1.
Introduction
Flexible energy conversion and storage systems have gained tremendous interest during the past few years due to the rapid development of flexible intelligent electronics such as electronic skins[1–3], implantable medical sensors[4, 5] and smart phones[6, 7]. Among various energy conversion systems, flexible organic solar cells (FOSCs) based on the bulk donor/acceptor heterojunction mechanism are of great promise because of their attributes in light weight and good mechanical flexibility, and the use of low-cost raw materials[8–13]. Recent breakthroughs in achieving high power conversion efficiency (PCE) of rigid-type OSCs over 10%[16–23] also provides enormous encouragement in the pursuit of high-efficient FOSCs. Another great advantage of FOSCs over conventional Si and thin-film solar cells is the potential compatibility with high-throughput full-solution roll-to-roll printing, which can meet the cost effectiveness for practical applications, even the PCE of the device is only a few percent. Full-solution processing of FOSCs refers to constructing all functional layers including transparent electrode, photoactive layer, bottom back electrode, and all interfacial layers via solution manners such as casting and printing[24–37]. Till now, the transparent electrode[38–41] and photoactive layer with controllable microstructure and satisfied performance can be easily achieved by using solution processing methods such as spin-coating and ink-jet printing. On the other hand, printed metal electrodes are fabricated through top-down printing of nano/micro-sized metal particles, followed by sintering of the particles to form conductive electrodes. However, metal thin film fabricated through this top-down method typically requires high temperatures that are not suitable for flexible devices, especially for metals that are easy to oxidize, e.g., Cu. In addition, the printed metal electrodes show a high degree of roughness, as compared with metal deposited ones. Therefore, works towards solution-processed metal electrodes with superb electrical and mechanical properties are rarely reported due to the dramatic difficulty in making these metal layers thin and smooth enough. To date, metal electrodes, particularly the bottom electrodes, are often fabricated through vacuum evaporation of metals (Al, Cu, Ag, Au)[42–46], which inevitably increases the production costs and deteriorates the mechanical flexibility.
To address this issue, we have developed polymer-assisted metal deposition (PAMD), a solution approach to fabricate high-quality flexible metal electrodes at low temperature[47–50]. Unlike top-down fusion of nano/micro-sized metal particles in conventional printable metal inks, PAMD uses a bottom-up approach to grow dense metal layers such as Cu at low temperature via electroless deposition (ELD) with the aid of a surface-grafted polymer. This bottom-up approach enables the high conductivity, controllable thickness and roughness, excellent fatigue resistance, and low cost of the resultant metal electrodes. We recently demonstrated that full-solution processed FOSCs using a printed Cu cathode through PAMD (PAMD-Cu) showed much superior flexibility and device stability with record-breaking PCE[32], when compared with the reference devices with Cu cathode by vacuum evaporation.
However, that proof-of-concept work did not provide a detailed study on the interfacial properties and modification of the printed metal electrodes. It is well-known that the conductivity and surface roughness of the back bottom electrode play an important role in the device performance of OSCs[51, 52]. More importantly, the interface modification layer (IML) between the photoactive layer and the metal electrode is critical to the overall performance, because of its ability to facilitate the extraction and transporting of charges, to suppress the leakage current, and to act as an optical spacer for maximizing the light harvesting[53, 54].
In this paper, we systematically investigate the surface properties of printed metal electrodes made via PAMD and their interfacial engineering with different IMLs. Two types of metal electrodes, PAMD-Cu and PAMD-Ag, are prepared and used as the back bottom cathodes. Branched polyethylenimine (PEI) and ZnO thin films are utilized as IMLs to modulate the surface morphology and work function (WF) of the PAMD metals. Poly(3-hexylthiophene) and 1-(3-methoxycarbonyl) propyl-1-phenyl[6,6]C61 (PC61BM)blend (P3HT : PCBM) is used as the model absorber. The morphology of PAMD electrodes and the effect of IMLs on electron transporting and light absorption are carefully characterized and discussed through both experimental and stimulation studies. With optimized interfaces, we demonstrate full-solution processed FOSCs with PCE over 3% and remarkable device stability at 500 cycles of flexibility tests. To the best of our knowledge, this is the most efficient full-solution processed FOSC reported in the literature.
2.
Experimental section
2.1
Materials
Poly(ethylene terephthalate) (PET) sheets with the thickness of 175 μm were supplied by the DuPont Company. PH1000, a solution of high conductive Poly(3,4-ethylenedioxythiophene):poly(styrenesulfonate) (PEDOT : PSS), was purchased from Heraeus Clevios. Poly(3-hexylthiophene) (P3HT) was purchased from Rieke Metals, Inc. 1-(3-methoxycarbonyl) propyl-1-phenyl[6,6]C61 (PC61BM) was purchased from American Dye Source, Inc. Poly[2-methoxy-5-(2-ethylhexyloxy)-1, 4-phenylenevinylene](MEH-PPV) was purchased from Guangzhou Lijian Co.. Poly (ethylene glycol) (PEG) (Mw = 4000 g/mol) and potassium peroxodisulfate (KPS) were purchased from Uni-Chem. Ammonium tetrachloropalladate(II) ((NH4)2PdCl4), [3-(methacryloyloxy)propyl] trimethoxysilane (MPS), 2-(methacryloyloxy)ethyl-trimethylammonium chloride (METAC) (80 wt.% aqueous solution), polyethylenimine (PEI), dimethylsulfoxide (DMSO) and all other chemicals were purchased from Sigma-Aldrich.
