全文HTML
--> --> -->相干反斯托克斯拉曼散射(coherent anti-Stokes Raman scattering, CARS)技术最早由Moya等[4]在1975年应用于气体温度测量, 是一种较为先进的光谱测温技术, 具有非侵入式、空间分辨率高、信号便于收集等优点, 该方法在高温下测温的准确性已经得到了充分的验证[5-7]. 然而传统的纳秒CARS测温技术受限于激光器性能, 重复频率仅有10 Hz左右[8], 不适用于快速动态的温度测量, 同时纳秒CARS测温技术会受到非共振背景的干扰, CARS信号与非共振背景信号混叠在一起, 导致温度拟合变得更加困难. 而在纳秒CARS基础上发展的皮秒CARS测温技术虽然通过延迟探测脉冲避免了非共振背景干扰[9,10], 但是皮秒CARS技术信号的有效激发需要较高的单脉冲能量, 一般为上百毫焦, 而通常高能量的皮秒激光器的重复频率被限制在10—50 Hz范围内, 因此皮秒CARS测温技术的重复频率也仅有10—50 Hz, 动态测量能力十分有限[11,12]. 同时皮秒CARS测温无法忽略分子碰撞带来的影响[12,13], 建模过程十分复杂. 这些因素都限制了纳秒以及皮秒CARS测温技术在高温、湍流等实际环境下的测量精度与响应速度. 因此, 随着近年来飞秒激光技术的进步与成熟, 飞秒激光器所具有的高重频、高峰值功率以及宽线宽等特点, 推动飞秒CARS技术逐渐成为主流的CARS测温技术.
飞秒CARS测温技术根据原理的不同可大致分为3种, 即时间分辨飞秒CARS, 啁啾探测脉冲(chirped probe pulse, CPP)飞秒CARS以及混合飞秒/皮秒CARS. 时间分辨飞秒CARS测温技术需要利用机械位移台进行延时扫描探测[14-16], 单次温度的测量时间取决于位移台的扫描速度, 适用于稳定温度的测量, 无法满足实际环境下动态测量的需要; 而CPP飞秒CARS测温技术需要在光路中加入玻璃棒[17,18], 脉冲的功率受限于玻璃棒的损伤阈值, 从而限制了产生的CARS信号的强度, 导致高温情况下信号容易被淹没在噪声中, 同时CPP飞秒CARS的模型较为复杂, 参数计算可能需要数天时间[19], 因此CPP飞秒CARS测温技术在实际应用中局限较大; 混合飞秒/皮秒CARS技术则是利用飞秒激光作为激发光, 皮秒激光作为探测光, 从而可以在单脉冲内分辨分子精细的振动与转动跃迁结构[20-23], 是目前较为前沿的CARS测温技术, 更有潜力应用于高温、变化的复杂燃烧场的温度测量中, 然而目前该技术在高温动态燃烧场中的应用与研究相对较少[24].
