1.
Introduction
Printed and flexible electronics are definitely promising cutting-edge electronic technologies of the future due to their low cost, solution processability and large-area production compared with traditional silicon-based electronics. They can offer a wide variety of applications such as organic light emitting diodes (OLEDs)[1, 2], photovoltaics (PVs)[3–6], photodetectors (PDs)[7–10], and thin film transistors (TFTs)[11–14] in our daily life (as shown in Fig. 1[14–17]). Electrodes with good conductivity (surface resistance in several ohms to hundreds of ohms) and high transmittance (90% or more) in the visible light range, are extremely significant components of these optoelectronic devices[18]. Indium tin oxide (ITO) is the industrial transparent electrode in liquid crystal displays. However, with the popularity of flexible display and other flexible devices, the disadvantages of ITO conductive glass are exposed[19, 20]. Due to its brittleness, it has to be sandwiched in additional protective layers made of glass. It not only increases the difficulties of the production process, but also limits its applications in flexible devices. The lack of indium in nature adds to its difficulties in low-cost applications. In order to solve these problems, other promising conductive materials are found to be potential alternatives (listed in Table 1) such as carbon nanotubes (CNTs), silver nanowires (Ag NWs), silver nanoparticles (Ag NPs), copper nanowires (Cu NWs), copper nanoparticles (Cu NPs), graphene and conductive polymers[21].
Type | Composition | |||
Inorganic | Metal based | Metal NPs/NWs[22–24] | ||
Metal oxide[25–27] | ||||
Carbonaceous | CNT[28, 29] | |||
Graphene[30, 31] | ||||
Organic | Conductive polymer | Poly(3,4-ethylenedioxythiophene) (PEDOT)[32, 33] | ||
Polypyrrole (PPy)[34, 35] | ||||
Polyethylene naphthalate (PEN)[36, 37] |
Table1.
Classification of conductive materials as alternatives of ITO.
Table options
-->
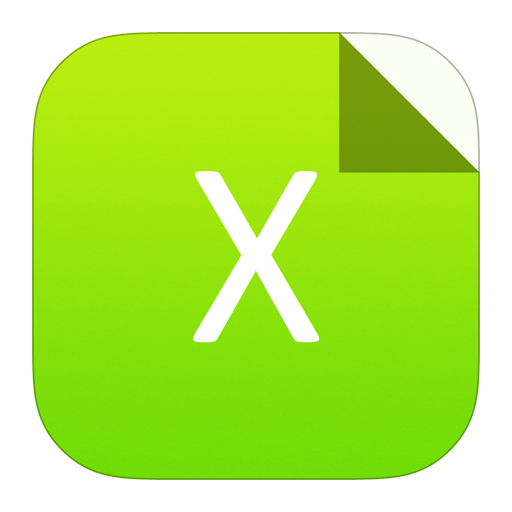
Download as CSV
Type | Composition | |||
Inorganic | Metal based | Metal NPs/NWs[22–24] | ||
Metal oxide[25–27] | ||||
Carbonaceous | CNT[28, 29] | |||
Graphene[30, 31] | ||||
Organic | Conductive polymer | Poly(3,4-ethylenedioxythiophene) (PEDOT)[32, 33] | ||
Polypyrrole (PPy)[34, 35] | ||||
Polyethylene naphthalate (PEN)[36, 37] |

class="figure_img" id="Figure1"/>
Download
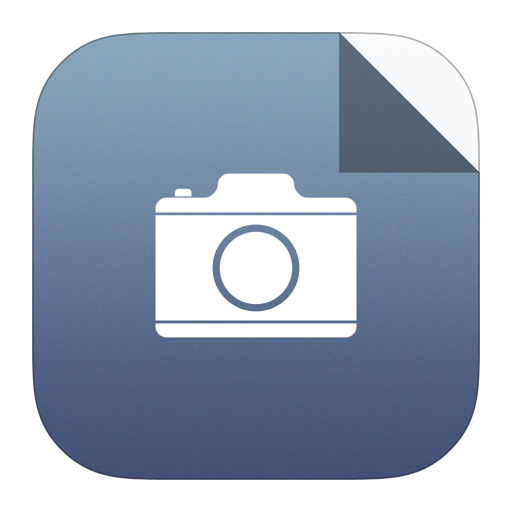
Larger image
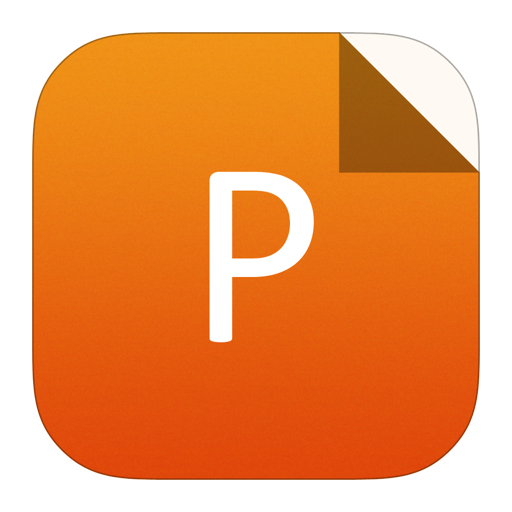
PowerPoint slide
Figure1.
(Color online) (a) The display’s circuitry is powered by the paper photovoltaic. Reproduced from Ref. [15]. (b) A flexible array of printed TFT. Reproduced from Ref. [14]. (c) Optoelectronic sensor that uses green and red polymer light LED and a silicon PD as the light detector. Reproduced from Ref. [16]. (d) A top emission OLED with CNTs as the transparent electrode. Reproduced from Ref. [17].
Printed electronics are based on printable conductive inks including organic and inorganic materials. These conductive materials can be dispersed in solvents and then made into designed patterns utilizing various printing technologies such as spin coating, inkjet printing, screen printing, and gravure printing (as illustrated in Fig. 2).

class="figure_img" id="Figure2"/>
Download
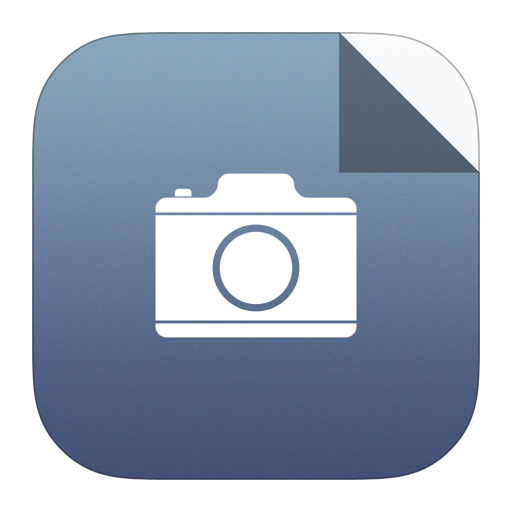
Larger image
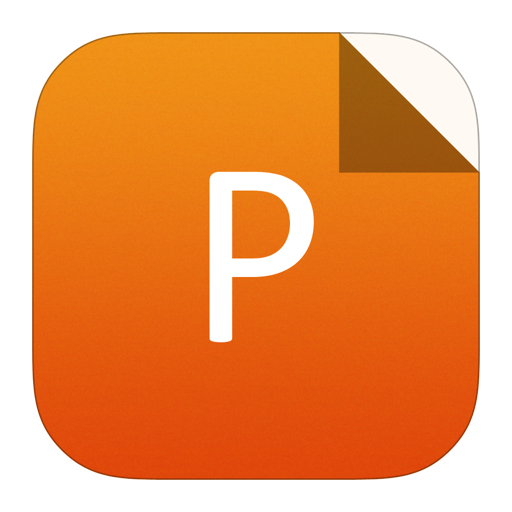
PowerPoint slide
Figure2.
(Color online) Different technologies for the fabrication of printed electrodes: (a) spin coating, (b) spray coating, (c) dip coating, (d) inkjet printing, (e) screen printing, and (f) roll-to-roll.
2.
Conductive ink
Conductive polymers are easily made into ink because of their excellent solution processability. Although the printed electrodes of conductive polymers are lightweight and flexible, the poor conductivity limits its large-area applica tions[33]. Compared with conductive polymer, inorganic materials are less flexible, but have much higher carrier mobility and conductivity[18]. In recent years, inorganic nanomaterials, such as nano-silver, nano-copper, graphene and CNT, have been successfully used in printed electronics. It was reported that inorganic materials became soft in low-dimensional structures[38, 39]. Printed devices made from inorganic nanomaterials have great potential in flexible electronics with higher performance compared with their counterparts made from organic materials. In this review, we will introduce the synthesis of printable inorganic nanomaterials for transparent electrodes.
Generally, the ink preparation methods include the direct addition of nanomaterial powders into suitable solvents and utilization of chemical reactions. Two key aspects related to the ink are the viscosity of the ink and its compatibility with substrate surface. Different printing methods have different requirements of the ink viscosity. For example, inkjet printing requires that the ink viscosity must be in the range of 10–20 cP (1 cP = 1 mPa·s) while gravure printing requires ink viscosity of hundreds of cP, and screen printing requires ink viscosity above 10000 cP[21]. In order to obtain high quality printed electronic devices, the ink and substrate surface need to match well with each other. If the substrate surface is highly hydrophilic, the ink combines well with the substrate and the obtained film is of good quality. If the substrate surface is hydrophobic, the lateral diffusion of the ink is much less, which leads to higher resolution.
3.
Printable nanomaterials
3.1
Metal nanomaterials
Compared with conductive polymer, the electrical and optical properties of metal nanomaterials are equivalent to that of ITO, sometimes even better than it[22, 23]. Metal nanomaterials in the forms of NPs (e.g. Cu NPs[40], Ag NPs[41, 42], and Au NPs[43]) and NWs (e.g. Ag NWs[44, 45], Cu NWs[23, 46], Au NWs[47, 48], and Pt NWs[49]) were intensively investigated. Thus the scalable synthesis of printable metal nanomaterials can contribute a lot to the development of printed transparent electrodes. In this section, we will summarize the synthesis process and applications of metal nanomaterials.
3.1.1
Ag NPs
Ag NPs used to be the common nanomaterials in conductive ink since Ag possesses the highest electrical conductivity and is stable in ambient conditions[18].
Ag NPs can be prepared by physical methods and chemical methods. Evaporation/condensation is a frequently used physical method[50]. It can be carried out using a tube furnace at atmospheric pressure. The source material within a boat centered at the furnace was vaporized into a carrier gas and then condensed to nanoparticles[51]. Chemical reduction is one of the most frequently applied methods for preparation of Ag NPs. In the process, silver ions in solution were reduced in conditions favoring the subsequent formation of small metal clusters or aggregates which finally led to the formation of Ag NPs[52]. The commonly used reductants are borohydride, citrate, ascorbate, and elemental hydrogen[50]. Then stabilizers were added to the Ag NPs solution to prevent aggregation caused by Brownian motion. Metal carboxylate, ammonium salts, and polymer stabilizers are frequently used stabilizers[53]. The stabilized Ag NPs inks can be printed into electrodes with different patterns. After proper sintering process[54], electrodes can be applied to various kinds of devices.