2.2
Fabrication of printed Cu and Ag cathodes via PAMD
PET substrates were washed with detergent, ultrasonically cleaned in acetone and ethanol, and dried by clean compressed air before use. After cleaning, PET substrates were plasma-treated for 4 min or immersed in NaOH solution (1 M) for 10 min, and immersed into a 0.5 wt% MPS solution (95% EtOH, 1% HAc) for 1 h, followed by rinsing. Then these substrates were immersed into an aqueous solution of 2-(methacryloyloxy)ethyl-trimethylammonium chloride (METAC) (80 wt.% aqueous solution) (20 wt%) and potassium peroxodisulfate (KPS) (2.5 g/L), and heated at 60 °C for 60 min for polymerization. Screen printing of catalytic ink ((NH4)2PdCl4, (23 mg), polyethylene glycol (PEG, 5 g, Mw = 4000 g/mol) and deionised (DI) water (1.3 g)) was performed on the PMETAC-modified substrates to form the ink patterns. At the end, the ink-patterned substrates were placed in the dark for 30 min to load PdCl42? by ion exchange, followed by treating with plasma for 2 min and rinsing with DI water to remove the ink completely. The ELD of Cu was performed in a plating bath consisting of a 1 : 1 mixture of freshly prepared solution A and B. Solution A contains NaOH (12 g/L), CuSO4·5H2O (13 g/L), and potassium sodium tartrate (29 g/L). Solution B is a diluted formaldehyde (17 mL/L) aqueous solution. The ELD of Ag was performed in a plating bath consisting of a 1 : 1 mixture of freshly prepared A and B solutions. Solution A contains NaOH (12 g/L), and potassium sodium tartrate (29 g/L) in DI water. Solution B is a formaldehyde (17 mL/L) aqueous solution. Then the activated PdCl42- loaded substrates were immersed in the Ag plating bath ([5 g/L Ag(NH3)2]NO3 and 50 g/L potassium sodium tartrate aqueous solution) for 2–10 min to achieve the printed Ag films with different thickness.
2.3
Fabrication of FOSCs
80 nm thick printed Ag and Cu electrodes on PET were prepared as described above. ZnO nanoparticles were prepared using an adapted procedure based on the work of Weller et al.[55]. The diameters of these nanoparticles are less than 5 nm. 40 nm thick ZnO film was formed by spin-coating from their chloroform (CF) or methoxyethanol (ME) solution on the metal electrode and then annealed for 30 min at 80–130 °C. PEI films were made by spin-coating from its 0.5 wt% 2-methoxyethanol solution on metal electrodes or ZnO at 5000 rpm for 60 s in a nitrogen-filled glove box and annealed at 100 °C for 10 min. Then, the active layer of OSCs was spin-coated on ETL at 800 rpm for 60 s in the glove box from the chlorobenzene solution of P3HT:PC61BM (10 : 8 in weight ratio) and annealed at 145 °C for 5 min. Finally, doped PH1000 was spin-coated on the active layer as the top electrode at 1000 rpm for 60 s, and then annealed at 130 °C for 20 min in the glove box to finish the device fabrication.
2.4
Optical simulation
The optical transfer matrix method was applied to simulate the optical absorption and normalized optical electric field distribution (|E/E0|2). The time average light energy absorbed at single wavelength per time unit of active layer in the device, Q, was obtained by the following equation:
$$Q = frac{1}{2}omega varepsilon '{varepsilon _0}{left| {Eleft( lambda ight)} ight|^2},$$ ![]() | (1) |
where ω was the angular frequency of the electromagnetic field, ε0 was the electric permittivity of free space, and ε′ was the imaginary part of the dielectric function; λ was the wavelength. Q was integrated by the volume of active layer, and then was normalized to the incident light density to obtain the absorption spectrum, abs(λ). TAP was calculated by the equation below:
$${ m TAP} = int_{400}^{700} {frac{{Sleft( lambda ight){ m abs}left( lambda ight)lambda }}{{hc}}{ m d}lambda } ,$$ ![]() | (2) |
where h was Planck constant, c was the speed of light, and S(λ) was the AM 1.5 spectra.
2.5
Characterization
Ultraviolet photoelectron spectroscopy (UPS) was measured by the ESCALAB 250Xi X-ray Photoelectron Spectrometer. Atomic force microscopy (AFM) was performed with an XE-100 (Park System) by non-contact mode. ZnO nanoparticles were characterized by using transmission electron microscopy (TEM, JEOL JEM-2010). A Keithley 2400 source meter was used to measure the current density–voltage (J–V) characteristics of the solar cells, and a 300 W Oriel solar simulator (91160, Newport, 100 mW/cm2, equipped with AM1.5 filter) calibrated by a standard silicon solar cell was used as the light source. The external quantum efficiencies (EQEs) of the devices were measured with a standard system equipped with a xenon lamp (Oriel 66902, 300 W), a monochrometor (Newport 66902), a Si detector (Oriel 76175_71580) and a dual channel power meter (Newport 2931_C).
