1.
Introduction
Silicon nitride (SiNx) has been widely used as the dielectric materials in the processing of optoelectronics and microelectronics semiconductor devices because of its excellent chemicophysics stability and superior optical properties such as high density, low leakage, anti-oxidation, high dielectric constant and remarkable masking ability[1, 2]. Most of the III–V compound semiconductors have a relatively high interfacial density, and the interface defects will produce the centers of interfacial recombination, which provide leakage channels and significantly deteriorate the electrical properties of the devices. Many studies have shown that SiNx is an outstanding candidate as a protection and passivation layer for III–V and other semiconductors[3–5]. For the mesa-type infrared detectors, the side-surface leakage current is one of the key components of the dark current, which reduces the detection limit of the devices. The effects of surface passivated SiNx deposition on the performances of InGaAs detectors have been investigated[5–6]. SiNx passivation has also been proved convincingly effective in reducing the surface recombination leakage current and improving the current gain of AlGaAs/GaAs and InP/InGaAs heterojunction bipolar transistors (HBTs)[7–10].
To deposit SiNx films, several kinds of technologies exist. Compared to conventional deposition technologies such as plasma enhanced chemical vapor deposition (PECVD) and low pressure chemical vapor deposition (LPCVD), inductively coupled plasma chemical vapor deposition (ICPCVD) shows many advantages, including higher plasma density, lower temperature, lower pressure, etc[1, 11]. On account of the high sensitivity to energetic ion bombardment and deposition temperature for III–V compound semiconductors, the use of conventional PECVD technology is usually disadvantageous due to the requirement of higher deposition temperature (250–400 °C)[1] and the presence of intensive ion bombardment. Chou et al.[12] have developed the SiNx passivation technique at a lower-temperature using high-density ICPCVD, which utilizes inductively coupled plasma to create high-density plasma (around 1012 cm–3) in the processing reactor. High density plasma allows the deposition of high quality SiNx at lower temperatures below 150 ℃[13]. Furthermore, the separation between deposition area and plasma generation area can reduce the surface damage deriving from energetic ion bombardment. Shi et al.[5, 14] has comparably studied the mesa-type n-on-p In0.83Ga0.17As photodiodes passivated by PECVD and ICPCVD deposited SiNx. They found the photodiodes passivated using ICPCVD show significantly reduced perimeter-dependent surface leakage current in contrast to those using PECVD. In recent years, the ICPCVD processing has been extensively investigated, while the effects of deposition temperature, ICP power, and chamber pressure on the quality of ICPCVD SiNx films have been reported[11]. However, due to the complicated deposition process, the investigations on the ICPCVD processing parameters and the optical properties of SiNx films are still insufficient. In this work, we have investigated the effects of the flow rate of ammonia (NH3) on the surface and optical properties of SiNx films deposited by ICPCVD. The composition, growth rate and chemical bonding of the deposited films were characterized in detail.
2.
Experimental details
The SiNx films were deposited on InP substrates using the SENTECH SI500D ICPCVD system. Before deposition, the InP substrates were rinsed with dilute hydrochloric acid for 5 s to remove the surface oxide layer, then washed with deionized water, dried with nitrogen and loaded into the deposition chamber immediately to keep a fresh surface. The SiNx films were deposited at 130 °C for 15 min. During the deposition, the radio frequency (RF) power was 350 W, the flow rates of silane (SiH4) and Ar were 135 and 145 sccm, respectively. To investigate the effects of NH3, four samples with different flow rates of NH3 from 3 to 12 sccm were deposited, and the detailed flow rates of NH3 for various samples are listed in Table 1.