本文报道了一种采用二次谐波带宽压缩方法[25,26]的混合飞秒/皮秒CARS测温方法, 利用飞秒脉冲在BBO晶体中的和频过程所获得的窄带高能皮秒脉冲作为探测脉冲, 在高温火焰中实现了千赫兹、单脉冲的温度测量. 针对实际应用中高温动态的复杂燃烧场, 本文利用标准燃烧器通过快速改变甲烷/空气的流速配比, 模拟了1700—2200 K 温度范围内的动态高温火焰. 在实验中, 利用该测温方法, 以千赫兹的光谱采集速率, 在模拟的动态高温火焰中进行了连续70 s测量, 验证了该方法在高温动态环境下测温的准确性、稳定性与响应速度.
2.1.CARS基本原理
CARS属于三阶非线性效应中的四波混频效应, 其能级跃迁过程见图1, 当泵浦光(ω1)与斯托克斯光(ω2)的频率差(ω1 – ω2)等于介质分子的拉曼共振频率ωR时, 介质分子本征的振动或转动得到共振增强, 此时探测光(ω3)入射, 产生向高频方向偏移的CARS信号(ω4). CARS的过程满足动量守恒与能量守恒, 即k 4 = k 1 – k 2 + k 3, ω4 = ω1 – ω2 + ω3, 其中k 1, k 2, k 3 k 4与ω1, ω2, ω3, ω4分别为泵浦光、斯托克斯光、探测光以及CARS信号所对应的波矢与中心频率.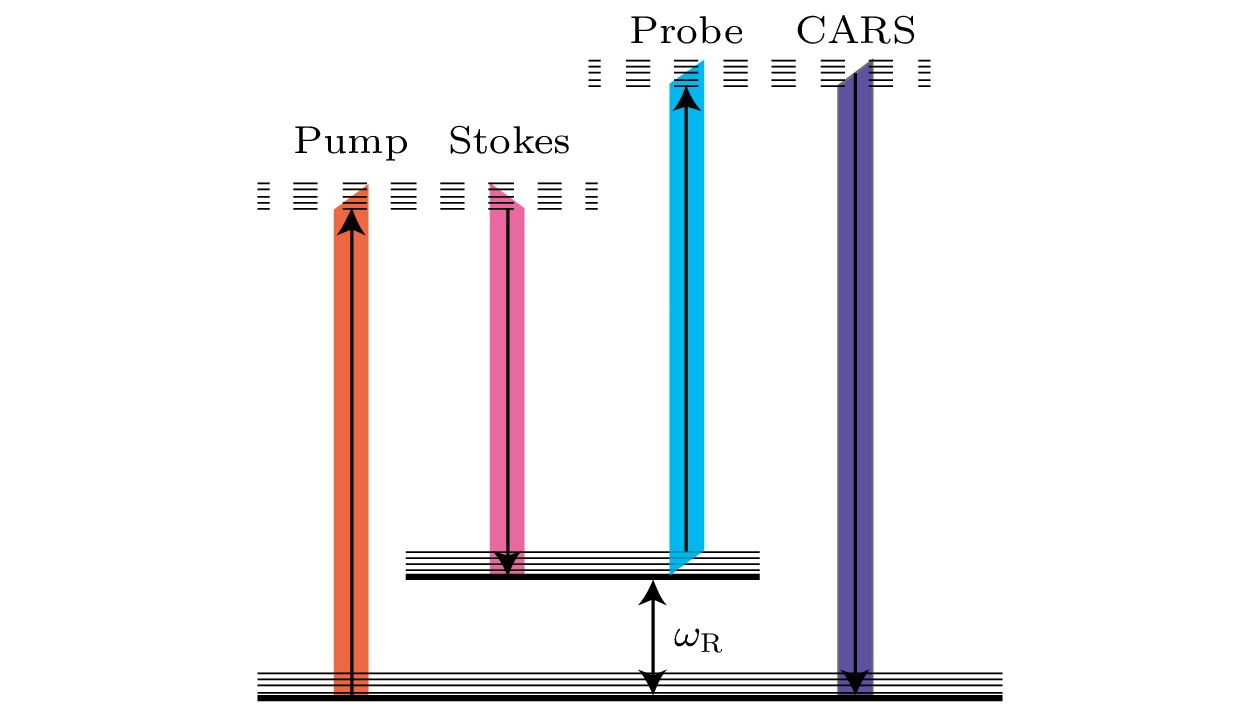
Figure1. Diagram of energy level transitions.
动量守恒条件的存在对CARS过程的空间相位匹配提出了严格的要求, 目前常见的CARS信号的空间相位匹配方式如图2(a)—(c)所示. 图2(a)为共线式相位匹配, 多用于生化样品分析或均匀气体中, 其空间分辨率较低, 不适用于燃烧场温度测量. 图2(b)为Eckbreth[27]提出的BOXCARS的相位匹配方式, 3束入射光与CARS信号以不同的角度在空间上分离, 从而获得了较高的空间分辨率. 在BOXCARS的基础上, 研究者进一步提出了折叠式BOXCARS的相位匹配方式[28], 如图2(c)所示, 通过将入射光与CARS信号的波矢分布在垂直于传播方向的两个平面内, 进一步提高了CARS过程的空间分辨率, 是目前CARS测温领域中最常用的相位匹配方式, 本文使用的相位匹配方式便是折叠式BOXCARS.
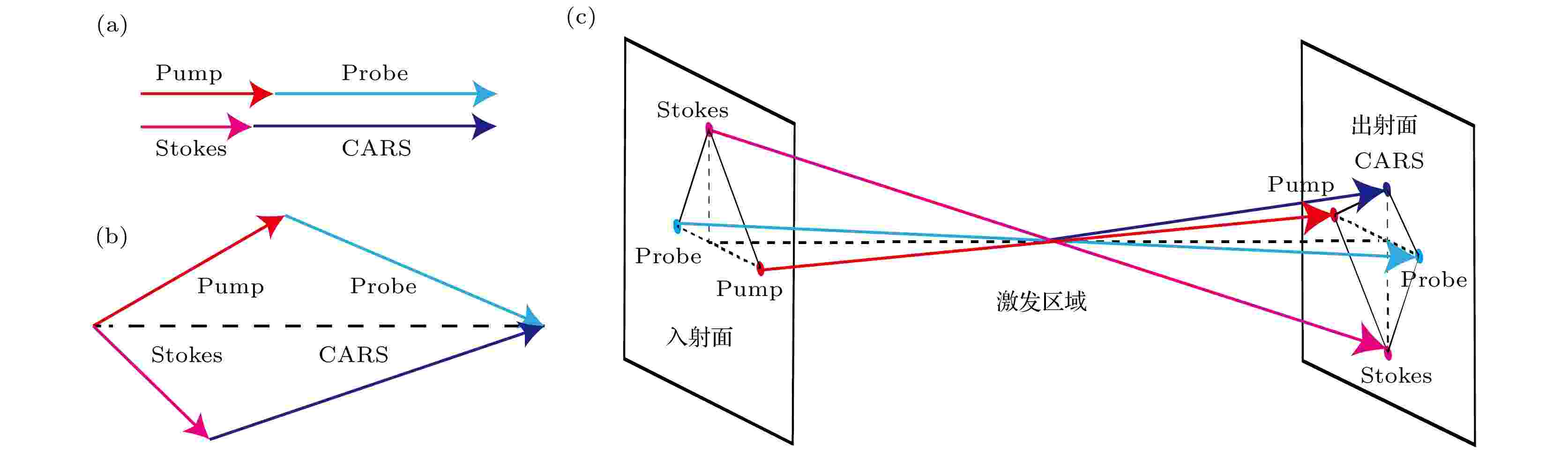
Figure2. (a) Diagram of collinear phase matching; (b) diagram of BOXCARS phase matching; (c) diagram of folded BOXCARS phase matching.