3.
Results and discussion
As shown in Scheme 1, FOSCs were fabricated though a full-solution layer-by-layer construction approach. Firstly, Ag or Cu electrodes with a thickness around 80 nm were prepared on PET substrates (175 μm in thickness) by using the PAMD technique in solution. The fabrication procedures are similar to those we published previously32 and the details can be found in the experimental part. In brief, the electrodes were obtained via multi-stage reactions, which included surface modification of PET with poly[2-(methacryloyloxy) ethyl-trimethylammonium chloride] (PMETAC) brushes, immobilization of (NH4)2PdCl4 through ions exchange, and ELD of metal. Unlike traditional ELD, the PAMD process renders metal films strongly anchored on substrates with the aid of the surface-anchored polymer layer. Consequently, these metal electrodes exhibited superb conductance stability when undergoing mechanical deformation such as bending, compressing, and stretching[47–50]. Compared with PAMD-Ag, PAMD-Cu is easier to be oxidized. The printed metals presented a high thermal stability in the glove box. An additional high-temperature annealing in N2 is helpful to enhance the stability of the printed metal electrodes. After obtaining the flexible bottom cathode, uniform PEI (15 nm) or/and ZnO (50 nm) thin films served as the IML were then spin-coated onto the surfaces of the metal electrodes. The averaged diameter of synthesized ZnO nanoparticles is less than 5 nm based on TEM observations (Fig. S3). Two types of ZnO films with different morphologies were prepared by spin-coating from CF solution in air or ME solution in N2. The resultant IMLs were denoted as CF-ZnO and ME-ZnO, respectively. To clarify the effect of PAMD metal electrodes and IMLs on the photovoltaic performance of FOSCs, model materials P3HT: PC61BM and PH1000 were subsequently spin-coated to form the active layer and the top transparent anode, respectively. The thicknesses of the active layer and the top electrode in all devices were carefully controlled respectively to be 200 nm and 120 nm to eliminate any fluctuations in photovoltaic performance caused by them.

class="figure_img" id="Scheme1"/>
Download
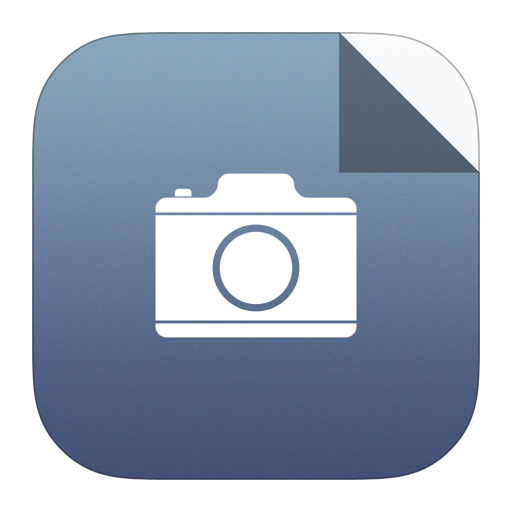
Larger image
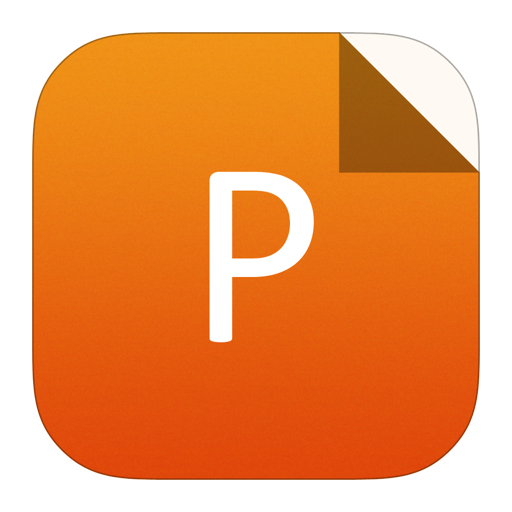
PowerPoint slide
Scheme1.
(Color online) Schematic illustration of the fabrication process of FOSCs based on PAMD metal electrodes.
The thicknesses of PAMD-Ag and PAMD-Cu on PET sheets were ca. 80 nm by controlling the ELD time. Four-probe measurements presented the sheet resistance of PAMD-Cu lower than 1 Ω/□. The sheet resistance of PAMD-Ag was even lower, which was ~300 mΩ/□. The surface morphology of obtained metal electrodes was observed by using AFM and typical results are listed in Table S1. It can be observed that Ag films with the RMS roughness of 12.0 nm have been evenly coated on the PET surface. The films are formed by the aggregation of Ag nanoparticles with the average diameter of ca. 80 nm, typical of the seed growth process[56]. Noticeably, the PAMD-Cu film on PET substrate showed a RMS roughness of 1.9 nm, which is almost equal to that of the thermal evaporated Cu film.
