1.
Introduction
Skin is the largest organ featuring soft mechanical properties and a complex sensory network that interfaces with the environment, which serves as an inspiration for the development of next-generation flexible electronics[1, 2]. An increasing number of activities have been devoted to skin-inspired electronics to mimic the properties and functions of natural skins, which represents an exciting research frontier with potential applications in advanced healthcare, human–machine interface, and soft robots/prostheses[3–5].
To this end, skin-like properties including mechanical stretchability, self-healing capability, and biodegradability have been incorporated into new classes of electronic materials[6]. Inspired by the structural designs and working mechanisms of living tissue and organism, various biomimetic sensors have been developed to realize comparable sensory functions[7, 8]. Further system-level optimizations of skin-inspired electronics in terms of wireless data transmission, large-scale data collection, and closed-loop networks, integrate intelligent functionalities for a broad range of practical applications[9–11].
In this review, we focus on recent progress of skin-inspired electronics including material selection, device design, and system improvement. We summarize advances, with particular emphasis on semiconductor materials and devices. Several strategies for biomimetic sensors, and advanced system-level functional improvement along with applications in robots/prostheses, human-machine interfaces, and healthcare monitoring are discussed. We conclude with the remaining challenges and potential future directions of skin-inspired electronics.
2.
Semiconducting materials with skin-like properties
Skin-like properties, including stretchability, self-healing capability, biocompatibility, and biodegradability, have been demonstrated in a new class of semiconductor materials to enable their wide application in skin-inspired electronics. These skin-like features are primarily based on new material concepts or innovative engineering approaches.
2.1
Stretchability
Typical biological skin has a modulus of 140–600 kPa and a maximal stretchability up to 75% tensile strain[12, 13]. The deformability of skin enables the free movement of our body while retaining excellent functional capabilities. Conferring stretchability to electronics can extend their applications to various environments, such as curved surfaces[3, 4, 14].
Strain engineering is a facile approach to enable stretchability in conventionally rigid and brittle materials. Inspired by wrinkles and creases of natural skin, geometry designs like kirigami, serpentine, buckling, and microcracks are utilized to create stretchable semiconducting materials[15–20]. Shyu et al. proved that stiff sheets acquired ultrahigh extensibility after top–down microscale kirigami patterning with strain tolerances up to 370%[17]. Cheng et al. reported a flexible pressure sensor based on silicon nanowires (SiNWs), which become deformable nanocrystals as compared with bulk silicon[21]. However, these approaches may be incompatible with planar device designs and high-density integrated circuits due to its complicated and expensive fabrication[22, 23].
Intrinsically stretchable semiconducting materials can be stretched without degradation in their electronic performances, which is attractive for skin-inspired electronics to achieve stable and robust performances. Polymers are among the typical examples of stretchable semiconducting materials formed through standard solution processes[24, 25]. Various energy dissipation mechanisms are introduced to the normally rigid and non-stretchable conjugated polymers, such as introducing dynamic breakable bonds and increasing disorder in the polymer morphology. Side-chain crosslinking is another approach which can be easily modulated to minimize the disruption of charge transport[26]. According to the recent report by Oh et al., 2,6-pyridine dicarboxamide (PDCA) was introduced as strain-releasing moieties to 3,6-di(thiophen-2-yl)-2,5-dihydropyrrolo[3,4-c]pyrrole-1,4-dione (DPP) polymer, which resulted in weak hydrogen bonds and reduced rigidity of the polymer backbone (Fig. 1(a))[27]. The DPP polymer with PDCA at a concentration of 10% can be stretched up to 100%, which allows the realization of a stretchable thin-film field-effect transistor (TFT) with relatively high mobility (> 1 cm2 V?1 s?1) maintained under stretched states with 100% strain. The introduction of PDCA units also partially breaks the conjugation and lowers the polymer backbone rigidity. This gives another mechanism for improvement of stretchability. Nano-confinement is effective in altering the physical properties of polymers, which has been reported to reduce the polymer glass transition temperature, the mechanical modulus and increase fracture strain[28]. Xu et al. developed a stretchable semiconducting material by introducing nanofibrils to a soft and deformable elastomer (polystyrene-block-poly(ethylene-ranbutylene)-block-polystyrene (SEBS)) (Fig. 1(b)). The high stretchability was attributed to the improved polymer chain dynamics and suppressed crystallization due to nano-confinement and embedding of the polymer network in an elastic matrix[29].

class="figure_img" id="Figure1"/>
Download
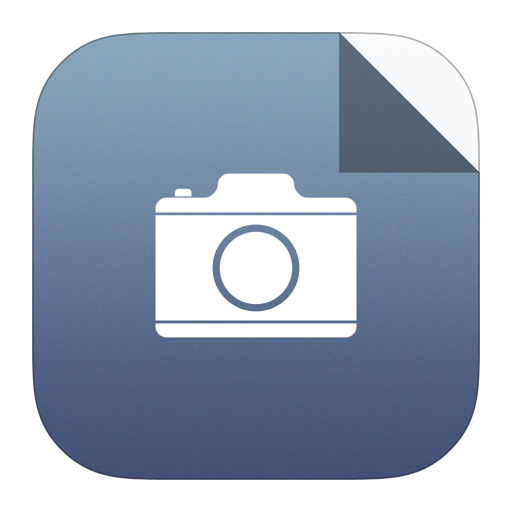
Larger image
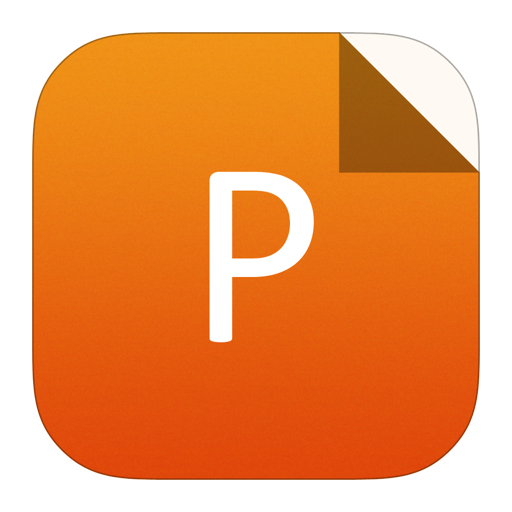
PowerPoint slide
Figure1.