2
2.2.CARS理论模型
CARS信号的强度正比于三阶非线性极化率模的平方, 而三阶非线性极化率由共振和非共振两部分组成[29], 故CARS信号的强度可表示为
将计算得到的频率差、粒子数差、拉曼散射截面以及拉曼线宽代回分子响应函数的公式(3)中, 可以得到分子响应函数在时域的曲线, 而进一步在(2)式中, 将分子响应函数与泵浦光和斯托克斯光电场包络的乘积做卷积计算, 之后再乘上探测光电场包络, 可以得到分子的共振非线性极化率, 而时域CARS信号强度等于非线性极化率幅值的平方, 最后将时域CARS信号强度做傅里叶变换即可得到理论计算的CARS光谱.
本文选择氮气(N2)分子作为CARS探测的目标分子, 这是由于一方面N2分子在大部分燃烧环境中大量存在, 另一方面氮气分子的理论模型较为完善, 便于理论建模与温度拟合.
3.1.CARS光路系统
混合飞秒/皮秒CARS测温系统如图3所示, 飞秒激光器(Astrella, Coherent)作为整个系统的光源, 产生中心波长在795 nm, 重复频率为1 kHz, 脉宽约为35 fs的近似变换极限的高斯脉冲, 脉冲能量约为6 mJ. 入射光经过分光镜BS1后, 约50%的光发生反射, 泵浦OPA (OPerA Solo, Coherent)装置, 产生中心波长在670 nm的飞秒激光, 作为CARS过程的泵浦光. 经过第1个分光镜BS1后的透射部分, 经过分光镜BS2后再次被分为两路, 其中透射光部分作为CARS过程的斯托克斯光, 电动位移台1用于调节泵浦光和斯托克斯光在时域上的重合, 保证激发效率最大.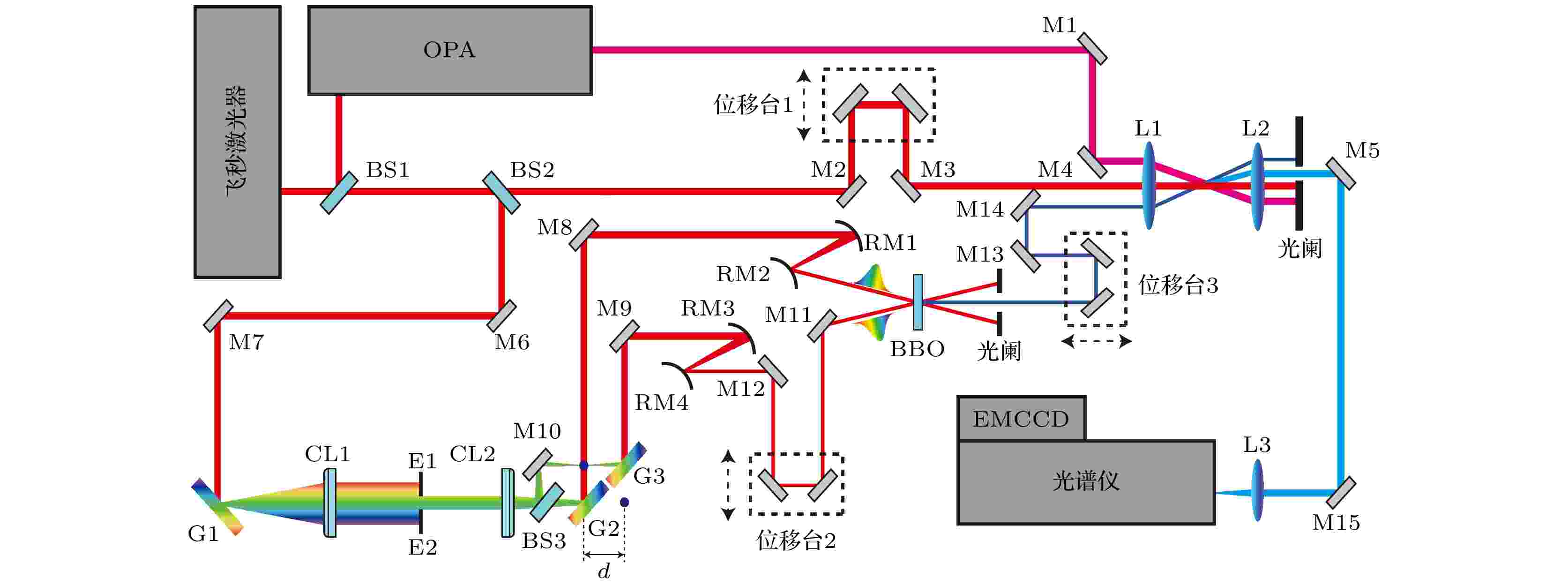
Figure3. Diagram of hybrid femtosecond/picosecond CARS optical system. BS1?BS3, beam splitter; M1?M15, mirror; G1?G3, grating; CL1 and CL2, cylindrical lens; RM1 and RM3, concave rear mirror; RM2 and RM4, convex rear mirror; E1 and E2, edge; L1?L3, lens; OPA, optical parametric amplifier; EMCCD, electron multiplying charge coupled device.