After deposition, the surface morphology and roughness of the SiNx films were measured by using Multimode 8 atomic force microscopy (AFM) in tapping mode. The cross-section morphology and thickness of the SiNx films were characterized by a JEOL 7800F scanning electron microscope (SEM). The etching rates of the SiNx films were also characterized by using a buffered oxide etchant (BOE) solution which consisted of HF and NH4F in the ratio of 1 : 6. The optical properties of the deposited films were investigated using an X-ray photoelectron spectroscopy (XPS) THERMO's ESCALAB 250 spectrometer using an Al Kα source. The Raman spectra were measured by a HORIBA Jobin Yvon HR 800 spectrometer, where a 514 nm argon ion laser with 3 mV output power was used as the excitation light source, and the light was converged onto the surface of the sample through a lens with the focused laser beam spot of about 1 μm.
3.
Results and discussion
AFM was carried out to characterize the surface morphologies of the SiNx samples, and Fig. 1 shows the AFM images with a 5 × 5 μm2 scanning area. The RMS roughness values are extracted and indicated. As the NH3 flow rate increases from 3 to 6 sccm, the RMS roughness decreases from 1.53 to 0.74 nm. As the NH3 flow rate further increases to 12 sccm, the RMS value increases to 1.29 nm.

class="figure_img" id="Figure1"/>
Download
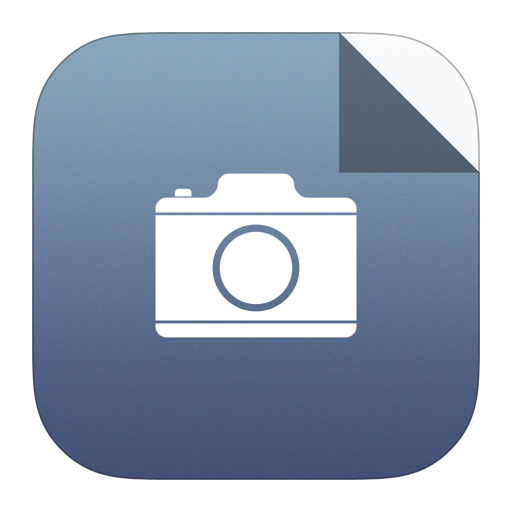
Larger image
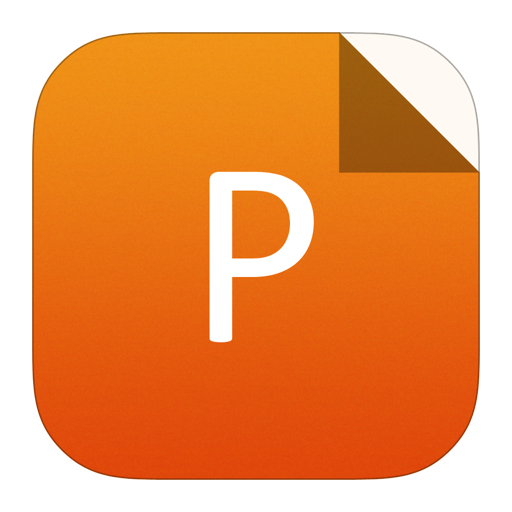
PowerPoint slide
Figure1.
(Color online) AFM images of the SiNx samples with NH3 flow rate of (a) 3 sccm, (b) 6 sccm, (c) 9.5 sccm, and (d) 12 sccm, respectively (scanning area: 5 × 5 μm2).
The SiNx samples were characterized by cross-section SEM, and the typical image is shown in Fig. 2(a). In the image, the clear and smooth interface between the SiNx film and the substrate is observed. The cross-section image also shows a dense surface and homogeneous film thickness. The film thicknesses and deposition rates are then extracted from the SEM images. Fig. 2(b) shows the RMS surface roughness and deposition rate as functions of the NH3 flow rate. The film grown with NH3 flow rate of 6 sccm has the lowest deposition rate of 11.3 nm/min. The deposition rate is increased to 16.4 nm/min for the NH3 flow rate of 3 sccm. As the reactants have a very low content of NH3, the deposition of amorphous silicon (α-Si) is the dominant process, causing a larger deposition rate. As the NH3 flow rate increases to 9.5 and 12 sccm, the deposition rate is increased to 17.4 and 18.8 nm/min, respectively. The increase of NH3 flow rate leads to more H atoms involved in bonding to form Si–H bonds and N–H bonds, making the SiNx film become loose and grow faster. Generally, a dense dielectric film is preferred for the passivation layer of the III–V semiconductor to reduce the surface recombination effects. Therefore, a moderate deposition rate of SiNx film is needed in the ICPCVD process.