Li et al.[41] synthesized stable Ag NPs with a particle size of < 10 nm via a simple process. These NPs were stabilized with easily detachable alkylmines, thus permitting their ready conversion to highly conductive silver elements at low temperatures. Organic TFTs with the printed silver source/drain electrodes of this nature exhibited TFT properties similar to those using vacuum-deposited silver electrodes. Wu et al.[42] demonstrated that addition of a carboxylic acid to the silver salt solution improved the continuity of the resulting silver film, leading to significantly increased conductivity. The obtained silver film worked far more efficiently as electrodes in TFTs.
Zhang et al.[55] synthesized monodisperse Ag NPs with a new reduction system consisting of adipoyl hydrazide and dextrose at ambient temperature. By this simple and rapid approach, high-concentration, monodispersed Ag NPs were obtained at low protectant/AgNO3 ratio which was highly beneficial to low cost and high conductivity. Based on the synthesized Ag NPs, conductive inks were prepared with water, ethanol, and ethylene glycol (EG) as solvents. A radio frequency identification antenna was fabricated by ink-jet printing[55]. Flexible electrocircuits produced by ink-jet printing would have enormous potential for low cost electrodes and sensor devices.
Ag NP inks are already commercially available (at least 10 companies in the United States, Japan, Korea, and Israel produce Ag-based conductive inks)[56].
Although Ag NPs film is less transparent, Ag NPs ink has been widely used to produce metal mesh or metal grid type of transparent conductive electrodes.
Wang et al.[57] fabricated flexible transparent electrodes with embedded Ag mesh using roll-to-roll ultraviolet-nanoimprint lithography. The optical and electrical performance of the Ag mesh electrodes were 82.0% in transmittance and 22.1 Ω/sq in sheet resistance and the performances could be further improved by optimization of the mesh structures.
Zhang et al.[58] demonstrated a new approach to fabricated transparent electrodes based on Ag grid by ink-jet printing. The Ag grids with average length of 5–6 μm could be sintered at room temperature by taking the advantage of coffee ring effect. Their performance was comparable to ITO, yielding a transmittance higher than 93.6% and a sheet resistance of less than 30 Ω/sq.
Hong et al.[59] fabricated a Ag grid transparent conductor using selective lasing sintering of Ag NPs ink. The Ag grid with high transmittance (> 85%) and low sheet resistance (30 Ω/sq) could be produced on polymer substrates on a large scale by rod coating process or roll-to-roll and selective lasing sintering process.
Transparent flexible electrodes made of Ag grid and mesh have been applied in touch screen panels and OLEDs[59, 60], suggesting its great potential as alternatives of ITO.
3.1.2
Cu NPs
The synthesis of Cu NPs also attracted great interest due to its huge potential for replacing the Ag NPs. Many methods have been explored to synthesize Cu NPs, such as wet chemistry and gas/solid phase methods[18, 24, 61]. The wet chemical method is very convenient by just adding reducing agent into the precursor to reduce the metal ions in liquid media. Thus, the particle characteristics can be well controlled by tuning the experimental parameters like temperature, pH, concentration of reagents and so on[62]. However, the inherent tendency to oxide in air of copper make it much more difficult to fabricate Cu electrode and films, because the oxidation leads to higher sintering temperature and lower conductivity[24]. Therefore, it is critical to synthesize air-stable Cu NPs.
There are numerous reports stated below which demonstrated the synthesis of Cu NPs to retard oxidation. Some research studies retard oxidization by performing reaction in organic surroundings under inert atmosphere[63]. The most common method is to form a protective layer surrounding the Cu NPs to minimize the exposure to the air. The protective layers can be classified into surfactants, polymers, and carbon-based materials. Polymers are the most effective of the above protective layers, because the polymeric layer is dense enough to effectively retard the oxidation rate[18]. Polyvinyl pyrrolidone (PVP) is the most widely used polymer both in organic solvents and in aqueous medium, and can form a dense layer on the surface of the Cu NPs[64].
Jeong et al.[65] used PVP as protective layers and succeed in controlling the thickness of the surface oxide layer on Cu NPs by changing the molecular weight of PVP added to the reaction mixture. In addition, their results confirm that the thickness of the protective layer is the predominant factor determining the electrical conductivity of granular film obtained by annealing NPs assembles. Lee et al.[64] used the same polymer, PVP 40, to synthesize conductive copper ink, and formed conductive patterns with inkjet printing. After a low temperature sintering process (200 °C for 60 min in H2), the obtained copper patterns had low electrical resistivity.
Another method to retard Cu NPs from oxidization is the use of antioxidants[66, 67]. It was reported that ascorbic acid was good reducing agent that could retard oxidization rate[68].
In reality, protective layer and antioxidants just retard the oxidation process rather than prevent it. Needless to say, it is still hard to synthesize Cu nanostructures with long-term stability utilizing above methods. Thus the core-shell model was proposed[69]. The copper core was coated with dense shell layers composed with nonoxidizable conductive materials. Firstly, 3 nm layer of graphene was formed as the shell through reducing flame technique. The obtained Cu NPs were not oxidized up to 165 °C. However, the conductivity of the obtained Cu NPs was five orders of magnitude lower than of bulk Cu[70]. In order to obtain high conductivity, bimetallic core-shell structure, the protective layer of which are made of noble metals, was proposed[71]. The shell is usually made of noble metals mainly through two methods. One is based on the use of a reducing agent, immobilized on the surface of core pre-particles[72]. The other one, called transmetalation, is based on galvanic displacement reaction which just occurs on the surface of Cu NPs[63]. It can retain the morphologies of NPs and avoid the self-nucleation of the shell layer. These advantages made the transmetalation widely used in synthesizing bimetallic core-shell structures.
Grouchko et al.[72] synthesized nonoxidizable Cu NPs based on a transmetalation reaction on the surface of NPs. This process resulted in formation of Cu@Ag core-shell NPs, with no individual Ag particles. The 2 nm shell of Ag prevents oxidation of the copper core and preserves its metallic characteristic. Concentrated aqueous dispersions of these core-shell nanoparticles were printed on various substrates. The patterns were stable to oxidation up to 150 °C. Tsuji et al.[73] prepared Cu@Ag core-shell NPs using a two-step polyol reduction method under N2 gas. As-prepared NPs had an average diameter of about 80 nm. The oxidation of Cu NPs was suppressed greatly by Ag shell.
Jang et al.[40] fabricated Cu film by inkjet printing. The film was crack free after sintering at 250 °C under reducing atmosphere. The patterns were electrically tested by light-emitting diodes (LED) and showed good conductivity and flexibility. Wu et al.[74] fabricated Cu films on low-Tg substrates by screen printing. High conductivities of 28 and 44 μΩ·cm were obtained after low-energy photonic sintering. Simple circuits and radio frequency identifications were also fabricated, demonstrating the feasibility of Cu NPs for practical applications.
Though Cu NPs were applied in some flexible electrodes, the low transmittance of Cu NPs films restricts its applications in flexible transparent electrodes. Thus much more research on Cu nanomaterials transformed to synthesis, optimization, and applications of Cu NWs in recent years.
3.1.3
Ag NWs
As an important branch of Ag nanomaterials, Ag NWs have become good choices to produce flexible transparent conductive electrodes because of their excellent flexibility, high transmittance, and good conductivity. In recent years, Ag NWs have become the most promising alternative for ITO, and draw much attention, especially in the industry of liquid crystal displays[75–77].
3.1.3.1
Preparation of Ag NWs
The preparation of Ag NWs can be divided into physical methods and chemical methods. Physical methods include magnetron sputtering, ultrasonic pulverization, and mechanical pulverization[78–80]. Chemical methods consist of hydrothermal synthesis[81], photochemical reduction[82], electrochemical method[83], template method[84], ultrasonic reduction[85], and polyol reduction[86, 87]. The physical method is much more complex with large energy consumption, high technical requirements, but offering poor uniformity of morphology, which severely restricts its utilizations. Compared with physical methods, chemical methods are much simpler in process, much lower in cost, and much diversified and uniform in morphology. Among those chemical methods, the polyol reduction method has the advantages of higher efficiency than other methods and was widely used.
The polyol reduction method. It was easy to obtain Ag NWs by introducing Pt NPs as seeds into the reaction system in which AgNO3 was used as precursor, EG as solvent and reducing agent, and PVP as surfactant[65, 88–90]. Other kinds of surfactants and crystal seeds were also investigated[91–93].
Sun et al.[94] prepared the various kinds of Ag NWs with uniform morphologies and high aspect ratio. Pt was firstly used as crystal seed at 160 °C to prepare the Ag NWs with diameter of 30–40 nm and length of up to 50 μm. Ag NWs with the diameter of about 60 nm without addition of extraneous seeds were prepared subsequently. Moreover, iron ions and chloride ions were also used as control agents to explore their impacts on the morphology[95]. Salt-assisted polyol reduction method has been popularly studied and improved to obtain high quality Ag NWs. Experimental parameters have been studied and improved[79].