(Color online) (a) Chemical structures of semiconducting polymers and mechanism for improved stretchability via dynamic bonding. (b) Schematic illustration of the embedded nanoscale polymer networks (top) and chemical structures of semiconducting polymer and elastomer (bottom). Panel (a) adapted with permission from Ref. [27]. Copyright 2016, Springer Nature. Panel (b) adapted with permission from Ref. [29]. Copyright 2017, American Association for the Advancement of Science.
Blending of functional nanomaterials into elastomers allows facile fabrication of stretchable composites with electrical conductivity and stable cycling[30–32]. Song et al. embedded the one-dimensional (1D) poly(3-hexylthiophene) (P3HT) nanowires into poly(dimethylsiloxane) (PDMS), which maintain the percolated networks under stretching due to their easy rotation and align along the stretching direction[33]. The FET based on this stretchable, transparent material showed excellent mechanical and electrical stabilities at strains of 100%. This method is generally applicable to other semiconducting materials (e.g., carbon nanotube (CNT) and graphene) as a new way to create stretchable nanocomposites and devices[34–38].
2.2
Self-healing
Self-healing capability is a unique feature of natural skin to enable autonomous self-repair after damage[39]. Electronic materials with self-healing abilities allow damaged devices to recover the initial connectivity and functions, thereby extending the lifespan and reducing maintenance expenses[40, 41].
Generally, self-healing capabilities are achieved through reversible bonds (e.g., hydrogen bonding or dynamic covalent bonding), dynamic interactions (e.g., host–guest interactions, π–π interactions, ionic interactions, and electrostatic interactions), and microcapsule-based composite with embedded self-healing agents[42–44]. Dynamic bonds and interactions are favorable for reversible self-healing processes, while the self-healing agent-based mechanism is limited to a single or a few occurrences of damage. For semiconducting materials, polymers have demonstrated great potential in developing self-healability through molecular design, which is understandable as most natural biological organs and systems are also polymeric[45, 46]. In addition, self-healing polymeric materials dissipate energy through reversible bond formation/rupture, which allows enhanced stretchability and high strain tolerance. Inspired by jellyfish, Cao et al. demonstrated a transparent and stretchable electronic skin sensor with self-healing capabilities (Fig. 2(a))[47]. Ionic species were incorporated into an amorphous polymer to introduce ion–dipole interactions, which favors the fast and repeatable self-healing behavior in aquatic conditions. According to the recent study by Oh et al., thermal and solvent annealing can heal the nanocracks by fatigue in DPP polymer with excellent recovery of the charge carrier mobility (Fig. 2(b))[27]. The result exemplifies complete recovery in conjugated polymer with high charge carrier mobility.

class="figure_img" id="Figure2"/>
Download
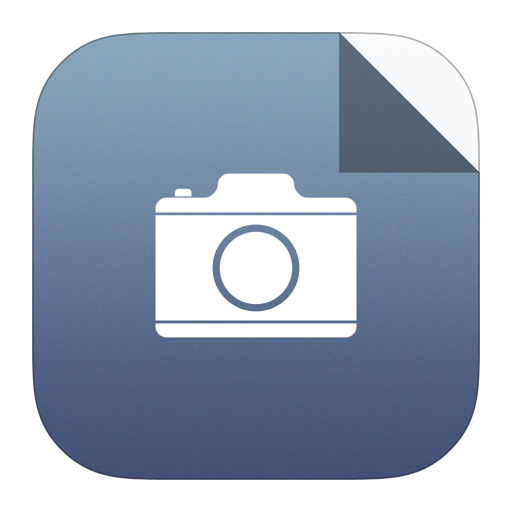
Larger image
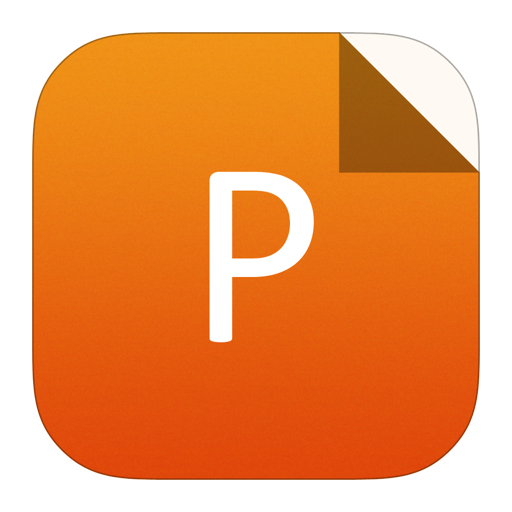
PowerPoint slide
Figure2.
(Color online) (a) Schematic illustration of the self-healing process of the polymer through reversible ion–dipole interactions. (b) Schematic illustration of the healing process of the treated polymer films (left) and transfer curves and mobility of the damaged and healed OTFTs (right). (c) Schematic of the self-healing composite of polymer networks and micro-nickel particles. Panel (a) adapted with permission from Ref. [47]. Copyright 2019, Springer Nature. Panel (b) adapted with permission from Ref. [27]. Copyright 2016, Springer Nature. Panel (c) adapted with permission from Ref. [53]. Copyright 2012, Springer Nature.