class="figure_img" id="Figure2"/>
Download
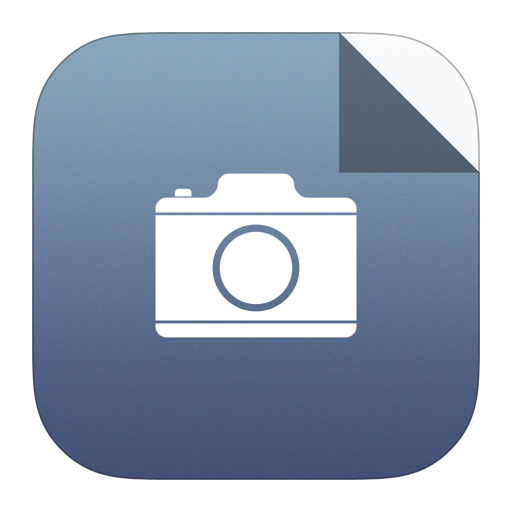
Larger image
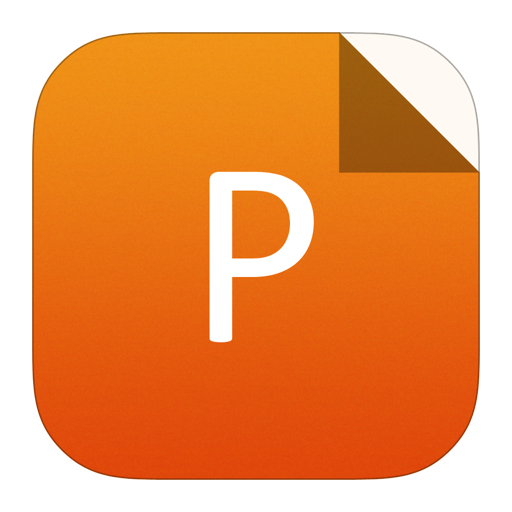
PowerPoint slide
Figure2.
(Color online) (a) Typical cross-section SEM image of the SiNx samples (sample 1). (b) RMS and deposition rate of SiNx samples as functions of NH3 flow rate.
It is also observed from Fig. 2(b) that the surface roughness and deposition rate show a quite consistent trend. The RMS roughness is increased for the samples with larger deposition rates. In the process of film deposition, the surface adsorption atoms are buried by the subsequent atoms and become a “bulk atom”, these “bulk atoms” usually are not able to diffuse again. When the deposition proceeds slowly, the atom can be fully diffused and occupy the lower energy position before it becomes a “bulk atom”, so that the free energy of the film is minimal, and the film becomes smoother and less defective. On the contrary, as the deposition rate becomes too large, once the atoms do not have enough time to sufficiently diffuse, they will be covered by newly deposited atoms and become “bulk atoms”, these atoms are essentially in the initial deposition site and not the lowest energy position. This kind of process results in the film surface with more three-dimensional islands or column crystals and causes more rough surfaces.
