials containing 72 possible compounds, of which 40 are previously synthesized known compounds (denoted by check marks). Of the 32 unreported, 21 are now predicted stable (denoted by plus sign), and 11 are predicted unstable (denoted by minus sign). Of the 21 newly predicted compounds, 11 were recently attempted and successfully synthesized in the predicted structures[25] (shown in red circles). The ternary compounds with cubic space group F-43m are nonmetals (shaded green), whereas the noncubic compounds are metal (shaded blue) (From Ref. [43]).
Interesting semiconducting properties were found in these new materials[23–25, 39–43]. For the unknown compound ScPtBi that was predicted to be a topological semimetal in Ref. [18], Ref. [25] confirmed that it is indeed thermodynamically stable with the Half-Heusler structure. Furthermore, two new topological semimetals (HfIrAs and HfIrBi) were predicted in Ref. [25]. It is interesting to note that many previously predicted promising optoelectronic materials in Ref. [16] are found to be thermodynamically unstable (e.g. NaMgN, NaCaN) or stable in non-cubic structures (e.g. NaCuS) in Ref. [23]. Similarly, Sc2ZnSe4 that was predicted to be a spinel compound with high ion mobility in Ref. [22], was found to be unstable in Ref. [24]. Thus, although the simple iso-formula elemental substitution is convenient to apply broadly to many nominal compounds, it can lead to deviation from the prototype compounds, which is remedied by the expanded iso-formula substitution[23–25] using as much as possible prior knowledge on crystal structures and thermodynamic stability. Later on, we will focus on the co-substitution and related approaches for the design of semiconductors that are more likely to result in rationally designed semiconductors.

class="figure_img" id="Figure3"/>
Download
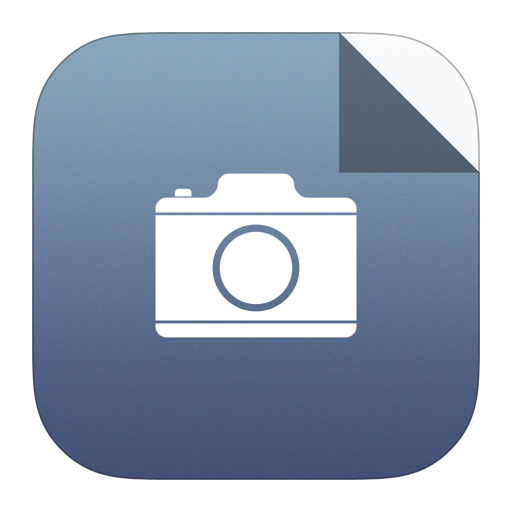
Larger image
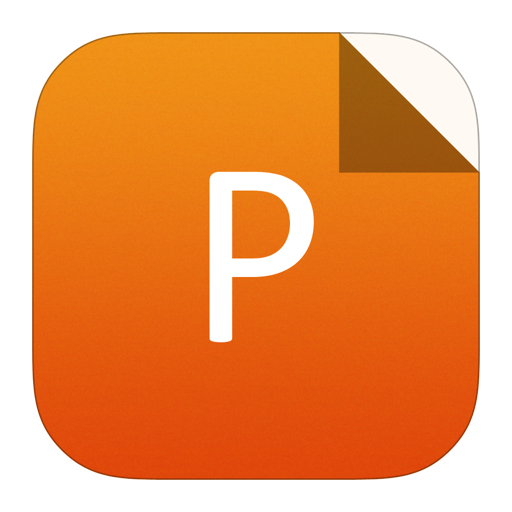
PowerPoint slide
Figure3.
(Color online) Thermodynamic stability analysis of Ni2ZnO4 and Co2BaO4 with green area showing the stability region. The blue/dark gray (red/gray) line is the cutting edge of the competing binary (ternary) phase, which cuts off a part of the triangle on one side of the line (Figure adapted from Ref. [24]).