经过分光镜BS2后的反射光部分经过1个4f系统, 在柱面透镜CL2后被分光镜BS3分为两路, 两路光分别经过放置于柱面透镜CL2焦点前d距离和焦点后d距离位置的光栅G2和G3, 从而在两路光中分别引入相等的正负线性啁啾. 图3中蓝色圆点为柱面透镜CL2的焦点位置, 实验中d为45 mm, 柱面透镜CL1和CL2的焦距均为200 mm. 两路具有相等正负线性啁啾的光分别经过由1个凹面反射镜和1个凸面反射镜组成的缩束系统后, 以约3°的夹角入射BBO晶体中, 产生中心波长在约401.8 nm, 谱宽约为0.128 nm的窄带和频信号, 作为CARS过程的探测光, 探测光的脉冲能量约为120 μJ, 时域半高全宽约为4 ps. 电动位移台3用于调节探测光相对于激发光的时间延迟.
三束光经过透镜L1后, 按照2.1节中的折叠式BOXCARS方式进行相位匹配, 激发焦点处的N2分子, 从而产生波长约在367 nm附近的CARS信号, 在激发区域处泵浦光、斯托克斯光以及探测光的脉冲能量分别约为90, 60和120 μJ. 利用光阑在空间上将信号光分离, 信号光经过透镜L3聚焦在光谱仪(Andor, Shamrock)的狭缝上, 从而被EMCCD (Newton, Andor)接收. 实验中光谱仪狭缝宽度设为30 μm, 利用CCD的成像模式测量出聚焦点的空间尺寸约为64 μm, 验证了BOXCARS相位匹配方式高空间分辨率的特点.
2
3.2.标准燃烧系统
本实验所用的燃烧器为目前燃烧实验普遍使用的McKenna (Holthuis & Associates)燃烧器, 其实物图与燃烧火焰图如图4(a)和图4(b)所示. 燃烧系统使用甲烷和空气作为燃料, 氮气作为保护气, 示意图如图4(c)所示. McKenna燃烧器作为标准燃烧器, 在不同流速下的火焰温度都有公认的测量数据作为参考, 本文使用德国宇航局的测量数据[36]作为不同流速下的参考温度, 测量区域选择在燃烧器中心上方15 mm附近, 如图4(b)所示.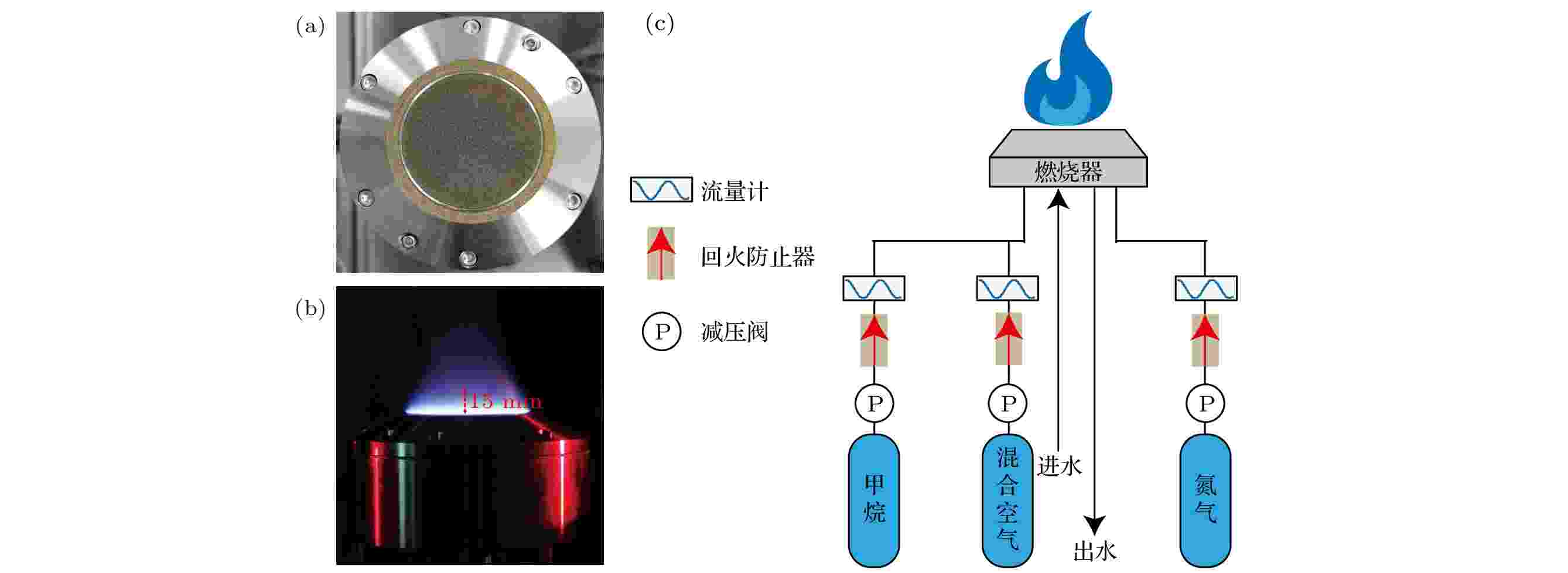
Figure4. (a) Image of burner; (b) image of flat flame; (c) diagram of combustion system.