To further characterize the structural properties, the SiNx samples were etched using BOE solution and the etching rates were measured and listed in Table 1. The etching rate is almost zero for the film with NH3 flow rate of 3 sccm. The α-Si is dominant in the sample when the NH3 flow rate is low, while the Si-rich SiNx:H with many Si-Si bonds have a very slow corrosion rate in HF solution. In the Si-rich SiNx:H samples, many free Si atoms fill in the interspace of the Si-N network. This will increase the compactness of the SiNx samples and cause a smaller corrosion rate in the BOE solution. When the NH3 flow rate is increased, more H atoms are expected to be introduced in the film. Extra H atoms incorporated in the hydrogen-rich silicon nitride layer are Si3N4 network breakers, which also reduce the density of the layer. Both a larger amount of H and lower layer density are tending to increase the etching rate in the HF solution[13, 16]. The BOE etching rate gradually increases from 0 for sample 1 to 35 nm/min for sample 3 as the NH3 flow rate rises from 3 to 9.5 sccm, whereas it significantly increases from 35 to 230 nm/min as the NH3 flow rate is further increased to 12 sccm for sample 4. This implies that many extra H atoms exist and the layer density is remarkably decreased in sample 4. When the NH3 flow rate is between 6 and 9.5 sccm, the SiNx films exhibit nice compactness with moderate etching rates.
The optical properties of the SiNx samples were investigated by XPS and Raman measurements. The XPS measurements were carried out for the as-grown samples and those after being etched by Ar+ for 30 s. Fig. 3(a) and Fig. 3(b) show the XPS scanning spectra of sample 3 before and after Ar+ etching while other samples show similar spectra. The obvious peaks of O 1s, N 1s, C 1s, Si 2p and Si 2s core levels are observed in the XPS spectra and the elemental concentrations are estimated from the XPS measurements. Fig. 4 shows Si, C, N and O elemental concentrations in the SiNx samples as functions of the NH3 flow rate. For the as-grown samples, some O and C can be observed, which become negligible in the XPS measurements after Ar+ etching. The O and C elements are probably due to the contamination of atmospheric oxidation. The Si/N ratio has been calculated from the Si and N elemental concentrations in the XPS measurements after Ar+ etching and are listed in Table 1. As shown in Fig. 4(b) and Table 1, the Si concentration reduces and the N concentration increases as the NH3 flow rate increases. The Si/N ratio is decreased from 11.3 for sample 1 to 0.9 for sample 4. Theoretically, the Si/N ratio is 0.75 for standard Si3N4. The deviation of the measured Si/N ratio to the theoretical one is probably due to the relatively large measurement error of the absolute elemental concentration in XPS. Nevertheless, the relative trend of the Si/N ratio for the samples with different NH3 flow rates is obvious.

class="figure_img" id="Figure3"/>
Download
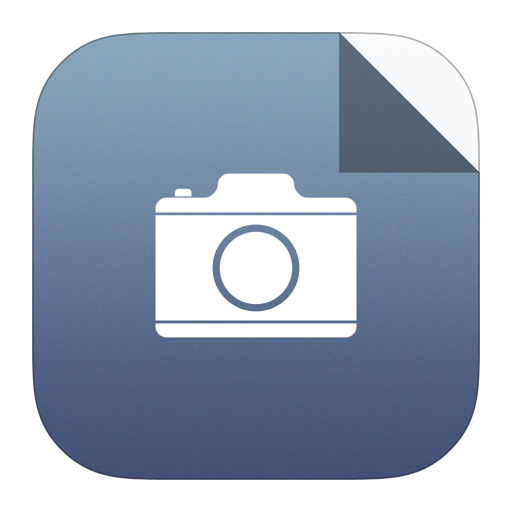
Larger image
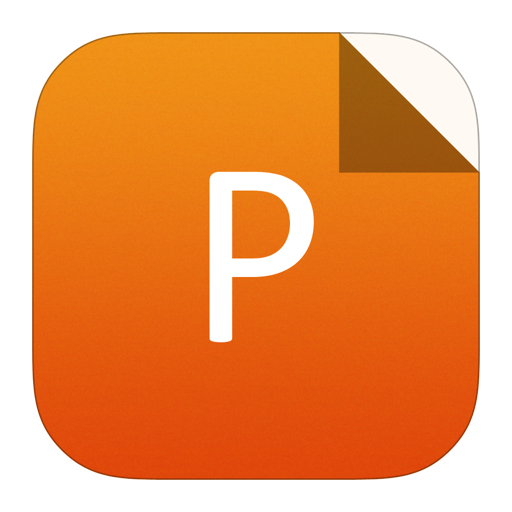
PowerPoint slide
Figure3.