To realize fully self-healable devices, functional components with autonomous healing capabilities without any external stimuli are expected to be developed concurrently[48]. For example, several self-healable polymer-based sensors and light-emitting displays have been developed[49–51]. Yan et al. designed supramolecular polymer materials consisting of soft polymeric chains and strong quadruple H-bonding cross-linkers, and employed them in self-healing electromyogram (EMG) sensors[52]. Tee et al. reported a room-temperature self-healing sensor based on a supramolecular composite with embedded nickel microparticles (Fig. 2(c))[53]. The conductivity reached 40 S/cm with a short healing time of 15 s. The piezoresistive composite material resembles the skin with self-healing capability and sensitivity to tactile/flexion forces. Khatib et al. employed all self-healing components to create a multifunctional FET[54]. Poly(urethane) (PUU) was adopted as the substrate and dielectric material that utilizes the reversible hydrogen and disulfide bonds to achieve autonomous recovery in the absence of any external stimuli. CNTs were drop-cast onto the PUU surface as the semiconductor material. All the components showed high self-healing efficiency (98%) and recovery of transistor-like electrical behavior from severe damage after 24 h.
2.3
Biodegradability
Biodegradable materials are preferred in skin-inspired applications, which enable the devices to degrade into harmless components after their service life. It is essential for transdermal or implantable devices to avoid side effects for biomedical applications[1]. Intensive research efforts have been focused on developing disintegrable materials like nature-derived materials and polymers, but semiconductors with biodegradability still remains a challenge[55–57].
Biodegradable materials like metals (e.g., Mg, Zn, Mo, and Fe) and silicon-based technology is an interesting option for transient integrated devices with high hydrolysis rate but without any harmful products[58]. Lu et al. constructed transient light-emitting diodes (LEDs) using semiconductor ZnO and an ultrathin electrode of Mo, where each layer can dissolve and degrade in a uniform manner[59]. According to Hwang et al., silicon nanomembranes (Si NMs) showed excellent transient behavior through hydrolysis, with a dissolution rate of 2 nm/day at room temperature (Fig. 3(a)[60]. Combining Si NMs with other disintegrable conductors and dielectric materials, Hwang et al. further designed bioresorbable complementary metal oxide semiconductor (CMOS) circuits, radio frequency (RF) electronics, and biosensors[61, 62]. Silicon-based transient electronics have demonstrated excellent biocompatibility by in vivo and in vitro experiments, which opens up tremendous potential for biomedical applications[63–65].

class="figure_img" id="Figure3"/>
Download
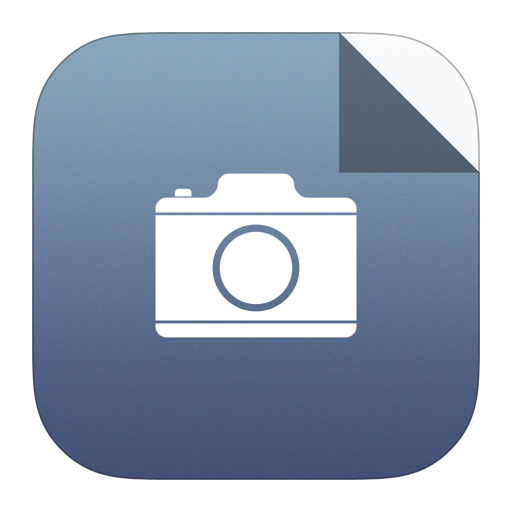
Larger image
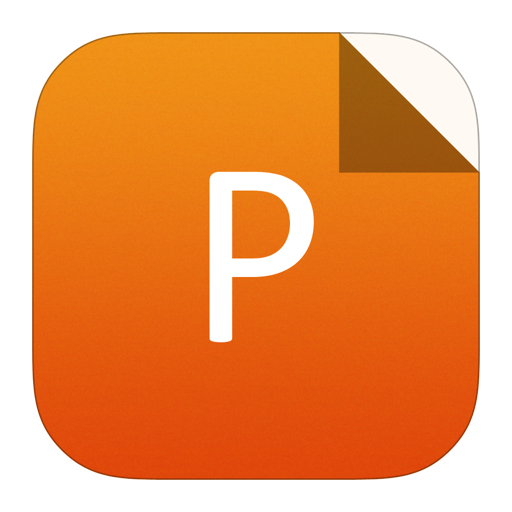
PowerPoint slide
Figure3.
(Color online) (a) Schematic of silicon-based transient electronics on silk substrate (top) and dissolution process in water (bottom). (b) Flexible devices with disintegrable polymers as the active material and substrate (left) and images of a device at various stages of disintegration (right). Panel (a) adapted with permission from Ref. [60]. Copyright 2012, American Association for the Advancement of Science. Panel (b) adapted with permission from Ref. [74]. Copyright 2017, National Academy of Sciences.
Decomposable conjugated polymers have emerged as another attractive choice for transient electronics[66]. Natural or nature-inspired resources (e.g., cellulose, chitin, silk fibroin, egg white, and pectin) are employed in “green” electronics due to their excellent biocompatibility, easy processability, and low cost[67–69]. For example, carbon materials derived from silk feature renewable resources with wide abundance and capability of large-scale production[70, 71]. Some other well-known materials like P3HT, (poly(3,4-ethylenedioxythiophene)-poly(styrenesulfonate) (PEDOT:PSS), and poly(vinyl alcohol) (PVA) have shown excellent biocompatibility in many applications[72, 73].
Based on reversible imine chemistry, Lei et al. synthesized a biocompatible semiconducting polymer that is readily decomposable in mild acidic environments (Fig. 3(b))[74]. Totally disintegrable transistors were prepared using iron electrodes and cellulose-based substrates, which were stable in water and completely degradable under acidic condition within 30 days. Boutry et al. selected several biocompatible and biodegradable materials (polyhydroxybutyrate/polyhydroxyvalerate (PHB/PHV), poly(glycerol sebacate) (PGS), and poly(octamethylene maleate (anhydride) citrate) (POMaC)) to build an arterial-pulse sensor that can be resorbed after several months without requiring removal[75].
3.
Skin-inspired devices and systems
Based on material selection and novel device design, artificial sensors imitating mechanisms and structures of natural skins, along with flexible circuits, are achieved. To go further beyond single-modal sensor unit, intelligent functionalities are incorporated to realize skin-like electronic devices and systems.