为模拟实际应用环境下的高温动态燃烧场, 实验中选择了4个不同温度的甲烷/空气流速配比, 通过在某一时间快速改变甲烷和空气流量计的流速, 实现火焰温度的快速改变. 4个不同的流速配比及其对应的参考温度如表1所列.
流速/(标准升·min–1) | 参考温度/K | |
空气 | 甲烷 | |
15.00 | 1.10 | 1706 |
30.30 | 2.55 | 1967 |
15.00 | 1.42 | 1799 |
36.18 | 3.42 | 2110 |
表14种流速配比及其对应参考温度[36]
Table1.Four flow velocity ratios and their corresponding reference temperatures.
2
4.1.光谱拟合
通过将实际实验中的参数代入2.2节中的理论模型, 可获得符合实验条件的、不同温度下的理论光谱库, 理论光谱库的温度范围为300—2500 K, 温度间隔为1 K. 利用最小二乘法计算采集到的单幅光谱与理论光谱库中不同温度下的理论光谱的误差, 从而得到每个单幅光谱在不同温度下的拟合误差曲线, 理论拟合温度为单幅光谱拟合误差曲线最小值所对应的温度. 下面选择一幅光谱为例具体说明.该幅光谱原始光谱如图5(a)所示, 原始光谱信噪比约为2373.5, 高于此前报道的高温下的单幅信噪比[33], 侧面说明了本系统实现单脉冲稳定测量的能力, 这里信噪比是指光谱峰的最大值除以背景噪声的标准差. 对原始光谱进行处理, 减去背景噪声, 将波长转换为对应的拉曼频移, 就可以得到如图5(b)所示的归一化光谱. 按照上文所述, 利用最小二乘法, 计算2201幅理论光谱与处理后的归一化光谱的拟合误差, 误差曲线如图5(c)所示, 误差最小值约为0.05832, 对应温度为1705 K, 则该幅光谱的拟合温度便为1705 K. 实际光谱与误差最小温度下的理论光谱的对比如图5(d)所示, 可以看出, 实际光谱与理论光谱拉曼峰的位置与强度基本一一对应.
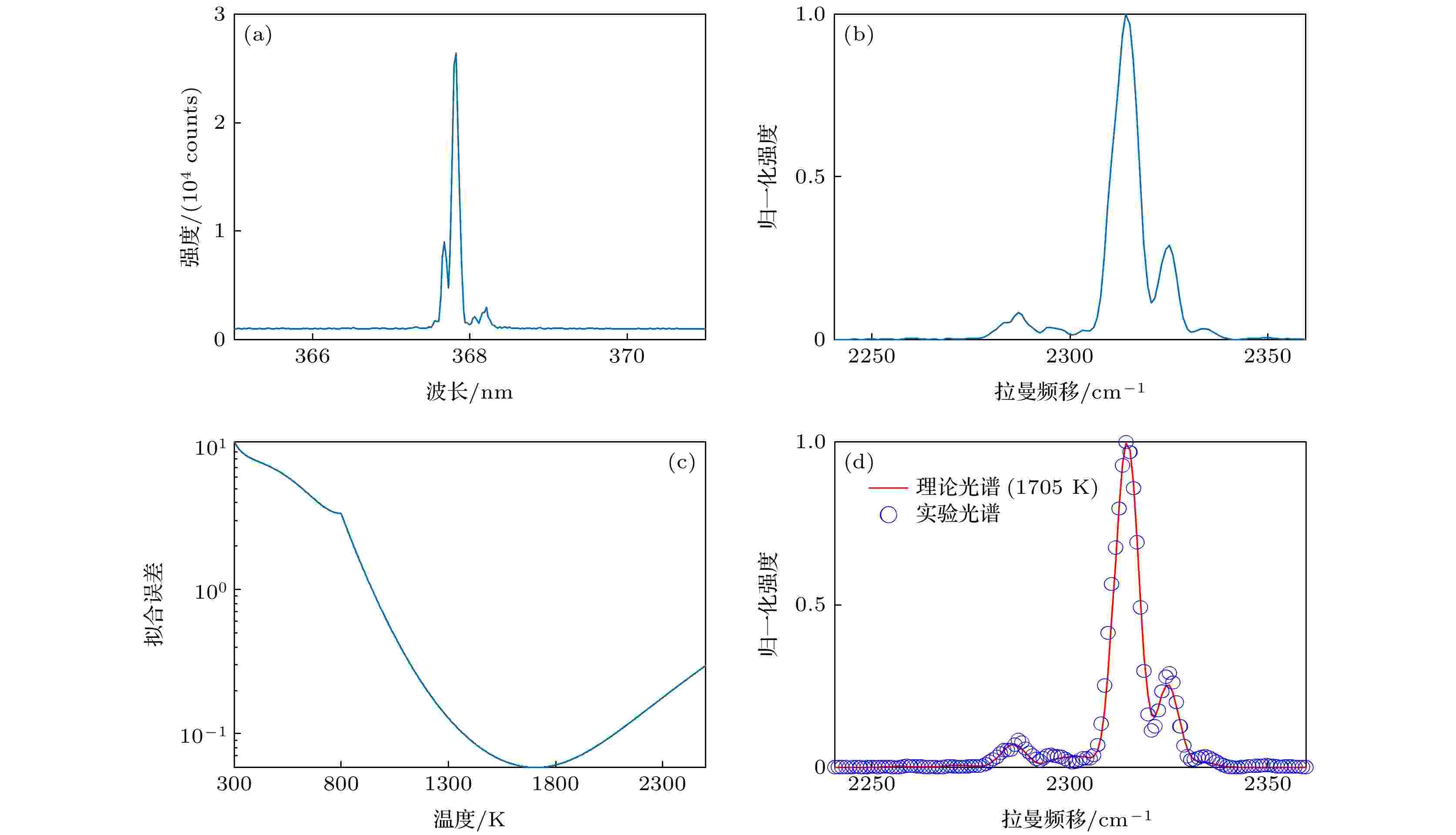
Figure5. (a) Original spectrum; (b) normalized spectrum after processing; (c) curve of fitting error; (d) comparison of fitting spectrum with actual spectrum.