Effects of experimental parameters on the morphology of Ag NWs. On the basis of the traditional polyol reduction method, the influence of various experimental parameters on the morphology of Ag NWs was studied in detail. Polyol species, molecular weight and concentration of surfactants, salt type and concentration, concentration of silver nitrate, and temperature are all included. In most of the polyol reduction reactions, EG was used as solvent. Interestingly, Shobin et al.[96, 97] used glycerol instead of EG as solvent to obtain Ag NWs with diameter of about 60 nm and length of about 7 μm. Bergin et al.[79] investigated the effect of temperature on the morphology of Ag NWs and found that Ag NWs became finer and shorter with the temperature increasing and became thicker and longer at lower temperature. Trace salts acted as control agents and played key roles in the preparation of Ag NWs. A variety of different salts such as FeCl3[98], CuCl2[99], NaCl[100], and Na2S[91], were used to assist the preparation of Ag NWs. Zhang et al.[44] used silver as a control agent to prepare Ag NWs with a diameter of 100–300 nm. Johan et al.[45] used CuCl2 and NaCl as control agents to explore their effect on the morphology of Ag NWs, respectively. Chen et al.[101] used KBr, KOH, KCl, Fe(NO3)3, and Na2S as control agents to prepare Ag NWs with diameters of 20–500 nm. Chenet al.[102] studied the effects of nucleation conditions and protective atmosphere on morphology and found that nitrogen tended to improve the reproducibility. Then Chen et al.[103] used Na2S as a control agent and found that lower concentration of Na2S led to formation of silver nanocubes, and Ag NWs could be obtained with high concentration of Na2S. The concentration of AgNO3 was directly related to the preparation efficiency and scales. Ma et al.[104] studied the impact of AgNO3 concentration on morphology by utilizing FeCl3 as control agent, and high purity of Ag NWs were obtained when the concentration AgNO3 was in the range of 0.1–0.6 M. Jiu et al.[74] analyzed the effect of agitation speed on morphology. They synthesized Ag NWs with length up to 60 μm and found that the Ag NWs tended to be longer as the agitation speed decreased. Coskun et al.[105] studied the effect of temperature, molar ratio of PVP to AgNO3, NaCl concentration, and agitation rate on the morphology. They demonstrated that the diameter of the Ag NWs gradually decreased with the increase of the temperature, the molar ratio of PVP to AgNO3 and the stirring speed, and no Ag NWs were formed in too low or too high concentration of NaCl and PVP. Li et al.[106] also studied the effect of different molecular weights of PVP to the synthesis of Ag NWs. Three different PVP (molecular weight: 50 k, 360 k, and 1300 k) were used in their experiments. The morphology of Ag nanostructures had a strong relationship with the molecular weight of PVP as shown in Figs. 3(a)–3(f)[106]. By adopting 360 k PVP, longer and thinner Ag NWs were achieved. The growth mechanism of Ag NWs using PVP with different molecular weight is also illustrated in Figs. 3(g)–3(i)[106]. The appropriate suppression of growth along (100) direction by adopting 360 k PVP facilitated the formation of thin and high Ag NWs. Lin et al.[107] explored the effects of temperature, the rate of silver nitrate addition, and the molecular weight and concentration of PVP on the diameter and length of Ag NWs. Ag NWs with a diameter of about 170 nm and length of 20 μm were obtained. It was found in the report that the diameter of Ag NWs became thicker and the length-diameter ratio decreased with the increase of PVP concentration.

class="figure_img" id="Figure3"/>
Download
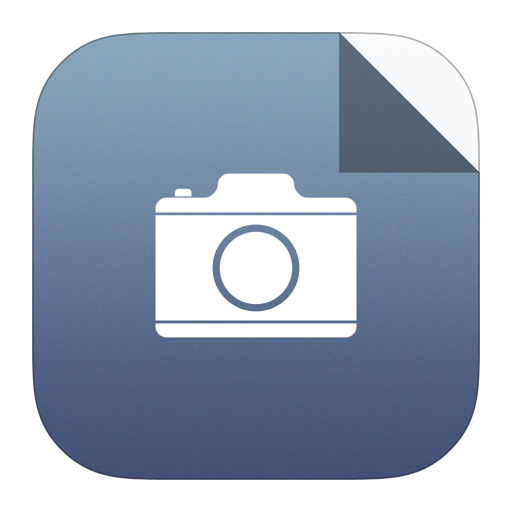
Larger image
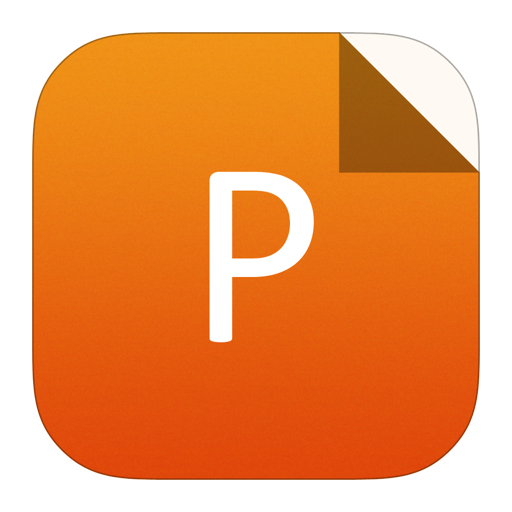
PowerPoint slide
Figure3.
(Color online) (a)–(f) Morphologies of Ag NWs. (a) and (b) show Ag NWs synthesized with 50 k PVP. (c) and (d) show Ag NWs synthesized with 360 k PVP. (e) and (f) show Ag NWs synthesized with 1300 k PVP. (g)–(i) show the growth mechanism of Ag NWs using 50, 360, and 1300 k Mw PVP, respectively. Suppression forces of different kinds of PVP are shown. Reproduced from Ref. [106].
It is worth noting that most previous research concentrated more on transparency, but overlooked the haze factor compared with ITO. Optical haze value is ratio of diffuse transmission and total transmission and high optical haze will significantly restrict its applications such as in display devices. To reduce the haze and to increase the flexibility of the printed devices, it is essential to synthesize ultra-thin Ag NWs with extremely high aspect ratio[108–110]. Lee et al.[109] developed a novel successive multistep growth method to synthesize ultra-long Ag NWs with length of 400–500 μm and aspect ratio of 1000–3000 which was an order of magnitude length enhancement from previous research. It was demonstrated that Ag NWs continued to grow through successive multistep growth as long as Ag ion rich conditions were maintained continuously. For successive multistep growth, the first grown Ag NWs were used as seed NWs to grow very long NWs by repeating the modified growth process.
3.1.3.2
Application of Ag NWs
In addition to the inherent excellent mechanical and electrical properties of nanowires, Ag NWs have high thermal conductivity and high transmittance, leading to a wide range of applications in conductive glue, touch screen panels, sensors, nano-heaters[111, 112], and other electronic devices.
Lee et al.[111] reported a highly stretchable and transparent supercapacitor based on electrochemically stable Ag@Au core–shell nanowire percolation network electrode. The resultant supercapacitor showed stable performance up to 60% tensile strain, and succeeded in operating a commercial LED. The working state of obtained supercapacitor is illustrated in Fig. 4(a).
Moon et al.[110] fabricated electrodes composed of synthesized thin Ag NWs via Mayor rod coating method without any annealing process. The fabricated electrodes maintain superior properties (~96%, 100 Ω/sq, 2% haze). A flexible and highly transparent touchscreen with an optimized Ag NWs percolation network was then fabricated. The panel composed of thinner Ag NWs had lower haze factor than those made of thick Ag NWs. Hong et al.[114] also fabricated a capacitive touch sensor using Ag NWs network through selective laser ablation. Munsik et al.[115] utilized high-quality, solution-processed Ag NWs films with an excellent optical transmittance of 96.5% at 450 nm and a low sheet resistance of 11.7 Ω/sq as transparent conductive electrodes in inorganic III-nitride LEDs. LEDs fabricated with Ag NWs electrodes exhibited excellent performance, showing a 56% reduction in series resistance, 56.5% brighter output power, a 67.5% reduction in efficiency droop, and approximately 30% longer current spreading length compared with LEDs fabricated with reference electrodes consisting of a 14 nm-thick oxidized Ni/Au layer. The utilization of Ag NWs remarkably enhanced the devices performance and reduced the cost, which suggested that the Ag NWs were promising for application as next-generation transparent electrodes to produce brighter, larger-area, cost-competitive inorganic III-nitride LEDs. King et al.[116] fabricated a highly sensitive, flexible and stable multidimensional strain sensor by using two layers of Ag NWs percolation network (as illustrated in Fig. 4(b)[113]). The obtained strain sensor shows large gauge factor (~20) and large stretchability (35%) suggesting its potential to detect any kind of natural strain with high sensitivity. Hong et al.[111] fabricated a highlystretchable and transparent Ag NWs heater (as illustrated in Fig. 4(c)[111]) for the first time based on partially embedded Ag NW percolation network on polydimethylsiloxane film. The obtained heater could operate well under strain over 60% and other real-time deformations commonly associated with human motion such as bending and twisting.
As stated above, Ag NWs play a key role in producing electrodes on flexible substrates. Additionally, the film preparation is relatively simple and can be formed by solution process or spray to make transparent electrodes[98]. High transparency, low surface resistance, smooth surface, and better chemical, thermal and mechanical stability are requirements for its applications in optoelectronics devices. Therefore, how to synthesize thinner, longer Ag NWs and to optimize the film fabrication process are extremely important for the high-performance electrodes[80].
3.1.4
Cu NWs
Compared to noble metal, copper has much lower cost (100 times less expensive than Ag and ITO) without much decrease (only 6%) in conductivity and has diminished electron migration effect[18]. So it is an extremely promising alternative of nano silver ink in printed electronics[117].
3.1.4.1
Preparation of Cu NWs
Many methods have been explored to synthesize Cu NWs, such as chemical vapor deposition (CVD), vacuum thermal decomposition, and electro-spinning[75]. In the past ten years, the solution process has been preferred due to its easy combination with large area production[18]. Wet chemical methods, including hydrothermal synthesis, reduction of a precursor solution, and catalytic synthesis have become popular routes to NW synthesis. In these methods reducing agents were commonly used to reduce metal ions into metal atoms and capping agents were used to transform metal atoms into the NWs[23]. The morphology can be well controlled by tuning the experimental parameters like temperature, pH, concentration of reagents and so on[117].
Li et al.[46] developed a novel and simple hydrothermal method to synthesize massive Cu NWs with different aspect ratios by using a commercial electric pressure cooker. Oleylamine (OLA) and oleic acid were used as dual surfactants to enhance the dispersion stability of Cu NWs and glucose was used as the reducing agent. Then transparent and conductive Cu NW networks with excellent transmittance and sheet resistance were fabricated by a spin-coating process. Zhang et al.[118] utilized catalytic synthesis method to prepare Cu NWs in liquid crystalline medium of binary hexadecylamine and cetyltrimethylammonium bromide surfactants. The obtained NWs were well-dispersed and ultralong (tens of micrometers to several millimeters). Similarly, Cu NWs with a high aspect ratio (diameter of 16.2 ± 2 nm and lengths up to 40 μm) were synthesized using a nickel (II) acetyl acetone (Ni(ac)2) catalyst in an OLA solution[19]. However, catalytic methods and hydrothermal methods suffer from a long reaction time (several hours to several days) and high temperature (120–180 °C). Lower reaction temperature and shorter reaction time are required in reduction methods with which shorter Cu NWs could be obtained[119].
In addition, the inherent tendency of copper to oxidize in air makes it much more difficult to fabricate copper electrodes, because the oxidation leads to lower conductivity[24]. Therefore, it is critical to synthesize air stable Cu NWs. Thus the core-shell model was proposed[120]. The copper core was coated with dense shell layers composed of non-oxidizable conductive materials. In order to obtain high conductivity, bimetallic core-shell structure in which the protective layer was made of noble metals, was proposed. The shell was mainly synthesized through two methods. The first was based on the use of a reducing agent, immobilized on the surface of core pre-particles[72]. The second was based on galvanic displacement reaction which just occurred on the surface of Cu nanomaterials[63]. It could retain the morphologies of NWs and avoid the self-nucleation of the shell layer.
