1.
Introduction
The development of modern integrated electronics has been established upon bulk crystalline silicon (c-Si), which is almost the best-known semiconductor material that boasts a vast knowledge base of how to establish precise micro processing, reliable passivation and doping controls for complementary logics. In addition, c-Si is earth-abundant, non-toxic and bio-friendly to human tissues, but however a rigid and brittle crystal that allows little flexibility, not to mention, stretchability. This has almost excluded it as a candidate for direct applications in soft electronics that are supposed to deploy or attach to curvilinear skin or tissue surfaces for bio-sensing, health monitoring or prosthesis implantation[1–6]. In the meantime, most flexible or stretchable electronics have been prototyped with soft polymer, elastomer and organic semiconductors, which are intrinsically soft but also hard to deliver the high carrier mobility and excellent stability and doping controls established based c-Si material[7–12]. So, is it possible to construct high performance soft electronics based on the hard c-Si technology? This has been posed as a very interesting and yet direction to explore.
Fortunately, as illustrated in Fig. 1, a quasi-one-dimensional (1D) form of silicon nanowire (SiNWs) is highly flexible and bendable[13–15]. However, the straight SiNWs are not necessary stretchable, as only a 3% tensile strain will cause a breakage or irreversible plastic deformation in the SiNWs with a diameter of only tens of nanometers[16]. It is important to note that, this is insufficient to design and fabricate a soft and comfortable electronics attached to human skin, as the latter has a typical stretchability of ~30%[5]. Fortunately, the line-shape degree of freedom (DOF) of the 1D SiNW channels, can be engineered to endow extra stretchability. For example, an elegant way to do that is to tailor the c-Si NWs into elastic line-shape of springs, fractal 2D patterns[17] or just 3D nano coils[18–24]. In this way, the axial stretching strain can be well translated into local bending of the curved segments of c-Si springs, as schematically depicted in the low panels in Fig. 1(b).

class="figure_img" id="Figure1"/>
Download
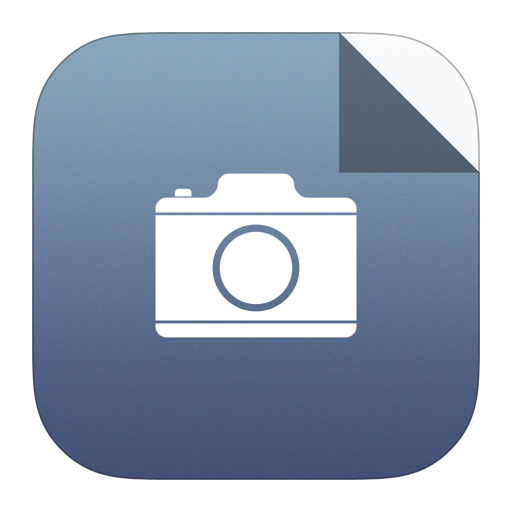
Larger image
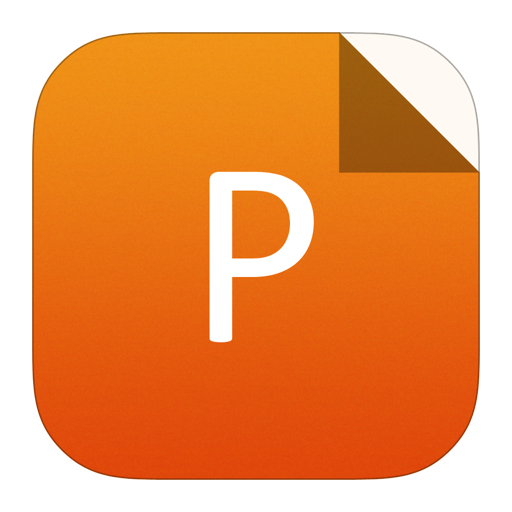
PowerPoint slide
Figure1.
(Color online) Schematic illustrations for the comparison of (a) the crystal pulling of thick bulk c-Si ingot at high temperature, to (b) the self-assembly growth of Si nanowires (SiNWs) at a much lower growth temperature, with yet a new possibility to engineer the line-shape for extra flexibility and stretchability.