3.1
Sensors
Pressure sensing is a common and vital function to convert mechanical stimuli into electrical signals, which has been extensively studied. As an example, Lipomi et al. fabricated a transparent pressure and strain sensor based on elastic films of CNTs (Fig. 4(a))[36]. CNTs were spray-deposited on the elastomeric substrate as compliant conductors. Arrays of capacitive sensors were easily achieved by sandwiching a layer of silicone between two patterned CNT electrodes. Mannsfeld et al. designed a capacitive pressure sensor based on pyramidal microstructured layers[76]. Such a sensor design has been adopted by many research groups due to its high sensitivity and good reversibility. To some extent, these microstructures are similar to those composed of interlocking hills between the epidermis and the dermis, which play an important part in the pressure sense of natural skin[77, 78]. This microstructure-based design is beneficial for ultrasensitive and ultrafast responses, and is thereby compatible with different sensing principles[79–83].

class="figure_img" id="Figure4"/>
Download
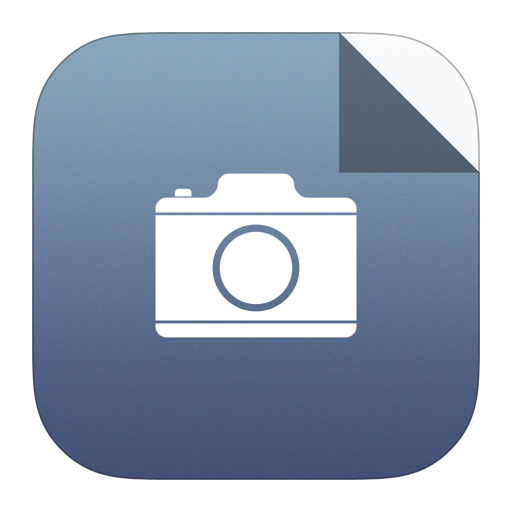
Larger image
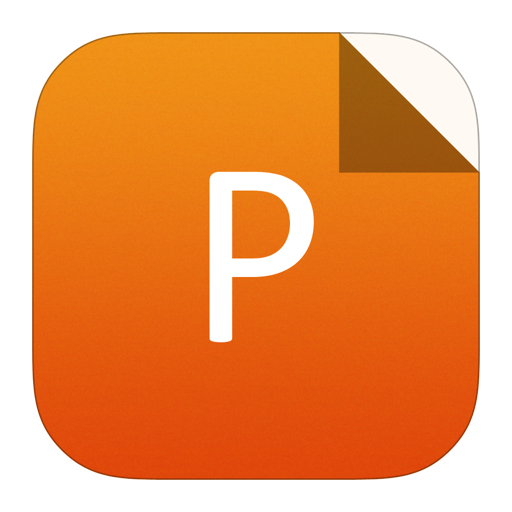
PowerPoint slide
Figure4.
(Color online) (a) The working mechanism of the CNT-based pressure and strain sensor (left) and the array design (right). (b) Schematic and image (inset) of a hierarchically pyramidal-structured pressure sensor. (c) The fabrication step of pressure-sensitive polymer transistor with a microstructured PDMS dielectric layer. (d) Schematic of a chameleon-inspired e-skin. (e) Images of a Si nanomembrane diode sensor array with magnified views of a single sensor. Panel (a) adapted with permission from Ref. [36]. Copyright 2011, Springer Nature. Panel (b) adapted with permission from Ref. [84]. Copyright 2017, IEEE. Panel (c) adapted with permission from Ref. [85]. Copyright 2013, Springer Nature. Panel (d) adapted with permission from Ref. [86]. Copyright 2015, Springer Nature. Panel (e) adapted with permission from Ref. [96]. Copyright 2013, Springer Nature.
Schwartz et al. integrated microstructured PDMS dielectric with polymer transistor to amplify the capacitance responses and enable its operation in the subthreshold regime (Fig. 4(b))[84]. By adjusting the size and density of the pyramidal microstructures, Cheng et al. developed a pressure sensor with high sensitivity and ultralow hysteresis (Fig. 4(c))[85]. Chou et al. reported a chameleon-inspired resistive pressure sensor, in which pressure can be discriminated through visible color change with the aid of stretchable electrochromic devices (ECDs) (Fig. 4(d))[86]. The pressure sensor utilized spray-coated single-walled carbon nanotubes (SWNTs) over a pyramidal-microstructured layer to achieve sensing response by modulating contact resistance. Other microstructures based on nanocracks, nanowires, nanoparticles, and nanospheres are also beneficial for flexible pressure sensors[21, 87–89]. Inspired by the crack-shaped slit organ of the spider, Kang et al. designed a strain and vibration sensor based on the nanoscale cracks of a platinum (Pt) layer with high sensitivity, as a result of the reversible disconnection–reconnection process under the external strain and vibration[90].
In natural skins, ionic mechanotransduction contributes to the conversion of mechanical stimuli into biochemical signals[91, 92]. Inspired by the ionic mechanotransduction in epidermal Merkel cells, Jin et al. reported a piezocapacitive pressure sensor based on artificial ionic ?uids and polymer networks[93]. The sensor demonstrated an ultrahigh sensitivity (1.4 nF/kPa) with room for further improvement by using pillar patterns similar to the periodic ridges in Merkel cells.
Temperature sensing is another vital function of the skin sensory system. Wearable skin thermography represents a complementary technology for traditional infrared imaging and point-contact sensors. Conventional measurement is typically based on temperature coefficient of resistance (TCR) that requires complicated readout circuits for amplification[94]. Semiconductor diodes allow facile temperature readout by the shift of the turn-on voltage[95]. Webb et al. designed a conformal, precise, and continuous temperature sensor array using PIN diodes of doping silicon nanomembranes (Fig. 4(e))[96]. This device array designs allow wearable, continuous, and time-dynamic spatial mapping of body temperature. Polymer composites show high resistivity change with temperature, which is attributed to the transformation of the crystalline phase to the amorphous phase, volume expansion, and the increased inter-particle distance. Jeon et al. enhanced the reproducibility of temperature sensing by utilizing conductive polymer filled with Ni microparticles[97]. The composites demonstrated a high sensitivity (0.3 V/°C), which is applicable for monitoring body temperature.