Stewart et al.[121] demonstrated a room-temperature solution phase process to synthesize Cu@Ag and Cu@Pt core-shell NWs in which ascorbic acid removed the passivating copper oxide coating from the Cu NWs, and reduced noble metal ions onto the Cu NWs while preventing galvanic replacement. Cu@Ag NWs were highly conductive as printed and the obtained NW films exhibited equivalent optoelectronic properties to films of Ag NWs with a similar aspect ratio. Unlike Cu NWs, Cu@Ag NWs with core-shell structure were resistant to oxidation in dry air at 160 °C and under humid conditions (85%) at 85 °C for 24 h. Xue et al.[122] synthesized Cu@Ni alloying shell NWs via one-pot method. The NWs showed excellent properties such as crystalline alloyed shells, clear and abrupt interfaces, smooth surface, and length of more than 50 mm. They studied the effects of different nickel content on its transmittance, electrical properties, and oxidation-resistant performance. When the ratio of nickel and copper was about 4 : 1, the stability of the NW film could be enhanced to 900 times compared to film made from pure Cu NWs[18]. Additionally, other materials such as reduced graphene oxide (RGO)[123] or Al-doped ZnO (AZO)[120] were also used as cover layers of pre-made Cu NW films in recent reports. RGO and AZO can not only protect the underneath Cu NWs from oxidation, but also provide 2D pathways for charge transport between non-percolated NWs, resulting in high stability and high conductivity. Niu et al.[125] synthesized ultrathin Cu@Au core-shell NWs using trioctylphosphine as a strong binding ligand to prevent galvanic replacement reactions. The epitaxial overgrowth of Au shell with a few atomic layers on the surface of Cu NWs could greatly enhance their resistance to heat (80 °C), humidity (80%) and air for at least 700 h, while their optical and electrical performance remained similar to the original Cu NWs.
More recently, it was revealed that CuxO NWs could be reduced to Cu NWs via laser irradiation[126]. Those processes can be performed rapidly at room temperature without using any vacuum process and reagent. The resulting Cu NWs exhibit superior conductivity with better resistance to the environment due to the enhanced Cu NW junctions. As a result, CuxO NWs can be employed as starting materials for Cu NW thin films, thereby facilitating long-term storage and versatile applications of the material[126].
In short, the latest reports demonstrate various methods to synthesize air-stable Cu NWs. The proposed bimetallic core-shell structure successfully solved the problem, as prepared electrodes can be stable for at least several months of oxidation after inkjet printing and proper sintering pro- cess[19, 63]. Nevertheless, its conductivity still needs to be improved. Additionally, there are still challenges that need to be overcome, such as easier anti-oxidation process and large scalable fabrication, before Cu NWs can be applied to commercial devices. Further studies on solving these problems are required.
3.1.4.2
Applications of Cu NWs
As stated above, many efforts have been made to prepare Cu NWs and Cu NW-based transparent conductive films with excellent performance in terms of optoelectronic properties and mechanical flexibility. Many potential applications have also been demonstrated.
Song et al.[127] utilized Cu@Cu-Ni NWs with lower resistance of 62.4 Ω/sq at 80% transparency as electrodes in OLED devices. The performance of obtained OLED did not show evident degradation during 600 cycles of bending, stretching, and twisting tests as illustrated in Fig. 4(d).
Han et al.[128] demonstrated a touch screen panel based on flexible transparent Cu NW electrodes using ultrafast plasmonic nanoscale welding. As illustrated in Fig. 4(e), the panel could work well with low haze factor. Zhang et al.[129] developed a glucose sensor that could detect glucose with an excellent limit of detection, a wide linear range, and high sensitivity. This was mainly attributed to the large surface area, excellent conductivity, and efficient electron transfer of the as-prepared Cu NWs. Stewart et al.[130] fabricated an organic photovoltaic (OPV) using Cu@Ni NWs as anodes. They further investigated that the film-based electroless nickel-plating process that gives Cu NW films a stability comparable to Ag NW films, even under humid conditions. Finally, they demonstrated that bulk-heterojunction OPVs using films of Cu@Ni NWs as the transparent anode could achieve a device efficiency of 4.9%, nearly equivalent to the 5% efficiency of a bulk-heterojunction OPV utilizing Ag NWs as the transparent anode in their experiment[130].
3.1.5
Au NWs
The typical Ag NWs with diameters of 30–80 nm have the drawbacks of causing scattering or haze at low wire densities, while Cu NWs are normally not air-stable, so in some cases inert metal NWs such as Au or platinum (Pt) NWs are imported to solve these issues when low cost is not the first priority[108]. Various synthesis methods were developed to promote the utilization of Au NWs, including chemical reduction[131], electrodeposition[132], and slicing thin Au films[133]. However, most of the early reported techniques resulted in the production of polycrystalline or relatively large diameter (45 nm) Au NWs with low aspect ratios and rough surface[108].
Huo et al.[47] synthesized ultrathin single crystal Au NWs with diameter of ~1.6 nm and length of few micrometers with high yield by simply mixing HAuCl4 and oleylamine at room temperature. Unlike other 1D nanomaterials, which are straight with high persistence length, ultrathin Au NWs are serpentine at the nanoscale behaving like “polymer chains” because of their ultrathin nature. Chen et al.[48] further developed a stretchable and transparent nanomembrane using ultrathin Au NWs. The self-assembled thin film is mechanically flexible and electrically conductive, with an optical transmittance of 90%–97% over a wide spectral window of 3001–100 nm. Garcia et al.[134] reported novel types of transparent conductive materials based on ultrathin Au NWs with diameters below 2 nm and high aspect ratios by wet-processing. The Au NWs films combine high mechanical flexibility, optical transparency, and chemical inertness. They also investigated different annealing processes based on temperature and plasma treatment, to remove the ligands after deposition to improve electrical conductivity[134].
Au NW-based transparent electrodes have also been applied to different devices. Bao et al.[135] reported patterned Au NW networks with high conductivity and stability used as transparent electrodes in self-powered flexible CH3NH3PbI3 (MAPbI3) PDs (as illustrated in Fig. 4(f)[135]). The PDs had higher flexibility than ITO-based devices, and 60% of the initial photocurrent could be retained. Gong et al.[136] constructed a highly sensitive, flexible pressure sensor by sandwiching ultrathin Au NW-impregnated tissue paper between two thin polydimethylsiloxane sheets. The constructed pressure sensors could be operated at a battery voltage of 1.5 V with low energy consumption, high accuracy, and high stability (450000 loading–unloading cycles). Nevertheless, high cost is still the barrier for large-scale applications of Au NWs.

class="figure_img" id="Figure4"/>
Download
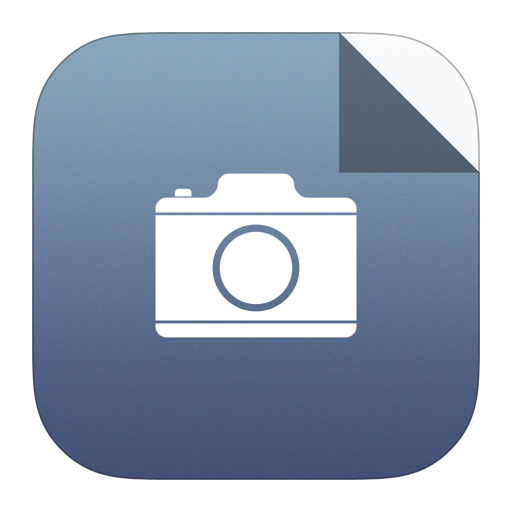
Larger image
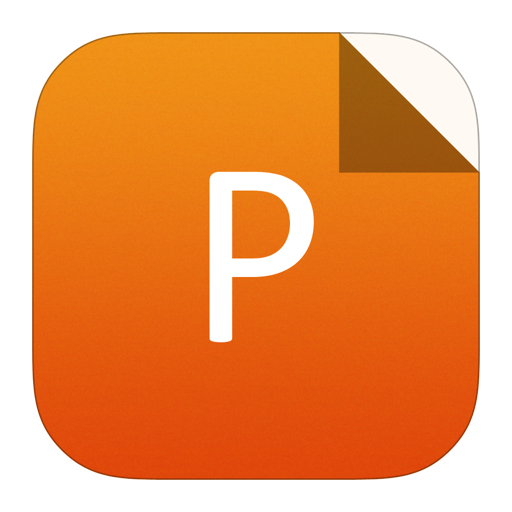
PowerPoint slide
Figure4.
(Color online) Various applications of metal NWs. (a) Photo of Ag@Au core–shell NW-based supercapacitor at 30% strain. A commercial LED could be turned on at the stretched conditions. Reproduced from Ref. [113]. (b) Photo of a multipixel strain sensor array (left) and it is embedded in a glove to provide handgrip motion (middle and right). Reproduced from Ref. [116]. (c) Photo of a stretchable and transparent heater affixed to a human wrist at outward bending (left), neutral (center), and inward bending (right) conditions. Reproduced from Ref. [111]. (d) OLED applications of Cu@Cu4Ni NW conductive elastomer composites. Reproduced from Ref. [127]. (e) Photo of the touch screen panel fabricated with Cu NWs transparent electrodes. Reproduced from Ref. [128]. (f) Photo of the flexible MAPbI3 PD based on PEN/Au NW transparent electrodes. Reproduced from Ref. [135].
3.2
Carbon nanomaterials
3.2.1
Graphene
Graphene has a 2D planar structure in which the carbon atoms are bonded to each other by an sp2 orbital to form a honeycomb hexagonal ring[137]. It has been extensively examined around the world since it was first successfully exfoliated from graphite by physicists in University of Manchester since 2004[137]. Graphene has high carrier mobility (up to 150 000 cm2?V?1?s?1 at room temperature), good transmittance (97.7%), and high tensile strength limit (42 N/m). The extremely excellent performances make it the promising conductive material in the future. Many methods were proposed to synthesize graphene and graphene ink for their wide applications.
3.2.1.1
Preparation of graphene
Preparation of high quality graphene is the basis for its applications. There are various methods, which can be divided into bottom-up method and top-down approaches according to the mechanism.
In bottom-up method, carbonaceous compounds are selected as a carbon source and thermally decomposed to form a small molecule to synthesize graphene[21]. CVD and segregation growth are all bottom up methods to synthesize large-area monolayer graphene[107]. CVD has been the commonly used method which can produce defect-free single crystal graphene since it was first used in 2009[138]. Plasma-enhanced CVD (PECVD) is able to grow single layer graphene under shorter reaction time and lower deposition temperature conditions than the CVD process[139]. However, the rates of growth on metal foil (such as Cu foil) are extremely low. Xu et al.[140] synthesized single-crystal graphene with growth rate of 60 μm/s (more than two orders of magnitude faster than previous reported rates) by placing the copper foil above an oxide substrate which can provide a continuous supply of oxygen to the surface of copper during the CVD growth. For ink preparation, the metal substrates must be removed through chemical etching and then resultant graphene films are transferred into solvents[141]. Although the approaches can produce single-crystal graphene with less defects, these methods are not widely used because of their complexity, limited scaling-up capability and the high cost of metal substrates[142].