The WFs of as-prepared PAMD metal cathodes were measured by using ultraviolet photoelectron spectroscopy (UPS). As shown in Fig. 1, the WFs of PAMD-Ag and PAMD-Cu were 4.7 and 4.5 eV, respectively, which are nearly the same as those of evaporated Ag and Cu. After coating with PEI or ZnO, the WFs of such metal cathodes were dramatically reduced. From Fig. 1, it can be found that the PEI IML can reduce the WFs of PAMD metals more effectively than ZnO, which may be due to the strong interfacial dipole induced by the large number of amine groups[27]. By coating with PEI, the WFs of PAMD-Ag and PAMD-Cu were decreased to 3.5 and 3.3 eV, respectively. In comparison, the WFs of PAMD-Ag and PAMD-Cu modified with ZnO were 4.0 and 3.9 eV. It is worth noting that ZnO IMLs prepared based on different solutions showed a negligible effect on the WF decrement. Both PEI and ZnO could successfully tune the WFs of the cathode below 4.0 eV, which matches the lowest unoccupied molecular orbital (LUMO) of PC61BM (3.7 eV). Thus, electron transport from the active layer to the metal cathode should be enhanced under the assistance of these IMLs. Meanwhile, considering their large band gaps (3.3 eV for ZnO, 6.5 eV for PEI), both ZnO and PEI can block the hole injecting into cathodes from the highest occupied molecular orbital (HOMO) of P3HT. It is also found that an additional PEI layer can further reduce the WF of ZnO-modified metal electrode. For example, the PAMD-Cu/ZnO/PEI electrode shows a WF of 3.6 eV, which is 0.3 eV lower than that of PAMD-Cu/ZnO. The energy level diagrams of the whole solar cells are shown in Fig. S1.

class="figure_img" id="Figure1"/>
Download
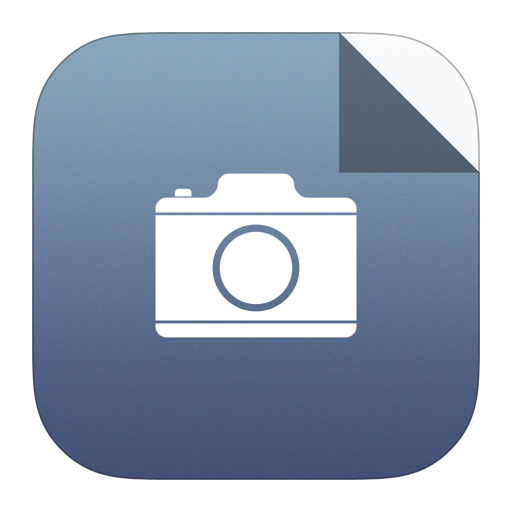
Larger image
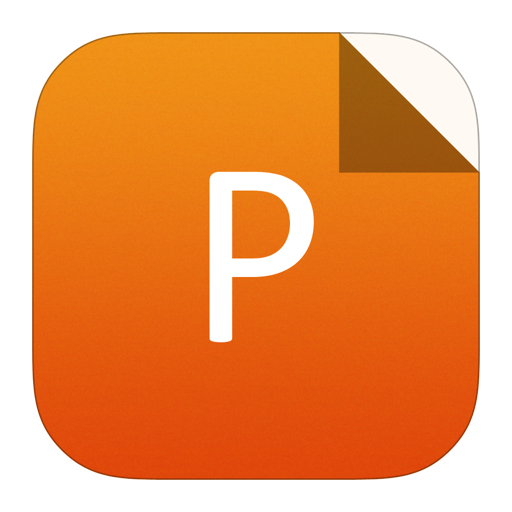
PowerPoint slide
Figure1.
UPS spectra of PAMD metals with/or IMLs.
To check the effect of IMLs on light absorption of the active layer, an optical model was built on the basis of the current device structure. The optical transfer matrix method was used in the simulation for the wavelength range from 400 to 700 nm. The actual optical constants and thickness of metal bottom cathode, PEI or ZnO IML, active layer, and PH1000 top anode were put into the simulation to calculate the distribution of electric field density in the device. As shown in Fig. 2, strong electric field coupling in the wavelength range of 400 to 600 nm appeared inside the active layer, which matched well with the absorption spectrum of P3HT:PC61BM. Because light absorption is proportional to the square of electric field density, the strong electric field coupling is conducive to the output current of OSCs. It should also be noted that, the intensity and profiles of electric field density in the active layer are quite different in various OSCs. Compared with the devices using a Cu cathode, higher electric field density within the active layer is always obtained in the devices based on a Ag cathode due to the higher reflectance of Ag. We also find that the electric field density in the range of 400–600 nm of the devices with a PEI IML is obviously higher than those using a ZnO IML. In addition, the different thicknesses of PEI and ZnO with various optical constants also gives rise to different optical spacer effects. In our device structure, the optical thickness of ZnO is larger than that of PEI, leading to a larger light phase. As a result, only a maximum intensity at 550 nm is located within the active layer in devices using ZnO IML, while two intensity maxima inside the active layer can be observed in devices using PEI IML. These results suggest that higher PCE and short circuit current density (Jsc) will be achieved in OSCs by utilizing PEI as the IML.