Considering that natural skin is responsive to various stimuli (e.g., pressure, strain, temperature, and humidity) simultaneously, multiplex sensors are desired for various wearable applications. Multimodal measurement can provide sufficient information to establish interconnections because some signals may be unavoidably affected by others[4, 98]. A trend of skin-inspired system is the integration of multiple types of sensors[99, 100]. Ho et al. developed a flexible, transparent all-graphene multifunctional sensor matrix, with graphene oxide (GO) and reduced graphene oxide (rGO) utilized as humidity- and temperature-sensitive materials, respectively[101]. The sandwiched layer and the sensitive materials allow simultaneous and independent measurement of external stimuli (pressure, humidity, and temperature). Wang et al. reported a silk-derived carbon fiber-based sensor for strain and temperature measurements with high sensitivity (gauge factor ~8350 and 0.81%/°C)[71, 102]. Inspired by the human somatosensory system with distributed receptors, Hua et al. designed a matrix network to greatly expand sensing functionality for eight types of stimuli. The multilayered design featured simultaneous detection and high selectivity with reduced decoupling interferences.
Future efforts may be the integration of biochemical sensors into the skin system, which may target metabolites (e.g., glucose and lactate) and electrolytes (e.g., sodium and potassium ions)[103–105].
3.2
Transistors
Organic field-effect transistors (OFETs) represent the building blocks for complex circuit systems for skin-inspired electronics[106–109]. Traditional inorganic transistors lack the compliant mechanical properties for flexible large-area skin electronics. Intrinsically stretchable organic materials can render such electronic units flexible and soft with low capital cost[37, 84, 110–112]. To achieve lightweight imperceptible electronics, Nawrocki et al. reported an ultrathin (300 nm) electronic skin fit with a transistor and a tactile sensor per pixel, which showed good adhesion and flexibility and can distinguish human touch from metallic touch (Fig. 5(a))[113]. For improved sensitivity and accuracy, a bending-insensitive pressure sensor based on CNTs and graphene was developed that measures only normal pressure and can operate under extreme bending conditions[114].

class="figure_img" id="Figure5"/>
Download
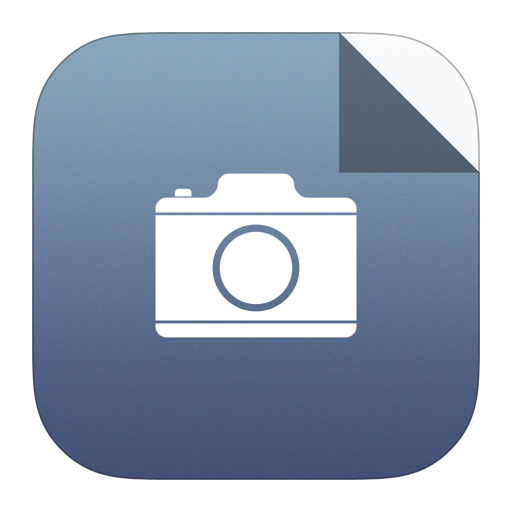
Larger image
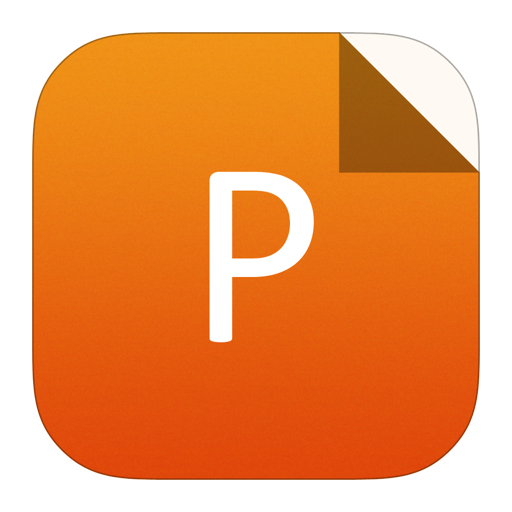
PowerPoint slide
Figure5.
(Color online) (a) A photo (left) and structure (right) of a 300-nm-thick electronic skin, with an OFET and tactile sensor per pixel. (b) Schematic of a strain sensor array based on piezoelectric nanogenerators and coplanar-gate graphene transistors. (c) Images of intrinsically stretchable transistor array (left, scale bar: 1 mm), amplifier in its initial and stretched state (middle), and use of the amplifier for arterial pulse signal measurement. Panel (a) adapted with permission from Ref. [113]. Copyright 2016, Wiley. Panel (b) adapted with permission from Ref. [115]. Copyright 2015, Wiley. Panel (c) adapted with permission from Ref. [125]. Copyright 2018, Springer Nature.
A transistor-based matrix exhibits advantages in imitating skin that consists of thousands of sensory units due to advanced sensing performances, minimized scale, large-scale integration, and lower signal cross-talk as compared with simple resistive or capacitive sensors[115–117]. Pfattner et al. reported a reversible and reliable pH sensing transistor based on an organic semiconductor with tunable sensitivity[118]. Zhu et al. reported organic thin-film transistor-based temperature sensors, which can suppress strain and improve the sensing accuracy afforded by the differential readout[119]. In order to simplify the fabrication process of transistor-based devices and arrays, Sun et al. adopted a novel transistor architecture design of a coplanar-gate graphene transistor-type pressure sensor matrix (4 × 4 pixels), which provided a simple route to flexible, low-cost transistor-based sensors[120]. Combined with piezoelectric polymers, the coplanar-gate graphene transistors were integrated into a strain sensor matrix that maintained output values under continuous strains and exhibited high performances (gauge factor = 389) (Fig. 5(b))[115].