2
4.2.动态结果分析
按照4.1节的步骤, 对70000幅光谱进行了分析, 得到70 s内连续测量结果如图6所示.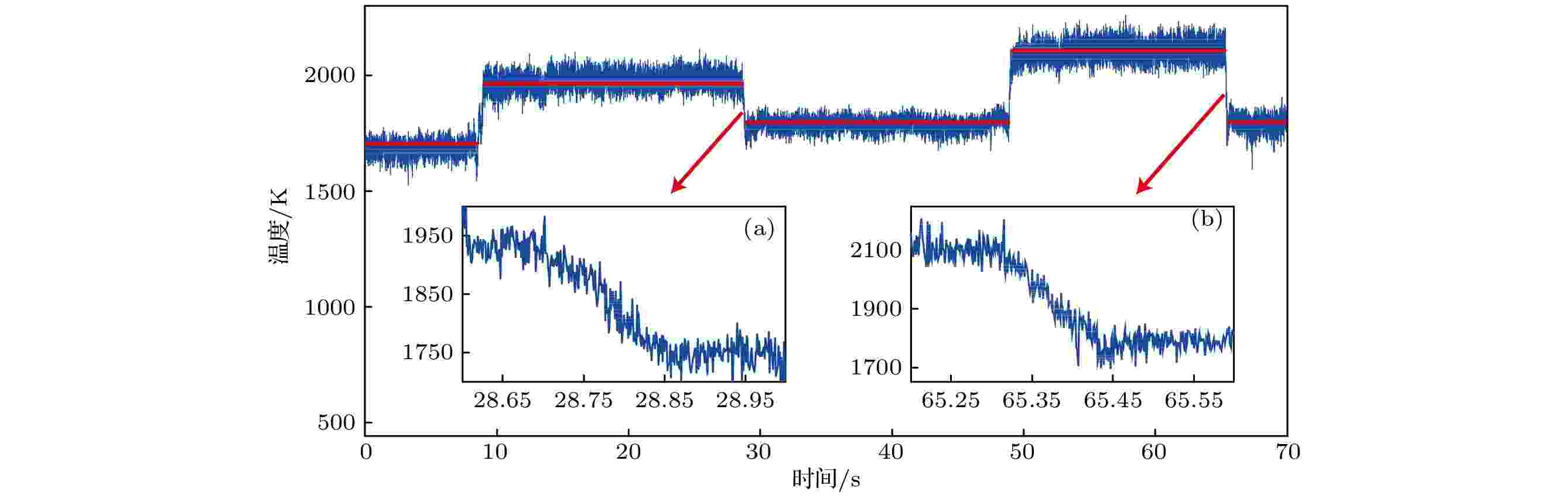
Figure6. Results of dynamic temperature measurement within 70 s: (a) Local enlarged between 28.6 s and 29 s; (b) local enlarged between 65.2 s and 65.6 s.
图6中各段的红色线条为该段流速配比所对应的参考温度, 可以明显地看出, 在约8.5 , 28.6, 48.8 和62.2 s时间位置处, 由于改变甲烷和空气的流速配比, 火焰温度发生了变化. 图6(a)和图6(b)分别为28.6—29 s以及65.2—65.6 s时间段温度变化趋势的局部放大图, 可以看出, 改变流量后温度变化过程发生在0.2 s内. 千赫兹采集到的单幅光谱的拟合温度准确描述了火焰温度递增与递减的改变过程, 与温度本身过程量的特征相符合, 验证了本系统对于动态变化的高温火焰的响应能力.
图6中前4个流速配比下单幅光谱的测量温度的分布直方图如图7所示, 图7中红色直线表示该流速配比的参考温度, 左上角为该流速配比下的参考温度与平均温度的具体数值.