In top-down method, graphite is the carbon source. External forces are used to overcome the van der Waals force between stacking layers to exfoliate graphene sheets[143, 144]. Micro-mechanical exfoliation, liquid-phase exfoliation (LPE), ultrasonication exfoliation, and solvent thermal exfoliation are the commonly used top-down method. LPE is the most widely used top-down method to obtain pure and high-quality graphene sheets in polar solvents such as N, N-dimethylformamide (DMF), N-methyl pyrrolidone (NMP), and terpilenol. However, the two-dimensional hydrophobic graphene sheets are extremely easy to aggregate in polar solvents. Thus, it is necessary to add a stabilizer to the solution to block aggregation[144]. The most commonly used stabilizers are polycyclic aromatic hydrocarbons, surfactants (sodium dodecyl sulfonate, sodium benzenesulfonate), and polymers (ethyl cellulose, PVP). Similar to that of CNT, hydrophobic ends of the surfactants adsorb on the surface of the graphene sheet while its hydrophilic ends span into the polar solvents[18]. However, the concentration of as-produced graphene ink is too low (up to 0.1 wt.%) to prepare conductive patterns by various printing technologies. Normally, tens of layers are printed to obtain dense and conductive patterns[18, 21].
Torrisi et al.[145] produced a graphene-based ink by LPE of graphite in NMP. Conductive and transparent patterns were produced, with ~80% transmittance and ~30 kΩ/sq sheet resistance. Hyun et al.[146] produced solvent exfoliated graphene by ultrasonication of graphite in a solution of ethyl cellulose (EC) in ethanol. This method can offer high yields as high as 0.2 mg/mL. In order to obtain high concentration of graphene solutions, they used a two-step centrifugation method. Thick and unexfoliated graphite was firstly removed and graphene sheets with the lateral size of about 70 × 70 nm2, thickness of ~3 nm were obtained in sediment in the second step. Then the as-produced graphene, mixed with EC powder, was dispersed in ethanol and terpilenol through bath sonication. The concentration of as-prepared conductive ink was about 80 mg/mL, much higher than a previous report using pristine graphene[147]. The high concentration graphene ink was screen-printed into patterns using high-resolution silicon stencil. After annealing at the 300 °C for 30 min, the conductivity of graphene patterns was (1.86 ± 0.19) × 104 S/m, highly competitive among solution-processed graphene. The bending test showed little change in resistivity during 1000 bending cycles. Secor et al.[143] dispersed the graphene/EC powder in a solvent system composed of 85 : 15 v/v cyclohexanone/terpilenol to prepare graphene ink. Then the ink was printed on different substrates by inkjet printing. What was appealing was that they used the intense pulsed light (IPL) to anneal printed patterns. IPL was compatible with flexible substrate like PET, PI, and PEN. The obtained patterns showed high conductivity of approximately 25000 S/m. IPL was also used in another report to anneal graphene and it set graphene as a more promising candidate in printed electrode on flexible substrates[148]. Casaluci et al.[149] also produced graphene ink by LPE of graphite in DMF. By using spray coating they deposited the ink on a fluorine-doped tin dioxide substrate to realize a large area (~90 cm2) counter-electrode with a transparency of 44%.
The most widely used method to prepare graphene ink is based on the reduction of GO from graphite utilizing strong oxidizing agents. Because of polar groups such as carboxyl groups and hydroxyl groups on the surface of the RGO sheet, it is not necessary to add stabilizer to avoid aggregation. At the same time, high concentration of solutions can be obtained, which is suitable for screen printing and gravure printing. Various reducing agents such as hydrazine, sodium borohydride, and lithium aluminium hydride were used[21]. He et al.[150] proposed a simple and eco-friendly solution approach to chemically reduce GO utilizing nontoxic inexpensive reductants to produce high-quality graphene ink. Kong et al.[151] fabricated graphene electrode onto flexible polymeric materials by inkjet printing. GO sheets were first dispersed in water and subsequently reduced into graphene utilizing an infrared heat lamp at a temperature of ~200 °C for about 10 min. The sheet resistance of the electrode could be as low as 0.3 MΩ/sq and its transmittance could be as high as 86% by tuning the spacing between adjacent ink droplets and the number of printing layers. The temperature sensitivity of the graphene electrode was also studied. The graphene electrode was found to have temperature response with negative temperature coefficient, but with longer response time compared with other temperature sensors[151].
Although reduction procedures of GO have been successfully applied, electrical and mechanical properties of obtained RGO are still much lower compared with that of pristine graphene due to the defects and low carbon content. Graphene inks with different functional groups were also studied. Ionic liquid, polyaniline, polyelectrolyte, and poly(sodium-styrenesulfonate) (PSS) anions were all used to modify graphene as shown in Figs. 5(a) and 5(b)[152]. The surface energy can be easily tuned by those functional groups attached to graphene. For example, surfactant (e.g. PSS anion)-modified graphene ink was very stable and had the best wettability (as shown in Figs. 5(c) and 5(d)) and thus very good adhesion to the substrate. Georgakilas et al.[153] proposed an effective procedure for dispersion of pristine graphene in water without any surfactants or polymers. Dihydroxyphenyl functionalized CNTs played the role of stabilizer in their research. The superstructure of graphene/CNT hybrid exhibited good dispersibility in water and polar solvents, as well as the low resistance up to Rs ≈ 25 Ω/sq.

class="figure_img" id="Figure5"/>
Download
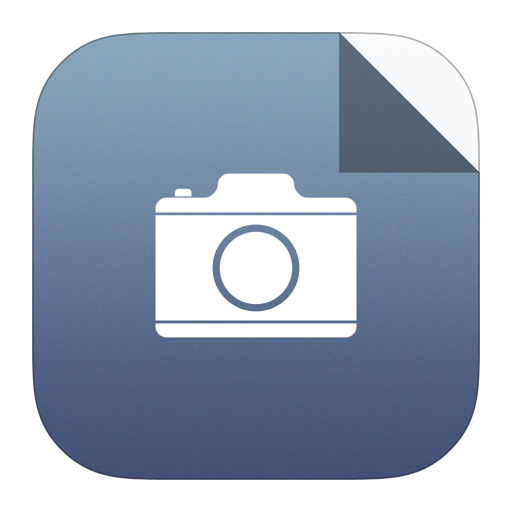
Larger image
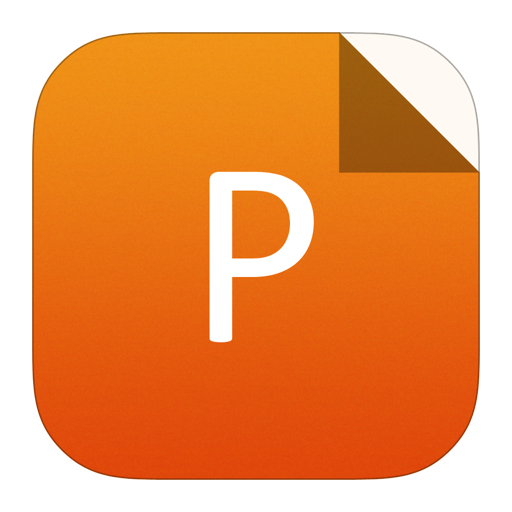
PowerPoint slide
Figure5.
(Color online) (a) Graphene functionalized by IL and (b) graphene modified by different functional groups of polyaniline, polyelectrolyte, and PSS anions. (c) and (d) Drops of graphene inks with different surface tensions on Si substrates after being drop-cast and when dried respectively. (1) IL-modified graphene (2) KOH-exfoliated graphene (3) polyelectrolyte-modified graphene, (4) PSS-modified graphene and (5) polyaniline-modified graphene. Reproduced from Ref. [152].
Most ink synthesis processes such as LPE or ultrasonication are not suitable for mass production because of the low throughput and complex procedure. At the same time, the conductivity of printed graphene patterns is still much lower than that of ITO. Thus much effort and research on graphene are still required.
3.2.1.2
Applications of graphene
The excellent electrical, optical, and mechanical properties have made graphene popular in optoelectronic applications especially in transparent electrodes[154].
Han et al.[155] fabricated a flexible OLED with polymer-modified graphene as anodes. Because of the high work function and low sheet resistance of anode, the OLED exhibited high luminous efficiencies (37.2 lm/W in fluorescent OLEDs, 102.7 lm/W in phosphorescent OLEDs), which were significantly higher than those optimized devices with ITO anodes (24.1 lm/W in fluorescent OLEDs, 85.6 lm/W in phosphorescent OLEDs). Fig. 6(a) illustrates the working OLEDs.
Yu et al.[156] fabricated a small hysteresis integrated circuit by introducing monolayer graphene for the electrodes. A transparent 16 × 9 integrated circuit array is illustrated in Fig. 6(b). This device with transparent and flexible properties showed excellent device performance with subthreshold voltage of 220 mV/decade, operation voltage of less than 5 V, on/off ratio of approximately 104, mobility of 81 cm2·V?1·s?1, and transparency of 83.8% including substrate. What is more, no significant transconductance changes were observed in 1000 times of bending test.
Lee et al.[157] demonstrated an all-graphene-based array on a stretchable rubber substrate as illustrated in Fig. 6(c). Graphene offered excellent mechanical, electrical, and optical properties, capable of use as source/drain electrodes as well as the semiconducting channels. The fabricated graphene transistors showed hole and electron mobilities of 1188 ± 136 and 422 ± 52 cm2·V?1·s?1, respectively. They could also operate stably at stretching up to 5% even after 1000 or more cycles.
Bae et al.[158] fabricated graphene electrodes with sheet resistances as low as ~125 Ω/sq with 97.4% optical transmittance by roll-to-roll technique. Then the electrodes were applied into a touch screen panel device as illustrated in Fig. 6(d). The obtained touch screen panel was capable of withstanding high strain.