The SiNWs, as well as NWs in other materials, can be fabricated via a low-cost, high yield and high throughput vapor–liquid–solid (VLS) self-assembly growth, where the absorption of gaseous precursors, for instance, the SiH4 gas for the growth of SiNWs, is catalyzed by the tiny metal nano-droplets that mediate a continuous deposition and uniaxial extension of the SiNWs[25, 26]. The formation of helical nano coils of SiO2 or SiOx have been first observed in an iron-catalyzed VLS growth, but at a rather high temperature of 1160 °C[18, 19], while the as-produced silica coils are only insulating dielectric. Later on, self-assembly SiNW springs and coils were produced by a wide range of different techniques that include laser ablation, post-annealing in reductive ambient or pressure-modulated chemical vapor deposition (CVD) processes[20–24]. In parallel, the initially straight SiNWs can be shaped into elastic sinusoidal spring by attached polymer substrate buckling[17, 27–29]. Despite of successful proof-of-concept demonstrations, there are still important technical difficulties that hinder a large scale integration of the VLS-grown nano springs for stretchable electronics. First, most of the VLS-grown nano springs or coils are produced as randomly distributed array or bundles, making them rather difficult to position, align and connect the Si nano-springs or coils upon foreign substrate for electronic applications. Second, the post-growth transferring and buckling of the brittle c-SiNWs are also challenging and risky. Therefore, in order to achieve a deterministic geometry control of the c-Si channels, recent efforts have been directed to elaborating 2D nano c-Si ribbons or serpentine channels by direct electron beam lithography (EBL), etching and patterning of the ultra-thin monocrystalline c-Si layer in SOI substrate[30–32]. The EBL technique has also been used to define nanoscale guiding channels to direct the VLS-grown SiNWs into pre-defined sinusoidal line-shapes[33]. Though largely stretchable c-Si nano channels can be produced by these ways, the high fabrication cost associated with the EBL elaboration is unacceptable for establishing a scalable and stretchable electronics.
In addition, the ratio of the c-Si spring channel diameter to the radius of the turning tracks, defined as
m{alpha }} equiv {R_{
m t}}/{{{d}}_{{
m{nw}}}}$

On the other hand, in view of future device applications, the SiNW channels are supposed to be positioned and electrically connected in the mainstream planar circuitry. To this end, vertical VLS-grown SiNWs or out-of-plane 3D nano coils are difficult to handle in conventional lithography, and thus difficult to integrate in the mainstream planar architecture. So, the c-Si springs are also supposed to grow into 2D planar spring, ideally via a high-yield and low-cost self-assembly process, with precise location control for subsequent electric devising. This has been a prerequisite for developing scalable and batch-manufacturable flexible and stretchable c-Si electronics.
In this review, we will first introduce a new in-plane solid-liquid-solid (IPSLS) growth mechanism of SiNWs, where solid amorphous Si (a-Si) thin film is absorbed as precursor to surface-running metal droplets to produce crystalline SiNWs. Then, two approaches to tailor the line-shape and geometry of the in-plane growth of SiNWs will be demonstrated, with the aids of a strong interface interaction or via a faithful guided growth technique. The focus will be placed on enabling a precise, scalable and readily engineerable low-cost fabrication of c-Si nano springs. Finally, the mechanical and electric transport properties of the in-plane SiNW springs will be presented and discussed, with a perspective for future high performance c-Si based stretchable electronics.
2.
In-plane growth of Si nanowires
As schematically illustrated in Fig. 2, both the VLS and the IPSLS growth of SiNWs are mediated by nano droplets of metal catalyst. The major difference arises from the different feeding precursor, where the VLS is carried out in a gaseous environment with SiH4, while the IPSLS takes place by absorbing surface-coating a-Si thin film as precursor. The driving force for the in-plane growth is the Gibbs energy difference[36–38], seen by the Si atoms, in the amorphous and the crystalline state, as depicted in the upper panel in Fig. 2(b), which is known to be δG = 0.1 to 0.15 eV[39, 40], depending on the disordering and distortion of the hydrogenated a-Si (a-Si:H) network matrix.