(Color online) XPS spectra of sample 3 (a) as-grown, (b) after Ar+ etching of 30 s.

class="figure_img" id="Figure4"/>
Download
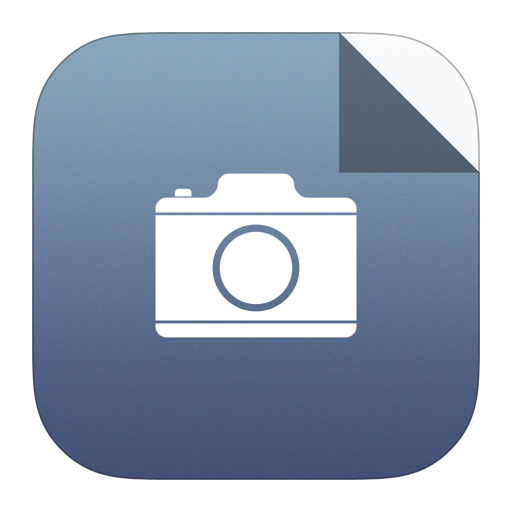
Larger image
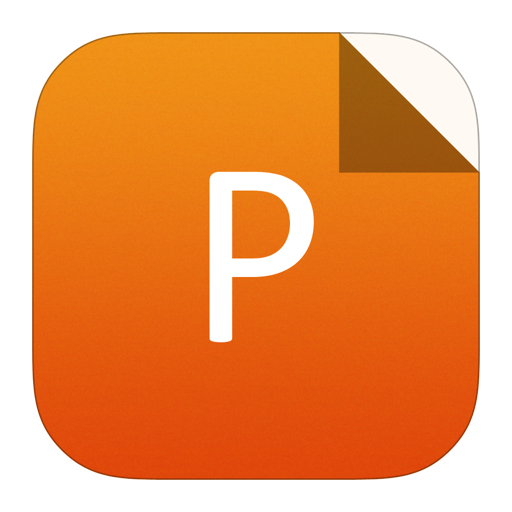
PowerPoint slide
Figure4.
(Color online) The concentration of Si, N, C and O in the SiNx samples measured by XPS as functions of NH3 flow rate (a) as-grown and (b) after Ar+ etching of 30 s.
Sample No. | NH3 flow rate (sccm) | BOE etching rate (nm/min) | Si/N ratio |
Sample 1 | 3.0 | 0 | 11.3 |
Sample 2 | 6.0 | 10?20 | 1.5 |
Sample 3 | 9.5 | 35 | 1.0 |
Sample 4 | 12.0 | 230 | 0.9 |
Table1.
The etching rate and Si/N ratio of SiNx samples with different NH3 flow rates.