class="figure_img" id="Figure6"/>
Download
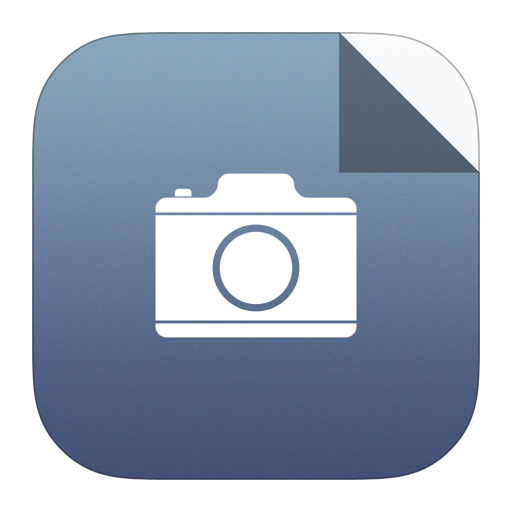
Larger image
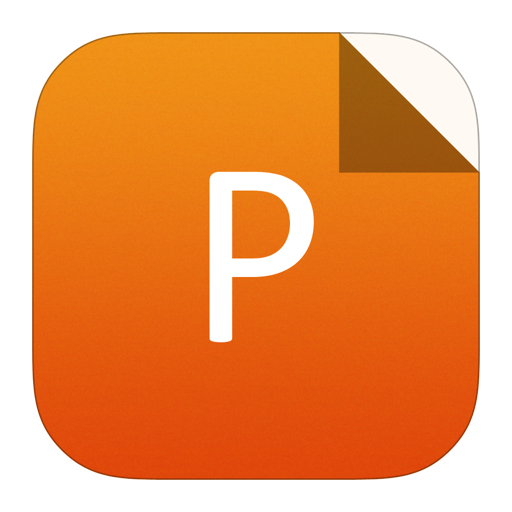
PowerPoint slide
Figure6.
(Color online) (a) Flexible OLED lighting device with a graphene anode on a 5 × 5 cm2 PET substrate. Reproduced from Ref. [155]. (b) Photo of the fabricated ?exible and transparent TFTs based on graphene on a PET substrate. Reproduced from Ref. [156]. (c) Arrays of transistors based on all graphene electrodes on balloon. Reproduced from Ref. [157]. (d) A graphene-based touch screen panel connected to a computer with control software. Reproduced from Ref. [158].
3.2.2
CNTs
CNTs are considered as the most straight, tough materials with hollow tubular structures with one-atom thick carbon wall. CNTs were successfully synthesized in the 1990s and classed into semiconducting type and metallic type according to chirality[159].
The basic transport properties of CNT were extensively studied. The resistivity of individual single-walled carbon nanotube (SWCNT) and multi-walled carbon nanotube (MWCNT) could be as low as 10?6 and 3 × 10?5 Ω·m, respectively[160]. They are expected to replace ITO transparent electrode because of their excellent electrical optical and mechanical properties.
3.2.2.1
Preparation of CNTs
CNTs can be produced by three major methods: (1) electric arc discharge (2) laser ablation (3) CVD[161, 162]. CVD has been the most widely used method to fabricate CNT because of its milder reaction condition, lower energy input, and good scalability compared with the other two methods[140]. In CVD growth, a substrate with catalyst nanoparticles was placed in a chamber with supply of carbon source and hydrogen source[162]. The most frequently used catalysts are Fe, Co, and Ni. CNTs were consequently grown directly on the surface of the substrate. Diameters of CNTs were mainly related to the diameters of catalyst nanoparticles. SWCNTs were usually synthesized using small catalysts with diameters of 0.5–5 μm while MWCNT can be obtained using bigger catalyst nanoparticles with diameters of 8–100 μm[163]. It was reported that additives (such as alkali metal salt[164] and chlorinated benzene[165]) could change the diameters of CNTs although same catalysts were used. Aligned SWCNTs and MWCNTs can also be synthesized via CVD combined with electric filed, laser ablations, or gas-flow. Alignment of CNTs can enhance of conductivity of as-produced electrodes[160].
One disadvantage of CVD-grown method is the presence of semi-metallic SWCNT which reduces the conductivity of as-produced electrodes[144]. Purification of SWCNTs are achieved according to chirality by density-gradient centrifugation in combination with selective surfactant wrapping[166] or by gel chromatography[167].
3.2.2.2
CNTs ink
It is required to disperse purified CNTs in proper solvents to prepare conductive ink[144, 160]. Due to the strong van der Waals forces between CNTs, it is difficult to disperse them evenly in solvents. Three methods were proposed to disperse CNTs.
In the first method, CNTs were directly dispersed in pure organic solvents[168, 169] or superacid[169]. Thus CNTs were not polluted and retained optoelectronic properties because there was no additive in the pure solvent[170]. However, the concentration of pure solvent dispersed CNTs ink is low, often unable to meet requirements of printing technologies (> 0.1 g/L). In the second method, surfactants such as polymer[171], polysaccharide[172], starch[173], urotropin[174], DNA[175], and cellulose derivatives[176] are added to the aqueous solution to disperse the CNTs well. Both electrostatic repulsion and steric effect can contribute to the dispersion and stabilization of CNTs in solution[176–178]. It was shown that surfactants with more charges enabled better dispersion of CNTs through the Zeta electric potential test[175]. The biggest problem is that surfactants on the surface of the CNTs seriously affect the conductivity, therefore a subsequent post-annealing step is required to remove the surfactant. The third method is the modification of the surfaces of CNTs[180–182]. CNTs can be well dispersed with the addition of functional groups on the surface[168, 183]. It was confirmed that this method could offer high concentration of single or small groups of CNTs. What is unsatisfactory is that the functional groups will destroy the sp2 bond of CNTs to some extent, which greatly reduce their conductivities[28]. In order to disperse the agglomerated CNTs into individual CNT, several methods are used, including high shear mixing, three stick grinding, ball milling and ultrasonication[18]. The most commonly used method is ultrasonication, which is particularly suitable for dispersing CNTs in aqueous solution. Pressure waves from the vibrated ultrasonic probe led to formation of instantaneous expanded and collapsed solvent bubbles, which separates the agglomerated CNTs. During this process, the surfactant has enough time to wrap the surface of CNTs to prevent the reagglomeration[184, 185].
3.2.2.3
Preparation of CNT films
CNTs films with high conductivity and transmittance are very critical to extend applications in conductive transparent electrodes. The typical film preparation process is illustrated in Fig. 7(a).
Although mobility of CNT films is still higher than that of ITO films, the carrier concentration of CNT films is usually as low as 1017 cm?3, which is three orders lower than that of ITO films[182]. Therefore, it is suitable to increase carrier concentration by doping in order to obtain high conductivity. NO2[187], Br2[188], HNO3[189], SOCl2[190] and other strong oxidizing chemicals were often used as p-type dopants to increase its conductivity. The conductivity of doped CNTs could be improved by 3–4 orders of magnitude[191]. Graphene was also reported as dopant[192].
When CNTs are utilized to prepare electrodes, the contact resistance of the network structures will greatly reduce the conductivity[193]. The individual CNT have a conductivity of up to 2 × 105 S/cm and a mobility of more than 105 cm2·V?1·s?1[194], while the undoped, randomly stacked CNT film has the conductivity as low as 6600 S/cm and the mobility is as low as 1–10 cm2·V?1·s?1[161, 195, 196]. It indicated that carriers have very poor transport properties between CNTs. Typically, individual CNT with length of 1 μm has a resistance of about 10 kΩ[197], and the contact resistance of CNTs is as high as 200 kΩ to 20 MΩ[198]. Wang et al.[199] dispersed SWCNTs in water and obtained high concentration of SWCNT ink with the help of surfactants. Conducting films were produced through a rod-coating method using the ink. The conductivity of SWCNT films were improved without post annealing but with nitric acid[199]. Nevertheless, the contact resistance of CNTs is very high, resulting in relatively poor photoelectric properties of CNT transparent electrodes. Therefore, the use of longer CNTs can effectively reduce the contact between CNTs, thereby reducing the resistance of electrodes and improving the device performance.
Increasing the ratio of metallic CNT to semiconducting CNT could also effectively reduce the contact resistance. Fuhrer et al.[193] studied all the contact types between the two CNTs. The experimental results showed that the current of metallic–metallic contacts at 100 mV was 123 times higher than that of metallic–semiconducting contacts, while the current of semi–semiconducting contact was 60 times of that of metallic–semiconducting contacts. This is due to the formation of Schottky barrier between the metallic and semiconducting CNTs[200, 201]. Therefore, obtaining high purity metallic CNTs is a prerequisite for the preparation of high performance CNTs electrodes.
The conductivity of CNTs films is also related to the bundle size of tubes, quantity of wall layers, and lattice integrity[184, 202]. The presence of amorphous carbon or sp3 carbon bonds reduced the resistance of CNT films. In general, the higher the G/D ratio measured by Raman spectroscopy, the higher conductivity could be obtained[203]. With smaller bundles, higher conductivity could be obtained[202]. This was because more tubes were not involved in conducting networks with greater bundles. The synthesis of long, high purity metallic, small bundle CNT will effectively improve the photoelectric properties of prepared conductive films.
In addition, composites can also be used to improve the photoelectric properties, such as composites of CNTs with Ag NWs[204], graphene[205], and conductive polymers[206]. It can greatly enhance the performance of CNT films as illustrated in Fig. 7(b).

class="figure_img" id="Figure7"/>
Download
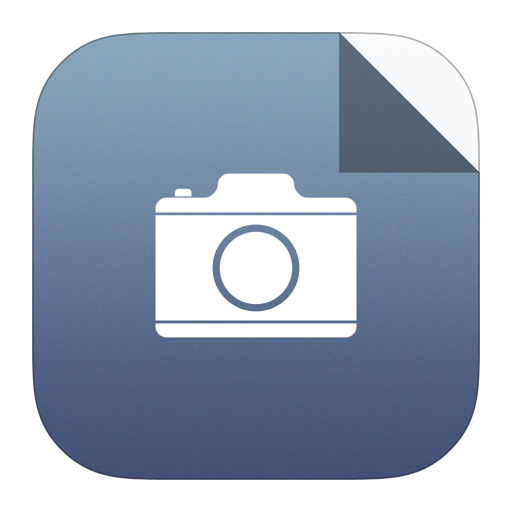
Larger image
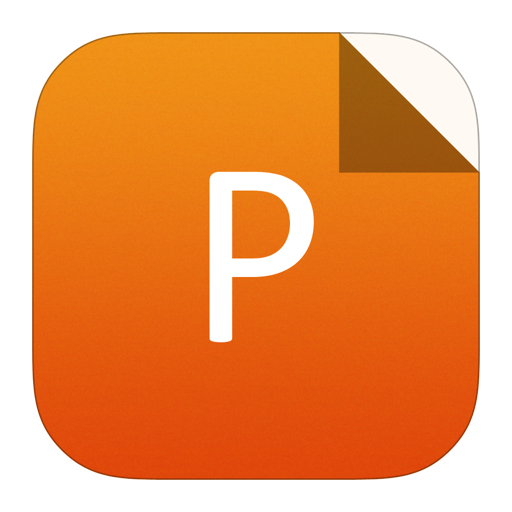
PowerPoint slide
Figure7.