class="figure_img" id="Figure7"/>
Download
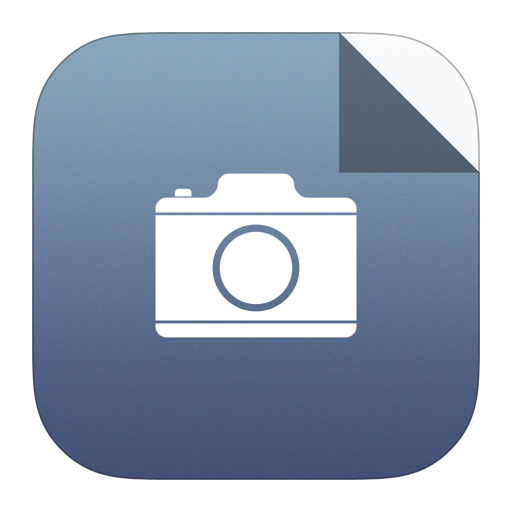
Larger image
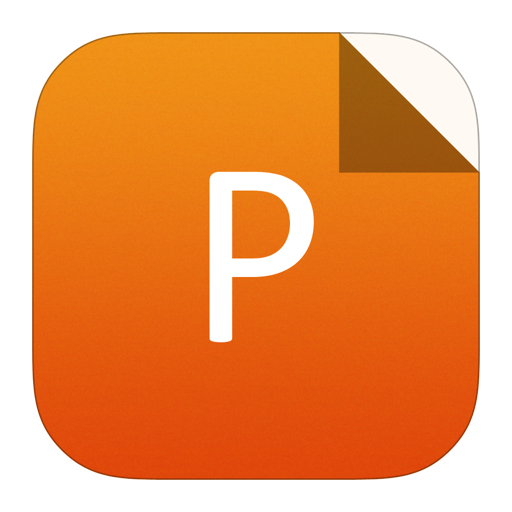
PowerPoint slide
Figure7.
(Color online) The oxygen partial pressure vs. temperature diagram for a range of values of oxygen chemical potential (From Ref. [24]).
3.
Co-substitution
The concept of co-substitution for designing new semiconductors was first proposed by Goodman[10]. Indeed, various series, especially the III–V series of semiconducting compounds that are synthetic compounds made in lab, were derived from known compounds via co-substitution of one element by pairs of elements, one with higher valence (or oxidation state) and the other with lower valence, i.e. a valence (or ionic-charge) disproportionation (e.g. Cd2+ to Cu1+ and In3+). The reason that co-substitution stabilizes a compound is analogous to the co-doping physics.
Co-doping was first introduced in 1963[44] and is now widely used in doping of wide-gap semiconductors for reducing band gaps[45] or overcoming the doping bottlenecks[46]. The energy efficient co-doping would be the mixing of a very shallow donor with donor level close to CBM and a very shallow acceptor with acceptor level close to VBM. The electrons in the donor level would then drops to the acceptor level, which releases a certain amount of energy. Furthermore, the electrostatic attraction between oppositely charged donor and acceptor along with the atomic relaxations upon donor-acceptor pairing releases another amount of energy. If the sum of these amounts of energies is close to the sum of the formation energies of the individual donor and acceptor, then the donor-acceptor defect complex will be easy to form[45].
From the view of defect physics, the stability of co-substitution that is a “100%” doping becomes clearer, if the sum of the energies cost for the formation of the two individual substitutions is smaller than the sum of energies saved from (i) the chemical transfer energy from higher energy levels to lower energy levels of the substituents, (ii) the Coulomb interaction, and (iii) the atomic relaxation when forming “donor-acceptor” pairs, then the co-substitution would be energetically favorable. Note that only from the defect physics point of view, it becomes clear that the two substituting elements act as oppositely charged species (“donor” and “acceptor”). Another energy-favorable feature of co-substitution compounds is that, once “100%” co-doping happens and the defects become ordered, an ordering energy would be gained due to lowered strain energy, Coulomb energy, etc.
Co-substitution is currently widely used in experimental and theoretical studies, leading to the finding of many new stable semiconducting functional materials. Experimentally, co-substitution of Zn and Sn in In2O3 was applied to design high performance transparent conductive oxides[47]; the Be-free deep-ultraviolet nonlinear optical materials were achieved via substitution of Be2+ by Li1+ and Al3+[48]; co-substitution of Pb2+ by Ag1+ and Bi3+ is found to be an effective approach to achieve Pb-free halide perovskite semicon- ductors[49]; chemical unit co-substitution was applied in the design of high photoluminescence phosphors[50, 51], etc.. Theoretically, the co-substitution chemical rule was used in the design of new I2–II–IV–VI4 semiconductors (see e.g. Fig. 8)[52] as well as a new group of I–III–Ge2N4 semiconductors[53] that are lattice-matched to GaN; high-throughput calculations were performed for the search of new Pb-free halide perovskites[54] based on the cation co-substitution rule.