In addition to simply recording human vital signs, transistor-based sensing devices are also favorable for in-sensor signal processing, such as direct signal amplification and noise elimination[121–123]. Molina-Lopez et al. reported inkjet printed transistors by stacking stretchable materials (PEDOT:PSS, CNTs, poly(vinylidene fluoride-co-hexafluoropropylene) (PVDF-HFP)), and the device was able to mimic synaptic neuron behavior, demonstrating potential for in vivo interfacing with biological systems[124]. Wang et al. recently reported the first large-scale fabrication method for an intrinsically stretchable organic transistor array (Fig. 5(c))[125]. The transistor utilized polymer dielectric, polymer semiconductors, and CNT electrodes. The transistor further enables the demonstration of constituted analogue and digital circuit elements (e.g., inverter, NAND gate, and amplifier) for wearable signal processing units.
Soft electronic devices have shown capabilities of acquiring high-quality biosignals due to the lowered interfacial impedance, reduced signal distortion, and high signal-to-noise ratio (SNR). Sugiyama et al. designed an ultraflexible organic differential amplifier for signal amplification and noise reduction, which allows the recording of weak electrocardiogram (ECG) signals with high signal integrity and sensitivity[126]. Lee et al. reported an organic electrochemical transistor (OECT) based on a non-volatile gel electrolyte as the electrophysiological sensor, which demonstrated high SNR of 24 dB, mechanical stability, and capability of long-term monitoring and multiple use[127].
3.3
Circuit systems
For skin-inspired electronics, high-density functional circuits play an important part in signal collection, processing, and transmission, which pave the way for applications in implantable sensors, tissue engineering, and soft robotics[128]. For example, analog signals have to be converted into electrical pulses because they cannot be directly transmitted through the nervous system[129]. Amplifiers are necessary to alter the amplitude of collected signals and the analog-to-digital (A/D) converter can help reduce the effects of interference[9]. Gao et al. designed a flexible integrated plastic-based sensor array with silicon integrated circuits for multiplexed perspiration analysis, bridging the existing gap between signal transduction, conditioning, processing, and wireless transmission[103]. Pre-processing techniques based on local conditioning and error-suppression circuits are common in rigid systems, but have not been effectively investigated in flexible and stretchable systems[119]. Therefore, Zhu et al. adopted static and dynamic differential readout approaches to suppress strain-induced errors in a CNT transistor-based temperature sensor and achieve an inaccuracy of only ±1? °C within 0–60% strain[130].
The key challenges for the development of flexible circuit systems lie in thin-film interconnects and circuits compatible with flat encapsulations[14]. The flexible electronic components are typically based on metal/silicon nanomembranes, carbon-based materials, and organic semiconductors[113, 131–133]. For example, Kim et al. described an epidermal electronic system, in which all the components are in the form of mechanically skin-like membranes (Fig. 6(a))[15]. The device exhibited ultrathin layout (7 μm) with improved geometrical designs, utilizing serpentine-shaped nanomembranes of silicon and gallium arsenide as active elements. This design may be further combined with a temporary transfer tattoo as the encapsulating layer to enable soft, flexible, and invisible contact with the skin[134, 135]. Compared with current printed circuit boards, this technique offers skin-like mechanical properties and breathability, which avoid constraints in motions, discomfort, or inflammation[136]. Recently, Tian et al. reported ultra-large-area, multifunctional epidermal electrical interfaces to record body-scale electrophysiological signals[137]. Extending scales of wearable, epidermal electronic circuits can provide robust and accurate body-scale recording capabilities, and is expected to be applied in control of a transhumeral prosthesis and cognitive monitoring.

class="figure_img" id="Figure6"/>
Download
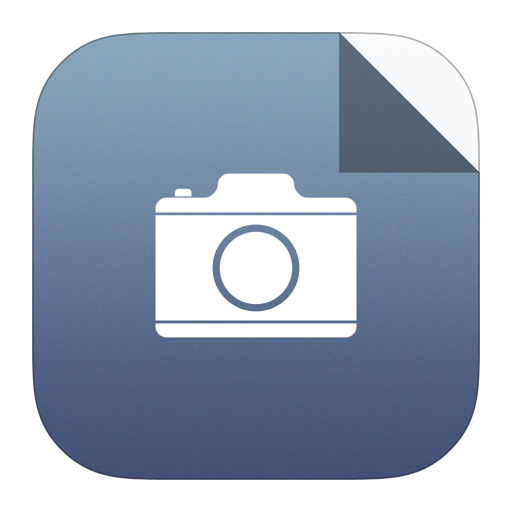
Larger image
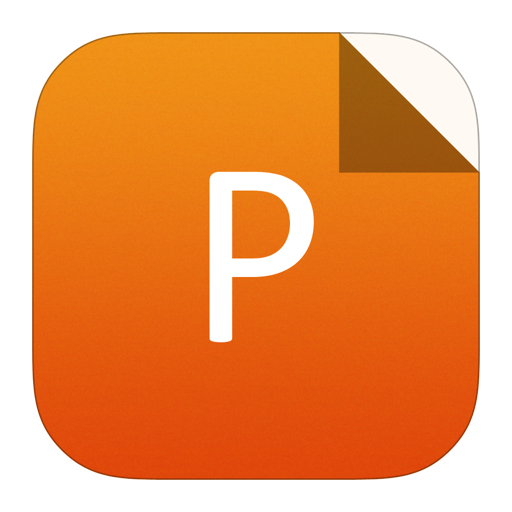
PowerPoint slide
Figure6.
(Color online) (a) A multifunctional epidermal electronics (left) and its integration with tattoo. (b) Design of CNT TFT device (left) and photos of devices attached conformally to human skin (right). (c) Schematic illustration of a digital tactile system composed of flexible organic circuits. Panel (a) adapted with permission from Ref. [15]. Copyright 2011, American Association for the Advancement of Science. Panel (b) adapted with permission from Ref. [143]. Copyright 2019, Springer Nature. Panel (c) adapted with permission from Ref. [144]. Copyright 2015, American Association for the Advancement of Science.