(Color online) (a) Schematic of CNT dispersion, film deposition, and post treatment. (b) Enhancement of the conductivity utilizing different methods. Modification/doping will decrease the sheet resistance of CNT to 50–200 Ω/sq. Ultra-long CNTs may reduce the sheet resistance to 10–50 Ω/sq. Incorporating with metal NWs/NPs can further reduce the sheet resistance. Reproduced from Ref. [196].
3.2.2.4
Applications of CNTs
Numerous research studies have been reported on SWCNT and MWCNT conducting films and their commercial applications.
Rowell et al.[207] fabricated flexible transparent electrodes by printing films of SWCNTs networks. The electrodes exhibited a transmittance of 85% at 550 nm and sheet resistance of 200 Ω/sq. Then they fabricated a solar cell using SWCNTs film as anode as illustrated in Fig. 8(a). The solar cells with efficiencies of 2.5% showed great flexibility.
Hecht et al.[208] incorporated CNTs films as top electrode in a four-wire resistive touch screen panel as illustrated in Fig. 8(b). Sliding stylus-pen tests revealed no loss of device linearity after 1 million stylus cycles. Single-point actuation tests revealed the superior mechanical performance of CNTs electrodes compared with ITO touch electrodes, with no loss of device functionality up to 3 million actuation.
Cao et al.[209] demonstrated a TFT composed of CNTs as source/drain electrodes and semiconducting channel. As illustrated in Fig. 8(c), implanted with plastic substrate and flexible plastic substrates, these TFTs exhibited high degree of flexibility.
Hu et al.[210] utilized transparent and conductive SWCNT network as electrodes in OLEDs. The OLEDs are illustrated in Fig. 8(d). They compared the SWCNT networks with ITO and SWCNTs exhibited much more durable sheet conductance under bending process. The obtained OLEDs showed outstanding light output and life time. Gao et al.[28] also fabricated the SWCNT transparent conducting films for the electrodes of OLEDs. The as-prepared SWCNT film displayed a relatively high sheet resistance of 82.6 Ω/sq at transmittance of 80.7% with a relatively large surface roughness of 30 nm. PEDOT:PSS, carboxylic acid and SOCl2 were used to modify the films, and the modified film had a lower sheet resistance of 70.6 Ω/sq at transmittance of 81%, and lower surface roughness of 3 nm.
Schindler et al.[211] developed liquid-crystal display with random CNT networks as transparent electrodes. As illustrated in Fig. 8(e), the display can work well during bending.

class="figure_img" id="Figure8"/>
Download
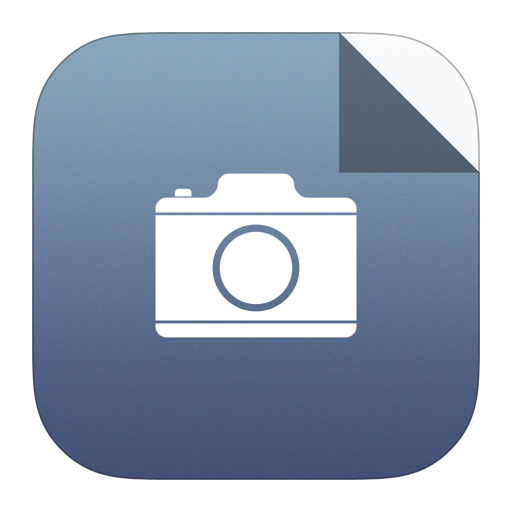
Larger image
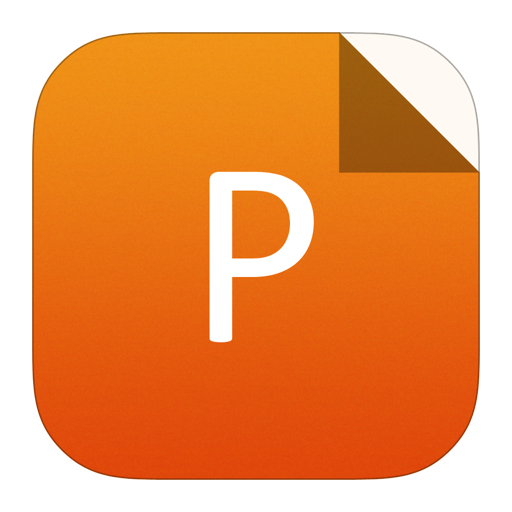
PowerPoint slide
Figure8.
(Color online) (a) Photo of the highly flexible cell using SWCNTs electrodes on PET. Reproduced from Ref. [207]. (b) Photo of touch screen panel utilizing CNT film as touch electrode. Reproduced from Ref. [208]. (c) Array of the TFTs based on CNT electrodes on a plastic substrate. Reproduced from Ref. [209]. (d) OLEDs fabricated with a SWCNTs network anode. Reproduced from Ref. [210]. (e) Liquid crystal display with CNT top electrode. Reproduced from Ref. [211].
DeGraff et al.[212] developed a printable, flexible and, low profile strain sensor using aligned MWCNT thin films, which could sense tensile and compressive strains as low as 0.005% and as high as 1%. The sensors could be conformed to surfaces to a fabric glove, exhibiting good sensor performance in detecting of finger movements. Guan et al.[213] presented a glucose biosensor based on MWCNTs immobilized with glucose oxidase. Aligned MWCNTs were also applied as flexible electrodes in batteries and supercapacitors. Aligned MWCNTs were also applied as flexible electrodes in batteries and supercapacitors. Pandit et al.[214] synthesized free-standing flexible MWCNTs bucky paper with a high specific capacitance of 270 F/g and superb stable behavior (88% at 10000 cycles), which was promising as electrode of supercapacitors.
The performances of those devices can be further enhanced following the synthesis of CNTs with high purity and identical length, diameter and chirality[144, 199]. Thus more efforts should be made in those aspect.
3.2.3
Carbon nanobud (CNB)
Fullerenes also exhibit many advantageous properties like SWCNT. Despite the similarities between these two forms of carbon nanomaterials, few attempts have been made to connect these two carbon nanomaterials until 2007. Nasibulin et al.[215] discovered nanobud, a novel hybrid carbon nanomaterial that combines fullerenes and SWCNTs into a single structure (as illustrated in Figs. 9(a) and 9(b)). In this hybrid structure, the fullerenes are covalently bonded to the outer surface of the SWNTs. CNBs were prepared in two different continuous aerosol methods. One utilized particles grown in situ via ferrocene vapor decomposition[216]. The other method used pre-made iron-catalyst particles produced by a hot wire generator[217].
Due to the higher reactivity of fullerenes compared with SWNTs, CNBs are much easier to be functionalized through chemical modification[218]. The attached fullerene molecules can weaken bundling adhesion forces and thus would allow better dispersion of the material and facilitate the ink preparation process[219]. Additionally, the attached fullerenes act as molecular anchors to prevent slipping of SWCNTs in composites, thus improving the composite's mechanical proper ties[214]. Moreover, due to the electron transport between SWCNTs and functionalizing fullerenes, both electrical and optical properties of the CNBs can be tuned. In the past ten years, many research studies focusing on physical properties of CNBs were carried out[220–222].
Ahangari et al.[223] investigated the structural, electronic, and mechanical properties of armchair (6, 6) and zigzag (10, 0) CNBs using the first-principles density functional theory (DFT) method. The CNBs were observed to be semiconducting regardless of whether the original SWCNT base was metallic or semiconducting. With attaching a C60 fullerene molecule to zigzag and armchair SWCNTs, the Young’s moduli of the CNBs decreased to 0.85 and 0.94 TPa, respectively. The Young’s modulus of a zigzag CNB is lower than that of an armchair CNB due to the existence of a weak interaction between the C60 molecule and the zigzag SWCNT. Zhao et al.[224] investigated the electronic transport properties of the CNBs with different configurations using non-equilibrium Green's function and first-principles DFT. The results showed that the charge distribution could extend from the tube to the bud region, which was beneficial to the cold electron field emission and enabled the CNBs as a good candidate for nanoelectronics.
In industry, a Finnish firm called Canatu has manufactured CNBTM transparent conductive films by aerosol synthesis, direct dry printing (DDP) and laser ablation[225]. DDP was a new thin film manufacturing method based on the work described in Kaskela et al.[226] and the thermophoretic technique described in Gonzales et al.[227]. The combination of aerosol synthesis and DDP is a simple, scalable, low cost and environmentally friendly thin film manufacturing process which enables patterned deposition on any substrate at ambient surroundings. The produced CNB films exhibit high transparency, conductivity, flexibility and stretchability for high-quality touch applications.
Canatu has been able to approximately double CNB film conductivity at a given transparency each year since 2007. Currently, Gen 5 films are manufactured with the following properties: 100 Ω/sq at 94%, 150 Ω/sq at 96%, and 270 Ω/sq at 98%. In the lab, the transparency could achieve as high as 100 Ω/sq at > 95%, which is needed for enabling bright display images and pattern invisibility. The sheet resistance of the films could remain nearly constant over 30000 cycles of 180° bending. In another similar test, the increase of resistivity was less than 7% in 140 000 bend cycles [225].
By using commercially available CNB films, flexible and 3D shaped touch sensor and high optical quality touch display device were produces and are illustrated in Figs. 9(c) and 9(d), respectively[225].

class="figure_img" id="Figure9"/>
Download
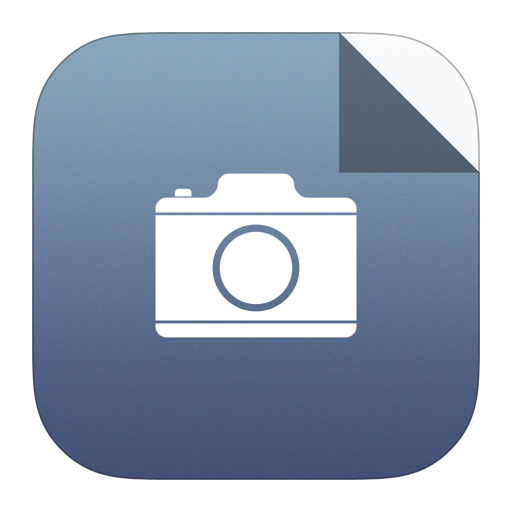
Larger image
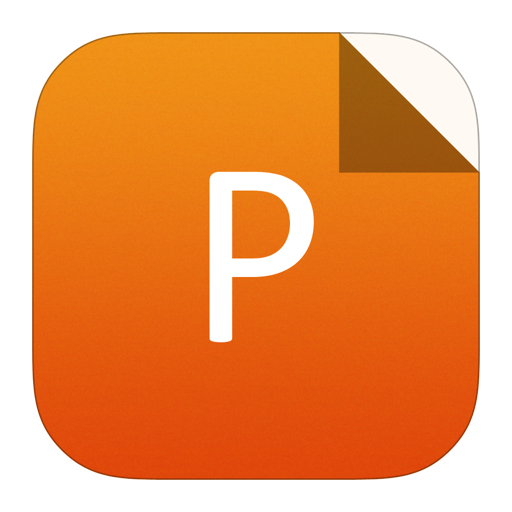
PowerPoint slide
Figure9.