class="figure_img" id="Figure8"/>
Download
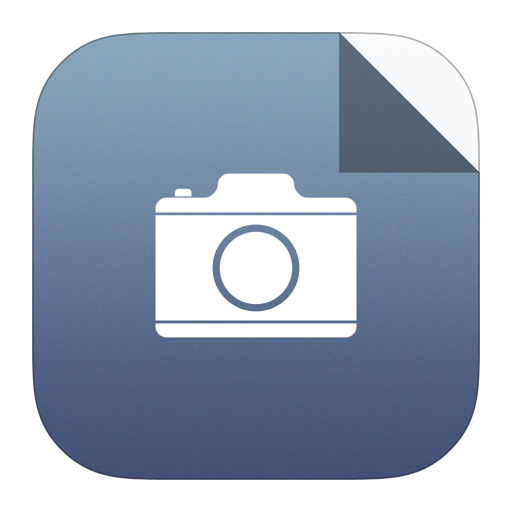
Larger image
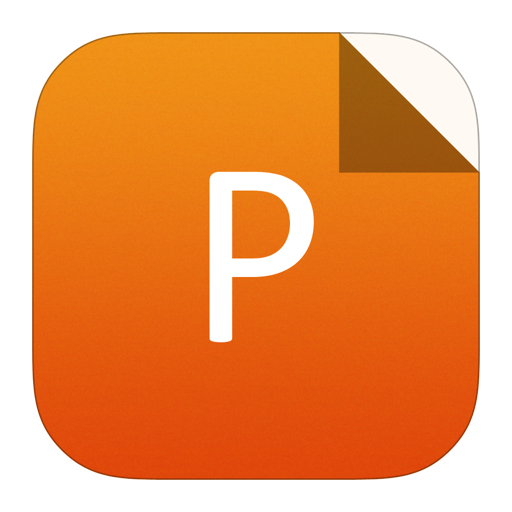
PowerPoint slide
Figure8.
(Color online) Derivation of I2–II–IV–VI4 and I–III–VI2 semiconductors from binary II–VI parent compounds[52].
The appearance of ordering and the ordered pattern of the co-substituted compounds with ionic-charge disproportionation could be related to the Madelung (electrostatic, Coulomb) energy of the lattice system. For co-substituted perovskite systems, the commonly found ordered pattern of substituent cations[49, 54] has the ions with high oxidation state (e.g. Bi3+) being the most separated. However, it is found that in certain cases, the ordering in co-substituted compounds is rather weak and order-disorder transitions could be driven by temperature variation or intended doping[55]. There could be significant variations of physical properties along with the order-disorder transitions, e.g. as shown in Ref. [55], from order to disorder phases, the band gap of Cs2AgBiBr6 perovskite changes from 1.93 to 0.44 eV, a range covering most of the band gaps of III–V semiconductors. Furthermore, along with significant change of ordering, the perovskite frame of the Cs2AgBiBr6 compound doesn’t change much[55]. The flexibility (e.g. tunable band gaps) of this type of semiconductors has potential applications in electronic devices. More flexibility in semiconductors could be achieved if they contain ordered vacancies or interstitials.
The elemental substitution along with the formation of ordered vacancies in diamond-like structures was discussed in 1960[11], which could be viewed as a generalized co-substitution, in which one of the substituents has zero oxidation state and is represented by a vacancy, forming ordered vacancy compounds. Analogous to co-substitution, the stability of ordered vacancy compounds could be understood from defect physics[56, 57].
The compounds derived from diamond-like structures by elemental substitution along with the formation of ordered interstitials were first discovered by Nowotny et al.[58] and Juza et al.[59]. The interesting electronic properties of this class of materials, called Half-Heuslers or filled tetrahedral structures, were revealed decades later[60, 61]. The way to derive this class of materials from diamond-like compounds could be viewed as another generalized type of co-substitution, in which one of the substituents is represented by an interstitial.
The ordered defects (vacancies or interstitials) that determine the electronic behavior of the above two classes of semiconductors could be tuned by external fields if they are mobile, to achieve flexible electronic properties in the rigid framework of diamond-like lattice; once the external fields are set to zero, the defects would redistribute into the original ordered patterns due to the ordering energy (see e.g. Refs. [56, 57]) gained by forming ordered phases. However, not all ordered-defect semiconductors have mobile-enough defects and rigid-enough frameworks. High-throughput first-principles calculations as discussed in Section 2 are needed for the prediction of new semiconductors with optimal properties.
4.
Summary
We reviewed the rational design approaches of multinary inorganic semiconductors, which encompass iso-formula elemental substitution, co-substitution, substitution along with ordered vacancy, and substitution along with filled interstitial. In these approaches, the basic structure motifs of parent semiconductors are maintained. Many recent theoretical predictions of new semiconductors and their physical properties have been verified by experiments, such as for TaIrGe[39], RbCuTe[41], TiIrSb, ZrIrSb, and HfIrSb[25]. The multinary semiconductors especially those with ordered defects are potential electronic materials with rigid framework and flexible/tunable electronic properties.