class="figure_img" id="Figure2"/>
Download
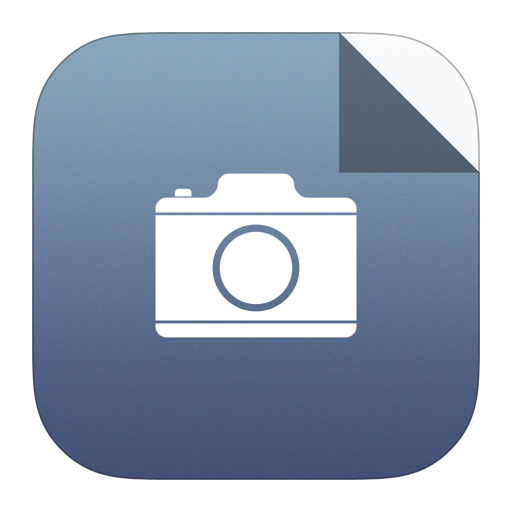
Larger image
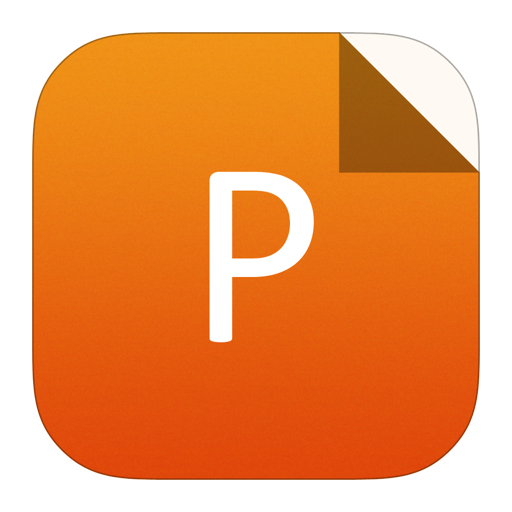
PowerPoint slide
Figure2.
(Color online) Optical simulations of total absorbed photons in device: (a) Ag(80 nm)/PEI(15 nm)/active layer(200 nm)/PH1000(120 nm), (b) Ag(80 nm)/ZnO(50 nm)/active layer(200 nm)/PH1000(120 nm), (c) Cu(80 nm)/PEI(15 nm)/active layer(200 nm)/PH1000(120 nm); and (d) Cu(80 nm)/ZnO(50 nm)/active layer(200 nm)/PH1000(120 nm).
Photovoltaic parameters of FOSCs with different metal cathodes and IMLs are summarized in Table 1. Data for each kind of FOSCs are averaged values obtained from 20 devices. Based on nearly the same open circuit voltage (Voc) and fill factor (FF), both PEI and ZnO are shown to be effective interface modifiers for the PAMD metal electrodes. Due to the superior reflectance over the Cu electrode, the PAMD-Ag electrode endowed OSCs with higher light absorption, leading to a higher Jsc. Moreover, for the OSCs based on the same metal electrodes, devices with PEI IML always showed higher Jsc than those utilizing ZnO. The highest device performance was achieved from the OSCs based on a PAMD-Ag cathode and PEI IML, which showed a Voc of 0.58 V, FF of 0.47, Jsc of 11.10 mA/cm2, and a PCE of 3.03%. To the best of our knowledge, this PCE is the highest value of full-solution processed OSCs using P3HT:PC61BM as a light absorber.
Metal electrode | ETL | Jsc (mA/cm2) | Voc (V) | FF | PCE (%) | Rs (Ω·cm2) | Rsh (Ω·cm2) |
PAMD-Ag | PEI | 11.10 | 0.58 | 0.47 | 3.03 | 12 | 285 |
PAMD-Ag | CF-ZnO | 8.60 | 0.58 | 0.49 | 2.45 | 12 | 230 |
PAMD-Ag | CF-ZnO/PEI | 8.39 | 0.58 | 0.46 | 2.24 | 19 | 310 |
PAMD-Ag | ME-ZnO | 10.01 | 0.58 | 0.36 | 2.11 | 32 | 166 |
PAMD-Ag | ME-ZnO/PEI | 8.70 | 0.58 | 0.45 | 2.27 | 24 | 232 |
PAMD-Cu | PEI | 7.88 | 0.58 | 0.55 | 2.52 | 15 | 718 |
PAMD-Cu | ME-ZnO | 7.52 | 0.58 | 0.47 | 2.07 | 21 | 320 |
PAMD-Cu | ME-ZnO/PEI | 7.22 | 0.58 | 0.45 | 1.90 | 28 | 277 |
Table1.
Photovoltaic parameters of the OSCs based on PAMD metal electrode.