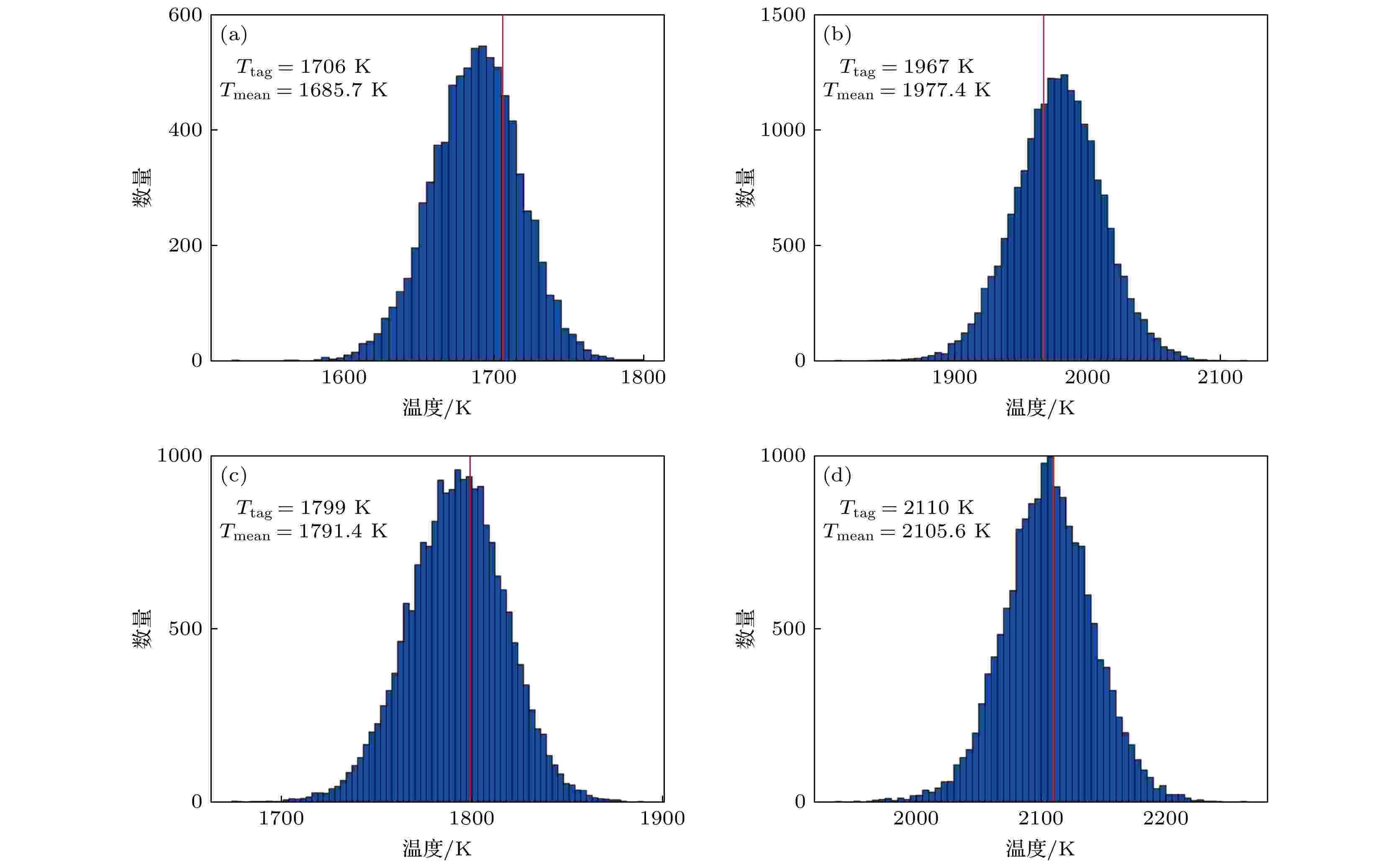
Figure7. Histograms of temperature measurements in different flow velocity ratios: (a) From 0 s to 8 s; (b) from 9 s to 28.5 s; (c) from 28.9 s to 48.8 s; (d) from 49 s to 65.3 s.
图7中Ttag为该流速配比下的参考温度, Tmean为平均温度, 可以看出, 4个流速配比下的单幅光谱的测量结果与平均温度均分布在参考温度附近, 说明高温下的测量结果较为准确稳定, 温度的抖动是由于高温下的热噪声以及火焰中折射率不均匀导致相位匹配的略微变化所产生的.
为进一步定量评价各段的测量精度, 计算了4个流速配比下温度测量的相对误差和相对标准偏差, 相对误差是利用该流速配比平均温度和参考温度的差的绝对值除以参考温度计算得到的, 而相对标准偏差是利用单幅光谱拟合温度的标准差除以平均温度计算得到的, 结果如表2所列.
时间段 | 参考温度/K | 相对误差 | 相对标准偏差 |
0—8 s | 1706 | 1.19% | 1.75% |
9—28.5 s | 1967 | 0.53% | 1.67% |
28.9—48.8 s | 1799 | 0.42% | 1.42% |
49—65.3 s | 2110 | 0.21% | 1.71% |
表24个流速配比下测温的误差分析
Table2.Error analysis of temperature measurement in four different flow velocity ratios.
4个流速配比下测温结果的相对误差均小于1.2 %, 相对标准偏差均小于1.8%, 说明单脉冲温度测量结果较为真实可靠, 验证了本系统在高温火焰中千赫兹、单脉冲温度测量的准确性与稳定性.