In addition to the design of circuit layout, 3D integration is another efficient method to create stretchable electronic systems with complex functions[138]. Huang et al. reported the fabrication of 3D electronics through layer-by-layer transfer printing of deformable circuits onto elastomeric substrates[139]. Functional component (e.g., electrodes, sensors, amplifiers, and radiofrequency units) was integrated with serpentine Cu/polyimide conductor and metallic vias. A wireless multichannel sensing system detecting vital body signals was developed based on the 3D circuit.
In spite of the stretchability and flexibility for the overall system, the rigid island design has to deal with the complicated layout and fabrication process. The robustness of the circuit is often compromised by the weak interfaces between hard and soft components. Fully stretchable circuits based on compliant materials exhibit the inherent advantage in terms of the resilience[140, 141]. Lee et al. demonstrated an ultrathin, transparent, and flexible transistor array for skin-like displays, which showed reliable high performance under various mechanical deformations[142]. Lei et al. reported large-scale flexible circuits based on polymer-sorted semiconducting CNTs (Fig. 6(b))[143]. The CNT TFTs demonstrated good uniformity and high performance. Circuit components, including basic logic gates, ring oscillators, sequential circuits, and gain amplifiers, were fabricated based on the CNT TFT, which demonstrates great potential for sensor acquisition systems in large-scale flexible electronics. Tee et al. designed a complementary ring oscillator consisting of three inverters utilizing organic semiconductors and silver electrodes (Fig. 6(c))[144] The oscillator demonstrated pressure-dependent output that mimics skin mechanoreceptors with low power consumption and stable oscillations. As the study illustrates, organic circuits are well-suited for various printing techniques such as gravure printing and inkjet printing, which allows low-cost fabrication of high-density skin electronics over a large scale[125, 144–146].
3.4
Integrated skin-like functional systems
Skin-inspired electronics are attractive for various emerging fields such as wearable healthcare monitoring, soft robotics, and human–machine interface[4]. The ability to make a fully functional artificial skin system has become a barrier that needs to be solved from the system-level functions of communication and processing instead of materials and electronics[147].
(1) Wireless data transmission
The trend for the next-generation electronic skin applications is developing wearable, on-demand, non-invasive, transdermal devices that detect physiological signals in real time[148, 149]. Research has focused on monitoring single/multimodal physical/biochemical information related to human health status such as body temperature, pulse waveforms, cardiovascular signals, respiration signals, and metabolites in sweat. To satisfy the needs for daily use, a wearable and portable device without complex wires and additional instruments is highly desired. Chung et al. recently designed a wireless epidermal monitoring system suitable for the attachment to neonatal skins[150]. The system incorporated serpentine metal traces to record electrocardiograms (ECGs) and photoplethysmograms (PPGs), which further employs chip-scale integrated circuit components for in-sensor data processing and analysis. Radio frequency identification (RFID) has been widely used in the wearable devices by eliminating wired connections and power supply for communications with portable terminals like smartphones[151–153]. Chen et al. designed a wireless, millimeter-scale resonant sensor with pressure sensitivity based on power reflection distortion (PRD) and group delay distortion (GDD) detection[154]. This method achieved compact size (1 × 1 × 0.1 mm3) for pressure monitoring, which allows in vivo tracking of pulse waveform and intracranial pressure (ICP). Niu et al. recently designed a body area sensor network (bodyNET) composed of on-body sensors and circuits for signal detection and wireless transmission (Fig. 7(a))[155]. The printed stretchable sensors did not require additional batteries and silicon chips, which were coupled with unconventional strain-tolerant RFID tags to exchange data with on-textile readout circuitry. The bodyNET is an appropriate candidate for continuous monitoring physiological signals over long-term even including sleep and exercise.

class="figure_img" id="Figure7"/>
Download
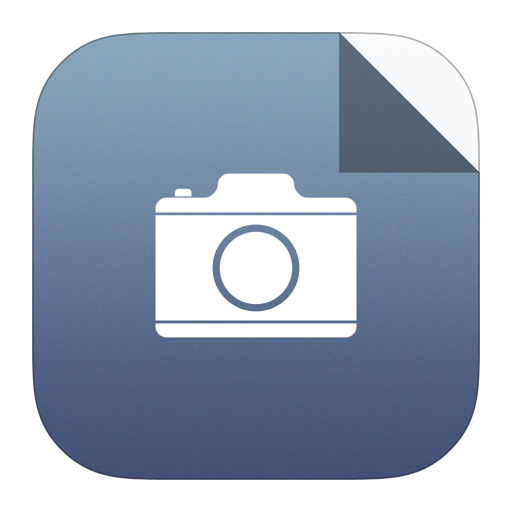
Larger image
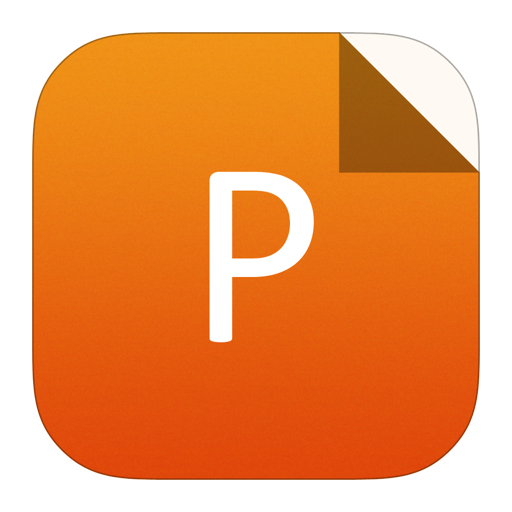
PowerPoint slide
Figure7.