Table options
-->
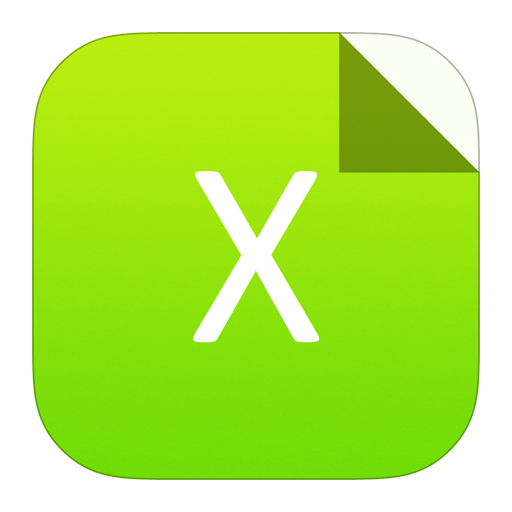
Download as CSV
Metal electrode | ETL | Jsc (mA/cm2) | Voc (V) | FF | PCE (%) | Rs (Ω·cm2) | Rsh (Ω·cm2) |
PAMD-Ag | PEI | 11.10 | 0.58 | 0.47 | 3.03 | 12 | 285 |
PAMD-Ag | CF-ZnO | 8.60 | 0.58 | 0.49 | 2.45 | 12 | 230 |
PAMD-Ag | CF-ZnO/PEI | 8.39 | 0.58 | 0.46 | 2.24 | 19 | 310 |
PAMD-Ag | ME-ZnO | 10.01 | 0.58 | 0.36 | 2.11 | 32 | 166 |
PAMD-Ag | ME-ZnO/PEI | 8.70 | 0.58 | 0.45 | 2.27 | 24 | 232 |
PAMD-Cu | PEI | 7.88 | 0.58 | 0.55 | 2.52 | 15 | 718 |
PAMD-Cu | ME-ZnO | 7.52 | 0.58 | 0.47 | 2.07 | 21 | 320 |
PAMD-Cu | ME-ZnO/PEI | 7.22 | 0.58 | 0.45 | 1.90 | 28 | 277 |
We also noted that the FOSCs based on Ag/CF-ZnO and Ag/ME-ZnO bottom electrodes displayed quite different Jsc and FF. A higher Jsc and a lower FF confirmed that a high leakage current in the OSCs with the Ag/ME-ZnO bottom electrode (Fig. 3(b)), which is thought to be ascribed from the different morphologies of ZnO IMLs. Therefore, the surface morphologies of different bottom electrodes were studied by AFM (Fig. 4). RMS of the different IMLs with two scan sizes of 1 × 1 μm2 and 10 × 10 μm2 are shown in Table S1. The Ag/CF-ZnO presented a low roughness of 4.8 nm, which is better than the 12.0 nm of Ag/ME-ZnO. During the formation of ME-ZnO films under N2 atmosphere, ZnO nanoparticles continuously aggregated and formed larger particles with diameters around 70 nm during annealing. However, more smooth CF-ZnO films could be achieved owing to the better disperse ability of ZnO in CF and the assistance of water in air on dense film formation (Fig. S3). High roughness will obviously bring a large leakage current, and the resultant small Rsh will lead to a lower FF. It has been reported that PEI was able to lower the WFs of the sol–gel derived ZnO, resulting in improvedJsc, Voc, and FF[45]. Here we also used PEI to further optimize the surface morphology of ZnO films and to enhance the photovoltaic performance. From Table 1, it can be observed that the Voc of the OSCs showed nearly no change after coating another layer of PEI on top of ZnO, demonstrating that the WF of ZnO was low enough for the electron injection from LUMO of PCBM. Only for the OSCs based on Ag/ME-ZnO, was there an obvious improvement in the FF from 0.36 to 0.45. This should be attributed to the optimization on the surface morphology. For the more rough ME-ZnO film, the modification of PEI layer decreased the roughness from 12.0 to 8.2 nm, resulting in decreased leakage current and increased FF. In order to clarify the effect of IML on the electron mobility of the OSCs, the electron-only device with a structure of PAMD-Ag/IML/P3HT:PC61BM/Ca(20 nm)/Al (100 nm) was fabricated. At low voltage (0–2 V), the electron mobility of the device based on PEI IML was higher than that of the device based on ZnO IML (Fig. S4), which indicates that the carrier mobility contributes to Jsc of the device directly.