Table options
-->
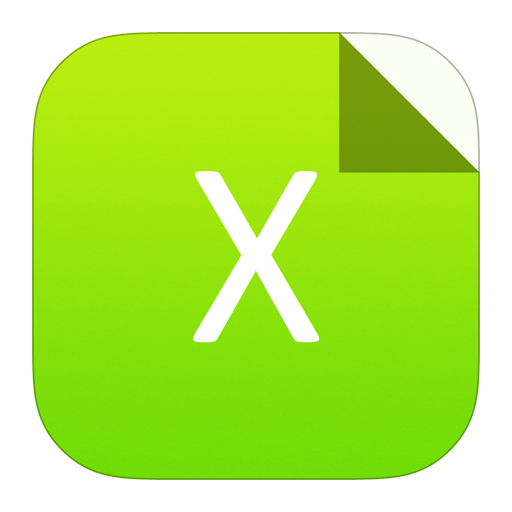
Download as CSV
Sample No. | NH3 flow rate (sccm) | BOE etching rate (nm/min) | Si/N ratio |
Sample 1 | 3.0 | 0 | 11.3 |
Sample 2 | 6.0 | 10?20 | 1.5 |
Sample 3 | 9.5 | 35 | 1.0 |
Sample 4 | 12.0 | 230 | 0.9 |
In the XPS spectra, the different binding energies correspond to different bonding states. Karcher et al.[17] have shown that there are five different forms of Si and N bonding states, Si, Si3N, SiN2, SiN and Si3N4, which can be expressed as Si0, Si+1, Si+2, Si+3 and Si+4, respectively. The binding energy of 102.0 eV corresponds to Si+4, and the 99.6 eV corresponds to Si0, the binding energies between them correspond to the bonding states of Si0 to Si+4[18]. Fig. 5 presents the change of Si 2p and N 1s core-level spectra in the four samples. It can be seen that the Si 2p core-level binding energy is at 99.7 eV for sample 1, close to the bonding state of Si0, which indicates the main components of α-Si in sample 1. The Si 2p binding energy shifts to about 101.5 eV as the NH3 flow rate increases to 6 sccm, and keeps around 102.0 eV for higher NH3 flow rates, indicating the bonding state of Si+4 for samples 2?4. In the α-Si crystal structure, each Si atom bonds with the other four nearest Si atoms. As NH3 is added continuously, an increasing number of the homopolar Si–Si bonds will gradually be replaced by heteropolar Si–N bonds. The charge transferring from Si atom to the more electronegative N atom leaves a positive charge on the original Si atoms, which results in the Si core levels shifting towards higher binding energy[17]. On the other hand, the N 1s core-level binding energy stabilizes at around 397.4?397.9 eV for the four SiNx samples with various NH3 flow rates, indicating that there is no significant change in the state of the N element: the N atoms mainly bond with Si atoms and form SiNx.

class="figure_img" id="Figure5"/>
Download
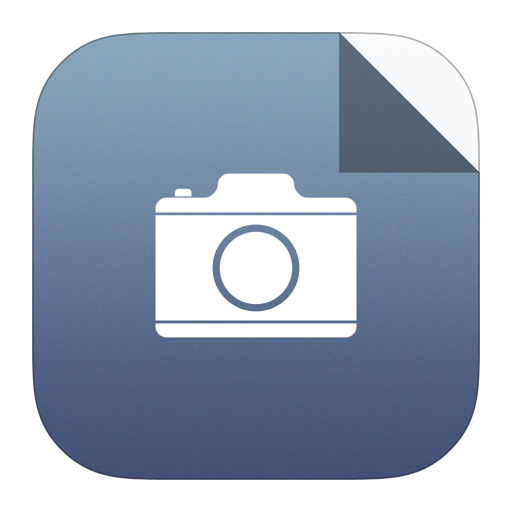
Larger image
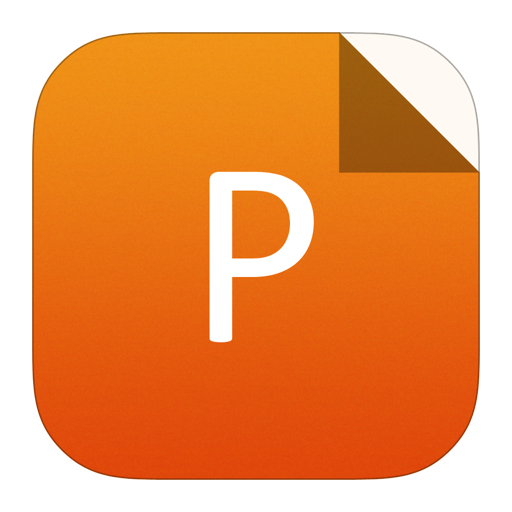
PowerPoint slide
Figure5.