2
4.3.不同温度下光谱对比
为进一步说明混合飞秒/皮秒CARS测温的原理, 从4个流速配比下各选择1个单幅光谱, 对比其与最佳拟合的理论光谱, 结果如图8所示, 其中第1段所选择光谱与4.1节中选择光谱一致.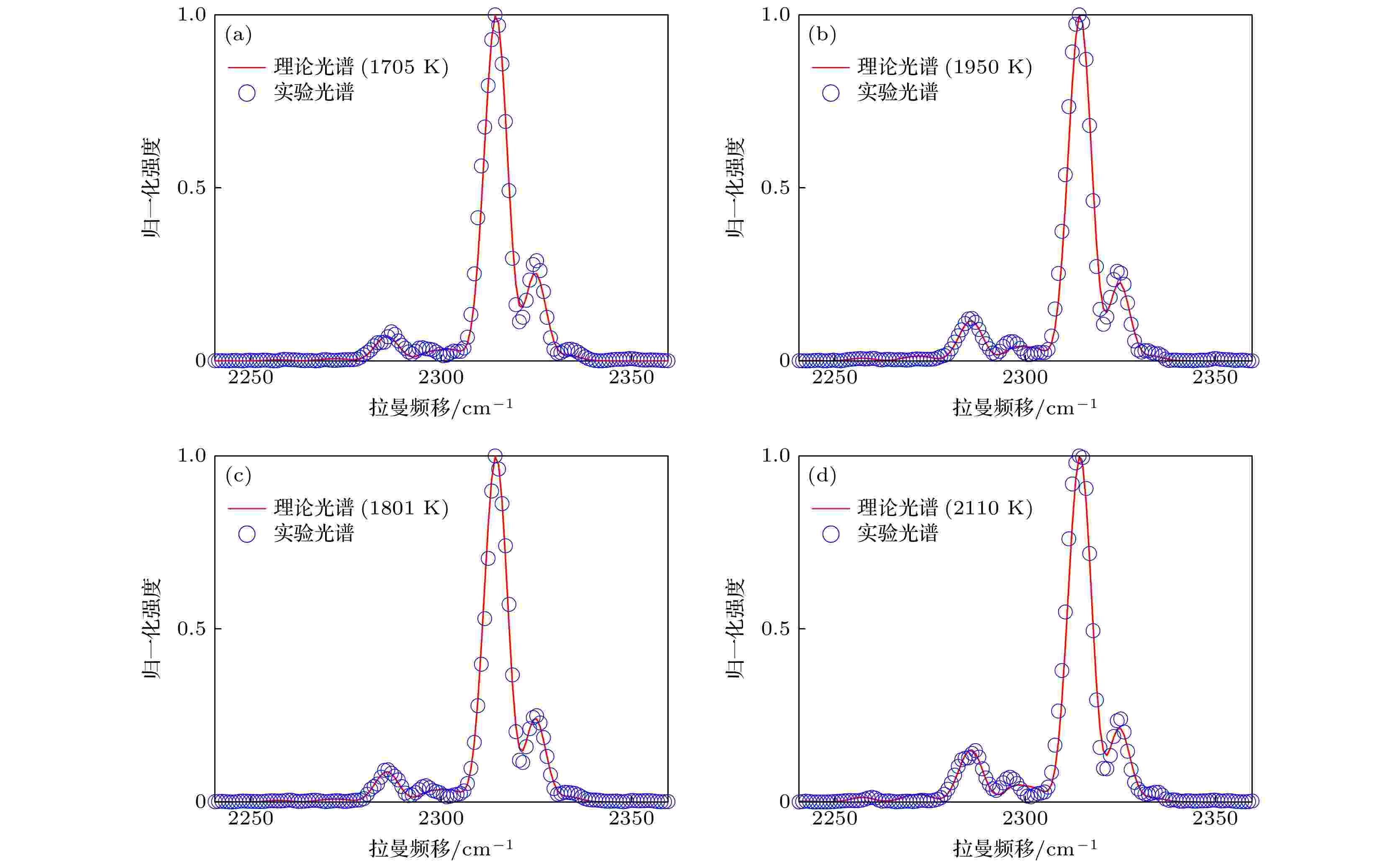
Figure8. Fitting results of single shot: (a) From 0 s to 8 s; (b) from 9 s to 28.5 s; (c) from 28.9 s to 48.8 s; (d) from 49 s to 65.3 s.
从图6可以看到, 随着温度的变化, CARS信号的拉曼峰的相对强度也发生了变化, 这是由于: 根据玻尔兹曼分布规律可以知道, 温度直接影响N2分子的粒子数分布, 而不同能级上粒子数分布的多少会直接影响该能级所对应的拉曼峰的强度, 如图8(d)和图8(a)对比, 温度升高后, 低能级粒子数减少, 高能级粒子数增加, 所以可以看到右侧拉曼峰的强度降低, 而左侧拉曼峰的强度升高, 通过拉曼峰的相对变化, 就可以准确分辨出温度的变化. 同时不同温度对应的N2分子的粒子数分布是各不相同的, 因此不同温度对应的N2分子的拉曼光谱具有唯一性.
实验中为了验证各阶段测温数据的真实可靠, 选择利用标准燃烧器模拟动态高温火焰, 所以受限于标准燃烧器的温度参考数据, 4个流速配比下的最小温度变化幅度为93 K, 而最终测量结果能够准确追踪0.2 s内的温度变化过程, 同时各阶段的测温结果较为准确稳定, 因此该方法具有推广到实际应用中进行动态高温火焰温度测量的潜力.