class="figure_img" id="Figure3"/>
Download
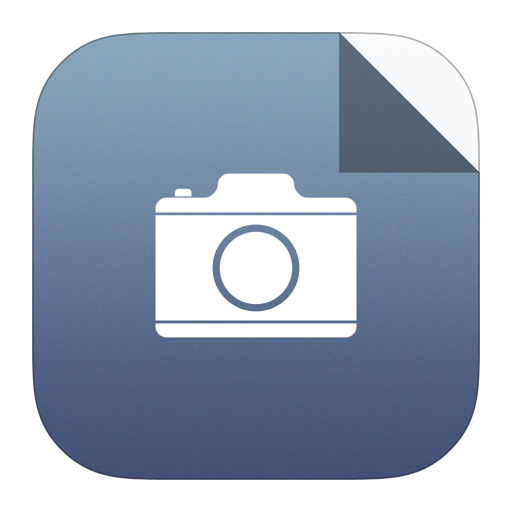
Larger image
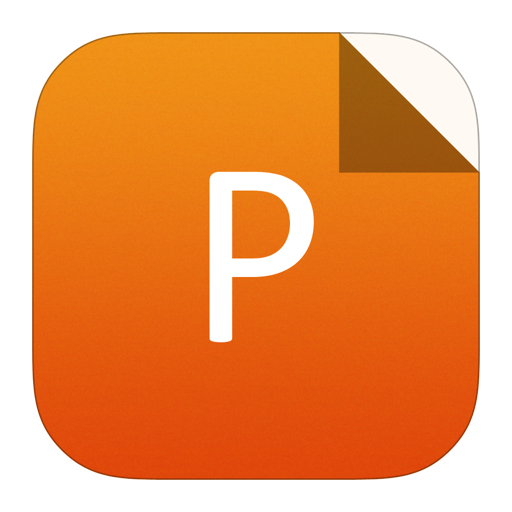
PowerPoint slide
Figure3.
(Color online) (a) J–V characteristics of FOSCs based PAMD-Ag under AM1.5. (b) Dark J–V characteristics of FOSCs based on PAMD-Ag under AM1.5. (c) Light and dark J–V characteristics of FOSCs based on PAMD-Cu. (c) EQE spectra of FOSCs based on PAMD metals.

class="figure_img" id="Figure4"/>
Download
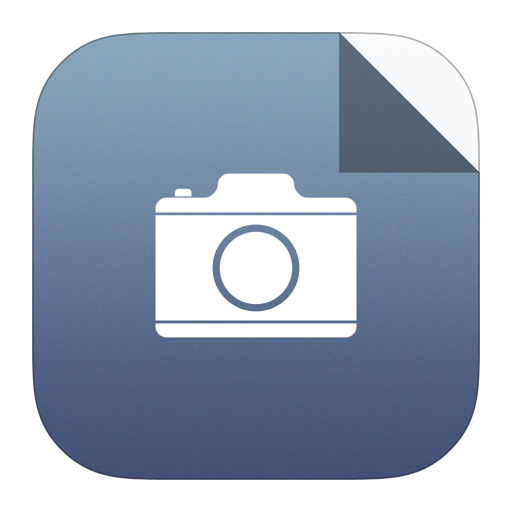
Larger image
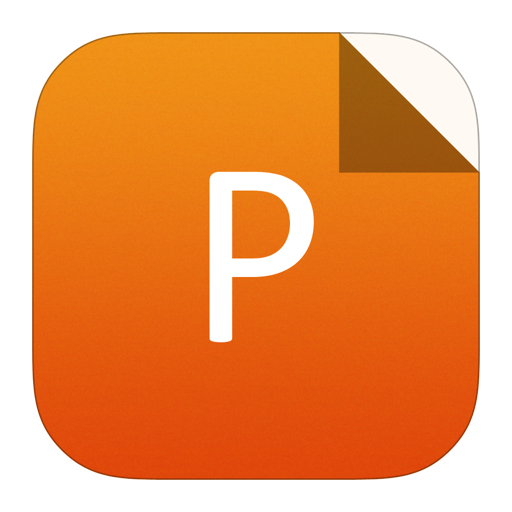
PowerPoint slide
Figure4.
(Color online) AFM images (1 × 1 μm2) of (a) PAMD Ag/PEI, (b) PAMD-Ag/CF-ZnO, (c) PAMD-Ag/ME-ZnO, (d) PAMD-Ag/CF-ZnO/PEI, (e) PAMD-Ag/ME-ZnO/PEI, (f) PAMD-Cu, (g) PAMD-Cu/PEI, (h) PAMD-Cu/ME-ZnO, (i) PAMD-Cu/ME-ZnO/PEI.
The light and dark J–V curves of the FOSCs based on the PAMD-Cu are shown in Fig. 3(c). Because Cu is easy to oxidize in the air, the PAMD-Cu electrodes were only modified by ME-ZnO in the glove box. The FOSC based on Cu/ME-ZnO presented an inferior device performance to that based on Cu/PEI, which was especially prominent in the lower FF. From the AFM image (Fig. S2), the relatively sharp protuberances of the ME-ZnO may cause larger leakage current, which is consistent with the dark J–V curves of the OSCs. We also used PEI to further smooth the Cu/ME-ZnO bottom electrode. But it was found that PEI could not improve the morphology of ME-ZnO obviously and the introduction of the PEI layer deteriorated the Jsc of the devices. External quantum efficiency (EQE) spectra of the OSCs based on PAMD metals with different IMLs were shown in Fig. 3(d). Besides the back metal electrodes, IMLs are also a big part of characterizing the photocurrent response profiles at the different wavelengths. The electrical field intensity can be tuned by the IMLs by shifting the propagation phase, thus moving the higher intensity into the active layers.