(Color online) (a) Schematic illustration (left) and photo (right) of a bodyNET with on-skin sensors and flexible circuits on clothes. (b) A piezoresistive sensor array consisting of 548 sensors covering the entire hand. (c) A self-healable electronic skin system composed of sensors and display. Panel (a) adapted with permission from Ref. [155]. Copyright 2019, Springer Nature. Panel (b) adapted with permission from Ref. [163]. Copyright 2019, Springer Nature. Panel (c) adapted with permission from Ref. [49]. Copyright 2018, Springer Nature.
(2) Large-scale array
Electronic skin may serve as the enabler of tactile sensation for humanoid robots and prostheses[147]. To fully mimic human skin, these devices are expected to conformally cover non-planar surfaces over large areas such as the fingers and joints[156–158]. A high spatio-temporal resolution and mechanical reliability is important for soft electronic skin systems to achieve various perceptions. Kim et al. designed a sensor array based on silicon nanoribbons for monitoring pressure, strain, and temperature[159]. The ultrathin and stretchable silicon and gold nanoribbons allow the whole device to cover the entire surface of a prosthetic hand. The resolution of the large-area sensor array is sufficient to distinguish many operations in daily life. Viventi et al. developed a class of flexible electronics based on silicon nanomembranes for implantable devices[160]. 2016 silicon nanomembrane transistors were integrated within a sensor system (measuring 14.4 mm by 12.8 mm). The device consisted of 288 amplified and multiplexed channels to enable simultaneous sampling of signals with millimeter spatial and sub-millisecond temporal resolutions. The enhanced spatial resolution presents challenges in sensor miniaturization and complexity of interconnection[161]. By utilizing scan and data lines, Wang achieved an active sensor matrix with a density of 47 transistors per cm2 and a resolution of one sensor per 2 mm, which allows accurate identification of a small ladybug[125].
Large-scale, high-resolution tactile datasets bring about abundant information for advanced signal processing tools such as machine learning[162]. Smart artificial systems are expected to be able to fully mimic humans in performing complicated operations such as distinguishing the contact position, direction, and magnitude of mechanical stimuli. Sundaram et al. recently designed a low-cost, scalable piezoresistive sensor array (548 sensors) on a knitted glove (Fig. 7(b))[163]. Using a convolutional neural network, the sensor array is able to recognize gestures, estimate categories, and distinguish the grasped objects with enhanced accuracy. This attempt was beneficial for the future development in soft robotics and human–machine interfaces, by providing insights into the dynamics in human action and correspondences between tactile signals.
(3) Closed-loops with feedback
For artificial intelligent electronic skin, sensory feedback is critical in building closed-loop control systems. For example, the effective and precise control of our hands largely rely on the sensory feedback and feedforward motor commands[164]. Provided with sensory feedback, artificial skin systems are expected to take full bionic replacements instead of acting as suboptimal assistive devices especially for skin prosthesis. Automatic tactile feedback provides enhanced operability and improved performances, such as robots that replace humans in hazardous environments or improve perception of tissue consistency and compliance in surgery[147]. For example, visual feedback was utilized with prosthetic sensors in a human-in-the-loop system by Gerratt et al.[165]. When the smart tactile glove grasped an object, the color representing the tactile information was presented to the wearer for adjustment to the target contact pressure. Son et al. designed a multifunctional electronic skin system composed of self-healable sensor and light emitting capacitor (LEC) display (Fig. 7(c))[49]. Each electronic module was integrated onto a polymer substrate efficiently by self-bonding to achieve a single on-board system. LEC pixels turned on and off according to the detected signals (e.g., ECG signals, heart rate, and strain values) for visual interpretation.
Another important step towards a smart electronic skin system is closed-loop system with instant smart drug delivery for point-of-care (PoC) healthcare applications[166, 167]. The collected information is processed and transmitted to active components for diseases treatment accordingly. Lee et al. reported an electrochemical device for diabetes monitoring and therapy[168]. The graphene-based device arrays are capable of monitoring glucose concentration and pH in sweat. A high glucose level triggers the heaters to dissolve the phase-change material-coated polymer microneedles, from which the drugs are released into the bloodstream to treat diabetes. Microneedles that painlessly pierce the epidermis are considered an efficient and fast platform for delivery of drugs, nanoparticles, and biomolecules[169–171]. Various monitored signals such as body temperature, tremors, sweat metabolite levels can actuate the drug delivery from carriers such as stimuli-responsive copolymers and thermo-responsive phase change materials[96, 172, 173]. The integrated sensing system, together with feedback therapy, has provided solutions for in-time, self-administrated treatment of chronic diseases with reduced disruption.
4.
Conclusion and outlook
In summary, we have reviewed recent progress in skin-inspired electronics from the aspect of semiconducting materials, sensor devices, and advanced systems. Inspired by the structures and mechanisms of living organisms, recent progress in soft materials and flexible electronics is beneficial for the achievement of skin-like materials and biomimetic sensors. An intelligent skin electronic system with advanced functions and capabilities like wireless data transmission, data collection of large-area arrays, and in-time feedback is explored to favor the real-world applications in fields such as wearable healthcare monitoring, human–machine interfaces, and smart robots/prostheses.
Several limitations still exist that require further studies including but not limited to the following aspects: (1) Enhancement of the functional characteristics. The flexibility, stretchability, biocompatibility, as well as sensing performances (including sensitivity, response speed, stability, anti-interference ability, and multimodal capability) need to be further improved for different application settings of wearable and implantable devices[174]. (2) Achievement of intelligent systems. A long-term picture beyond artificial skin lies in the integration of additional functions such as self-powered supply, in-sensor signal processing, and data storage[146]. (3) Simplification of the device layout and fabrication process. Large-area coverage of active electronic components is expected to be realized with simple, low-cost methods such as printing techniques[175, 176]. Overall, the rapid advancements along with the practical challenges in skin-inspired electronics suggest a bright future for this active field.
Acknowledgments
This work was supported by the National Natural Science Foundation of China under Grants 61825403, 61674078, and 61921005, the National Key Research and Development program of China under Grant 2017YFA0206302, and the PAPD program.