(Color online) XPS spectra of Si 2p and N 1s core levels for the samples with NH3 flow rate of (a) 3 sccm, (b) 6 sccm, (c) 9.5 sccm, and (d) 12 sccm, respectively.
Fig. 6 shows the Raman spectra of the SiNx samples. It is observed that samples 1 and 2 show four obvious regions, which represent the Raman spectral characteristics of the α-Si, as follows. The Raman frequencies around 100?180 and 420?550 cm?1 correspond to the transverse acoustic (TA) and transverse optical models of α-Si, respectively. The frequencies around 580?700 cm?1 and 850?1050 cm?1 probably correspond to the twofold longitudinal acoustic (2LA) and twofold transverse optical (2TO) models of α-Si[19, 20]. The Raman frequency at 355 cm?1 is the typical LO model of InP, which comes from InP substrate. As the NH3 flow rate increases, the Raman peaks of α-Si decrease significantly and become quite weak for samples 3 and 4. The Raman results also indicate the main components of α-Si for the SiNx samples deposited with low NH3 flow rates and the decreased α-Si content for larger NH3 flow rates.

class="figure_img" id="Figure6"/>
Download
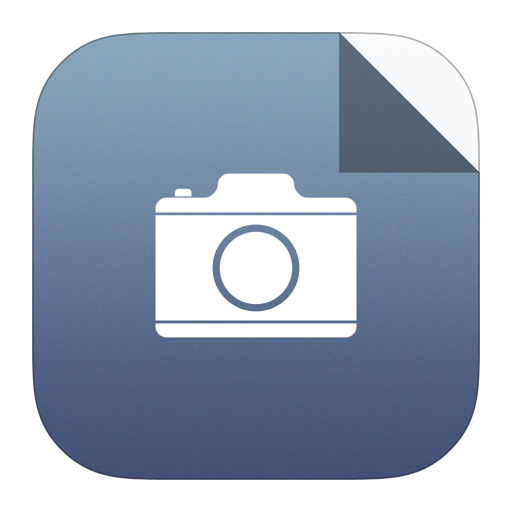
Larger image
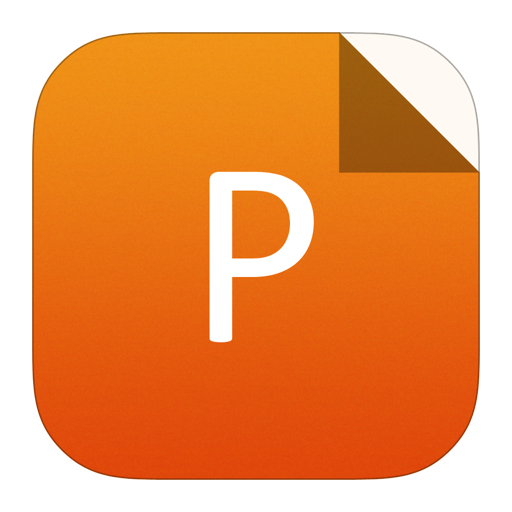
PowerPoint slide
Figure6.
(Color online) Raman spectra of various samples with NH3 flow rate of 3 sccm (Sample 1), 6 sccm (Sample 2), 9.5 sccm (Sample 3) and 12 sccm (Sample 4), respectively.
4.
Conclusion
In conclusion, the effects of ICPCVD NH3 flow rate on the surface and optical properties of SiNx samples have been investigated. XPS and Raman measurements have revealed that α-Si is dominant in the samples with low NH3 flow rates and the α-Si content is decreased for larger NH3 flow rates. AFM and SEM results show that low NH3 flow rates induce the increased surface roughness because of the dominant α-Si, whereas high NH3 flow rates also enhance the surface roughness due to the higher deposition rate. Moreover, the bonding states of the Si and N atoms are investigated by using XPS analysis, which reveals that the Si atoms transfer from Si0 to Si+4 with the increased NH3 flow rate.