Mechanical flexibility of as-made FOSCs was evaluated by monitoring the photovoltaic parameters during bending cycles. Bending tests were carried out on FOSCs using PEI or ZnO single component IML. The devices were bent from +180° (stretch mode) to –180° (compression mode) in one bending cycle with a bending radius of 6.5 mm and a bending frequency of 0.5 Hz. Normalized parameters including Jsc, Voc, FF, and PCE were plotted as a function of bending cycles. Similar to other best works[57, 58], Voc of these devices remained constant even after 500 bending cycles. The optical images of the FOSCs based on PAMD-Ag are shown in Fig. S5. No obvious crack can be observed in the active area of the FOSCs by using PEI or ZnO as the IML. The small decrease in FF and Jsc may originate from the invisible nano-cracks occurring in the interface during the bending, which could slightly damage the ohmic contact, decrease the effective device area, and increase the leakage current. Compared with inorganic ZnO films, polymer films certainly possess the unique advantage in flexibility. Therefore, the devices utilizing 15 nm of PEI IML showed superior flexibility to those based on 50 nm of ZnO film. The roughness of the bottom electrodes also affected the flexibility of the FOSCs. FOSCs based on PAMD-Ag with the roughness of 12 nm presented the inferior flexibility to the OSCs based on PAMD-Cu (roughness was as low as 1.9 nm). The best flexibility was demonstrated from FOSCs based on a PAMD-Cu/PEI bottom electrode (Fig. 5). The Jsc and FF of the device decreased only by 2% and 3% respectively, resulting in a 5% reduction in PCE after 500 bending cycles. FOSCs based on PAMD-Cu/ZnO showed a 5% loss in Jsc and a 5% loss in FF, which brought a 10% reduction in PCE. Reductions of PCE by 7% and 13% were obtained for the OSCs based on PAMD-Ag/PEI and PAMD-Ag/ZnO.

class="figure_img" id="Figure5"/>
Download
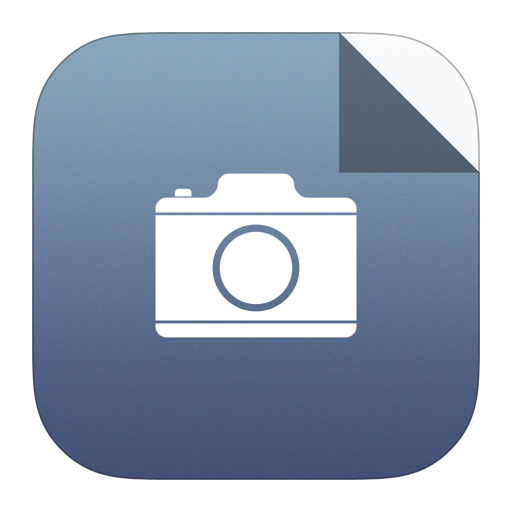
Larger image
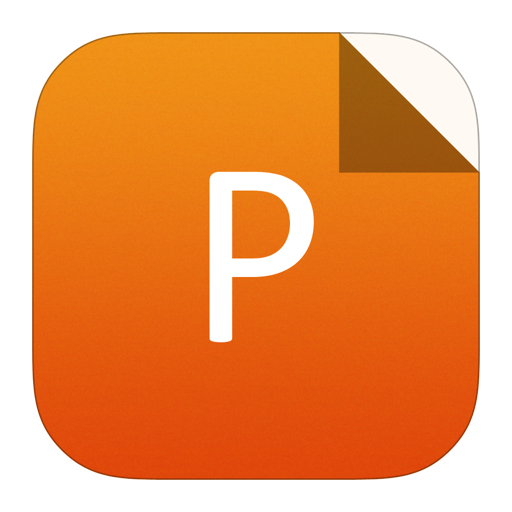
PowerPoint slide
Figure5.
(Color online) Normalized photovoltaic parameters of the OSCs with different cathodes and IMLs during 500 cycles of bending test. (a) PAMD-Ag/PEI. (b) PAMD-Ag/ZnO. (c) PAMD-Cu/PEI. (d) PAMD-Cu/ZnO.
4.
Conclusions
In conclusion, full-solution processed FOSCs using PAMD-Ag and PAMD-Cu bottom back electrodes have been fabricated, and the detailed interfacial modification and study on these metal electrodes have been carried out in this work. With different surface characteristics and electrical properties, both the electrodes were confirmed to be effective bottom back electrodes for FOSCs. It has been demonstrated that both PEI and ZnO are proper IMLs for the printed PAMD-Ag and PAMD-Cu cathodes. In addition, the solvent used and environmental humidity could change the morphology of ZnO IMLs that resulted from ZnO nanoparticle solution, which showed a remarkable effect on the FF of the device. PEI appeared to be a better choice than ZnO because of its higher flexibility and better film-forming quality. The champion device based on PAMD-Ag achieved a PCE of 3.03%, which appears to be the highest value reported on full-solution processed FOSCs using P3HT:PC61BM. The low-cost and low-temperature made PAMD-Ag and PAMD-Cu electrodes, which are compatible with high throughput roll-to-roll fabrication, are very promising to serve as the substitutes for those bottom back electrodes fabricated by vacuum deposition methods.