(Color online) (a) Fullerene–SWNT hybrid structures, reminiscent of buds on a branch. Reproduced from Ref. [225]. (b) Morphology revealing the presence of spherical structures on the surface of the SWNTs. Reproduced from Ref. [225]. (c) Photo of a flexible CNB touch sensor. Reproduced from Ref. [225]. (d) CNB touch sensor integrated in an Intel Ultrabook reference design. Reproduced from Ref. [225].
3.3
Composite nanomaterials
As follows from the above demonstrations, more and more efforts have been devoted to fabricate electrodes by printing process and developing novel materials including conducting polymers, Ag NWs or Cu NPs, graphene, and CNTs in replacement of ITO with high electrical conductivity, transmittance, flexibility, thermal stability, and lower cost. Given the impressive progress made on these individual material and conductive ink for printing process, a promising concept of hybrid electrodes made of composite nanomaterials is increasingly popular. Hybrid electrodes made of graphene/Ag NWs[228, 229], Ag NWs/Ag grid[230], CNT/Ag[231], ZnO NPs/ Ag NW[232], polyethyleneimine(PEI)/Ag/PEDOT : PSS[233], Cu NW/AZO[234], Ag NWs/GO[235], Ag NWs/ITO[236], graphene/PEDOT:PSS[237], Ag NWs/PEDOT : PSS[238, 239] are all demonstrated.
Lee et al.[228] produced a transparent and stretchable electrode based on graphene/Ag NWs hybrid nanostructure (as illustrated in Fig. 10(a)). The obtained electrode exhibited excellent mechanical flexibility (folding with bending radius of 3.7 μm and bending strain of 27%), good stretchability (10000 times with stretching strain of 100% toward the uniaxial direction and 30% tensile strain toward the multi-axial direction), and good stability against electrical breakdown and thermal oxidation.
Ye et al.[230] reported an inkjet-printed Ag grid combined with Ag NWs to form a transparent hybrid electrode (the microstructure is illustrated in Fig. 10(b)) with low resistivity (22.5 Ω/sq) while maintaining a high transmittance (87.5%), which was comparable to standard ITO[18].
Hu et al.[231] reported a novel method to improve the electrical conductivity of Ag electrode films by implanting CNTs into silver ink. The microstructure of the electrode is illustrated in Fig. 10(c). The resistivity of screen-printed Ag/CNTs film was decreased by 62.27% compared with that made from pure Ag film and exhibited excellent flexibility under a bending radius of 4 mm over 1000 cycles. They concluded that those improvements were attributed to the interconnected network structure of the Ag/CNTs, which provided electronic transport channels and prevented cracks from initiating and propagating.
Morgenstern et al.[232] reported the solution processable Ag NW films coated with ZnO NPs which acted as an electron extraction layer. Ag NWs had average dimensions of 6.5 μm length and 80 nm diameter while ZnO NPs have average diameters of 5 nm. The conductivity was slightly increased while mechanical and thermal stabilities of Ag NW films were efficiently enhanced. Inverted solar cells using Ag NWs/ZnO hybrid as transparent electrode reached power conversion efficiencies of 2.4%. The device structure is illustrated in Fig. 10(d).
Kang et al.[233] fabricated flexible transparent electrode consisting of ultra-thin Ag film between the PEI and PEDOT:PSS layer (as shown in Fig. 10(e)). The hybrid electrode showed high performance, including a visible-range transmittance > 95% and a sheet resistance < 10 Ω/sq. The hybrid electrodes were incorporated into solar cells and the solar cell exhibited high efficiency of 10%.
Won et al.[234] fabricated low-cost AZO/CuNWs/AZO hybrid electrodes. The embedded structure of Cu NWs dramatically enhanced the thermal and oxidation stability of Cu NWs. These hybrid electrodes could maintain high transparency (83.9% at 550 nm) and low sheet resistance (35.9 Ω/sq) at a bending radius of 2.55 mm within 1200 cycles. A thin film solar cell with power conversion efficiency of 7.1% was fabricated using the hybrid electrodes. The structure of the solar cell is illustrated in Fig. 10(f).

class="figure_img" id="Figure10"/>
Download
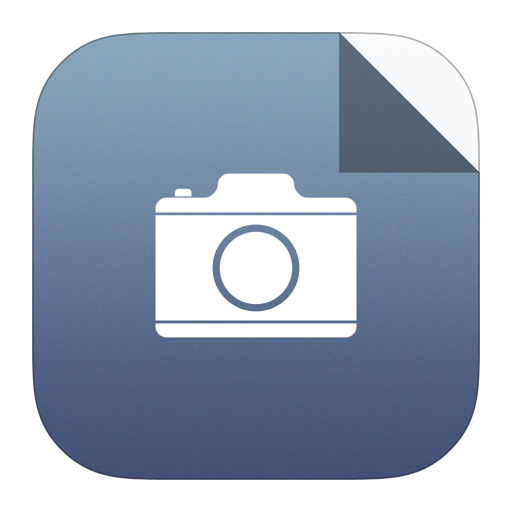
Larger image
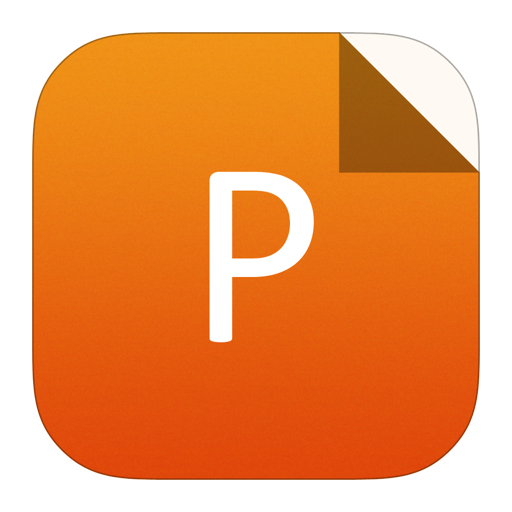
PowerPoint slide
Figure10.
(Color online) (a) Microstructures of conducting film based on graphene-Ag NW structures. Reproduced from Ref. [228]. (b) Microstructures of hybrid electrode of Ag NWs combined with Ag grid. Reproduced from Ref. [230]. (c) CNT enriched silver electrode films. Reproduced from Ref. [231]. (d) Device structure of a bulk heterojunction inverted solar cell using a ZnO NPs coated Ag NW film as the transparent electrode. Reproduced from Ref. [232]. (e) Hybrid electrode consisting of an ultra-thin Ag layer between PEI and PEDOT:PSS. Reproduced from Ref. [233]. (f) Structure of solar cells using AZO/CuNWs/AZO hybrid electrodes. Reproduced from Ref. [234].
As shown above, the combination of several types of materials can greatly enhance the mechanical properties, conductivity, and optical transparency of composite materials compared with individual materials. Those reports[228, 230, 231, 233] suggested that hybrid electrodes made of composite materials are a promising concept for the applications in transparent conducting technologies.
4.
Summary and outlook
Looking back conductive nanomaterials for electrodes by printing, several viewpoints on material research and developments, and applications are concluded as below.
Intelligent electronic technology, information technology, and big data are undoubtedly hot topics of the future world. Personalized, intelligent, portable electronic devices will become increasingly widespread in our daily life, which spur demand of ultrathin, uniform, large area, flexible, and transparent conductive electrodes with excellent optoelectronic and mechanical properties. In the past decades, the performance of printed flexible electrodes fabricated from metal NWs/NPs, graphene, CNT, and polymers all have been studied, especially for optical and electrical properties. However, there should be no limit for materials, and more innovative properties like biocompatibility will be discovered. The application field will be extended to biology and medical care fields due to the large demand of intelligent signal detectors, smart information processors, and biosensors in these areas (as typically shown in Fig. 11[240]). Electrodes and other conductive components are indispensable in those devices with functions of signal detection and transmission.

class="figure_img" id="Figure11"/>
Download
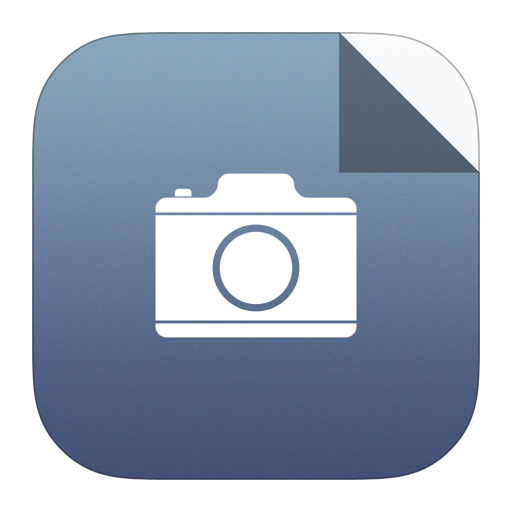
Larger image
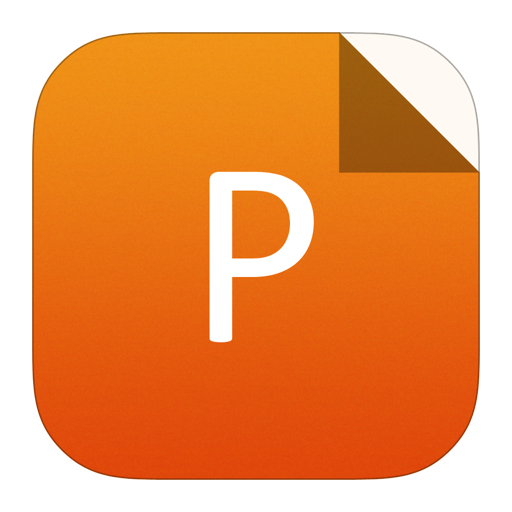
PowerPoint slide
Figure11.
(Color online) Diagram of the wearable medical devices. Reproduced from Ref. [240].
On the one hand, printed electronics are hopeful to solve industrial problems and serve the mass production. On the other hand, researchers are developing more advanced functional materials to provide more novel alternatives for printed electrodes such as composite materials. However, the key factor that must be considered in industrial production is the good balance of performance-quality-cost[241], which restricts the application of many conductive nanomaterials. Performance means the as-processed products could exhibit high purity, conductivity, excellent morphology, and transparency. Quality refers to the good quality control process to reduce defects for certain manufacturing process. The most critical factor is the cost, which relates to raw material cost, investment of equipment, and processing cost[75, 141].
Therefore, simple synthesis methods and low cost for conductive ink, printing techniques with higher resolution, and low temperature post-annealing process are still the obstacles for mass production of highly conductive printed electrodes. Many efforts are still needed in this promising field.