class="figure_img" id="Figure2"/>
Download
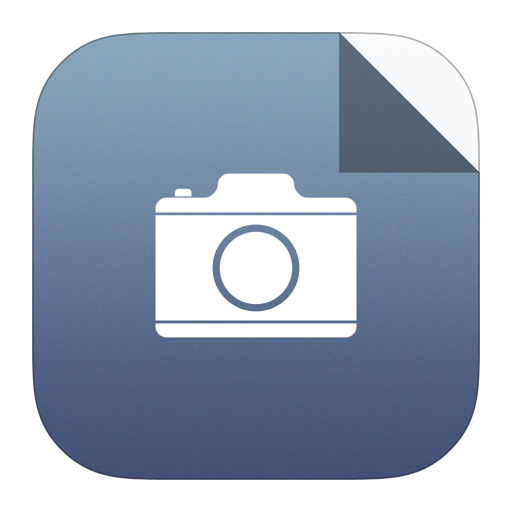
Larger image
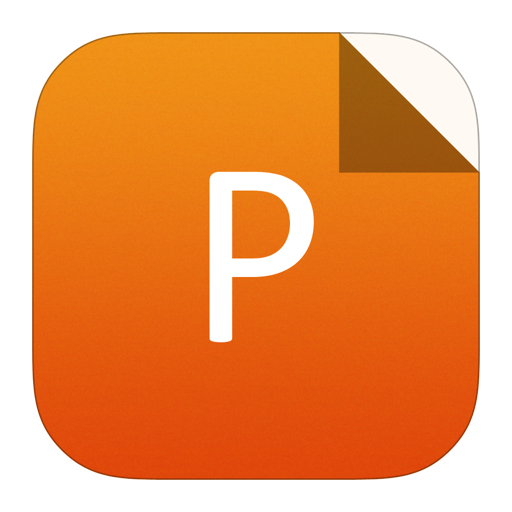
PowerPoint slide
Figure2.
(Color online) Typical SEM images of the low melting point metal (indium or tin) droplet catalyzed (a) vertical VLS growth of standing silicon nanowires (SiNWs) in a gaseous feeding environment, compared to (b) the in-plane solid–liquid–solid (IPSLS) growth of lateral SiNWs that take in amorphous Si (a-Si) thin film as precursor. The upper panel illustration in (b) depicts the driving energy for the IPSLS growth, where Si atoms in a-Si have a higher Gibbs energy, with respect to crystalline Si phase. While both growth mechanisms have a solid–liquid (S–L) rear deposition interface, the front absorption interface in IPSLS growth is relatively hard, as it is also of S (a-Si)–L (liquid droplet) type, which is remarkably different from the soft gas–liquid (G–L) front interface in VLS growth. This results in a strong front-rear interface interaction during an in-plane growth, as a basis for geometry engineering of the in-plane SiNWs. Adapted from Ref. [36–38, 41].
Usually, the fabrication procedure of in-plane SiNWs involves several key steps as depicted in Figs. 3(a)–3(c), (1) first, the evaporation of catalyst metals of indium (In) or tin (Sn) pads of 5–40 nm thick upon glass or wafer substrate, which are then treated by H2 plasma at 250 to 350 °C in a plasma-enhanced chemical vapor deposition (PECVD) system to reduce the surface oxide layer, which thus allows agglomeration of discrete metal droplets with a controllable diameter from several tens to several hundred nanometers by tuning the temperature and plasma conditions; (2) Then, the substrate temperature is lowered to around 100 to 180 °C for a SiH4 plasma deposition of an a-Si:H layer to typically 10 to 80 nm thick. Note that, the metal droplets become frozen at this low temperature below their melting points of 167 °C for indium and 252 °C for Sn; (3) After that, the samples are annealed at a high temperature of 350 °C in vacuum or H2 gas environment (without plasma). During this course, the metal droplets become molten again and start to absorb the a-Si medium in contact to nucleate and precipitate c-Si seeds. The enlargement of the c-Si seeds will eventually force the catalyst droplets to move laterally and produce crystalline SiNWs along their moving courses. At the end, the remnant a-Si thin film can be selectively etched off by a low temperature H2 plasma etching at around 100 to 150 °C, leaving only c-Si NWs on the substrate surface. The whole procedure, as well as their process temperature ranges, are summarized and listed in the Fig. 3(e), while the typical SEM images of the in-plane SiNWs, with indium as catalyst, are shown in Fig. 3(d) and its inset. More experimental details are available in the previous works[36–38].

class="figure_img" id="Figure3"/>
Download
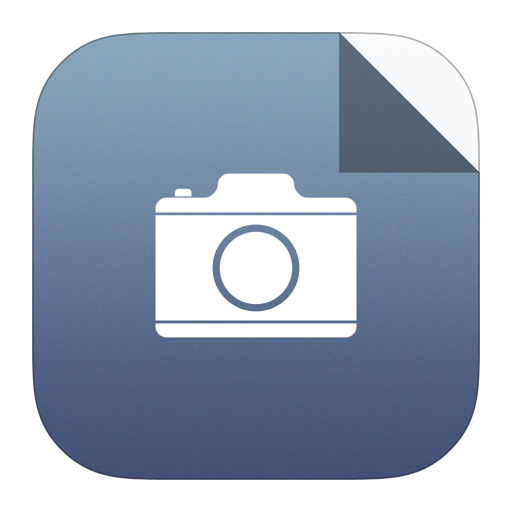
Larger image
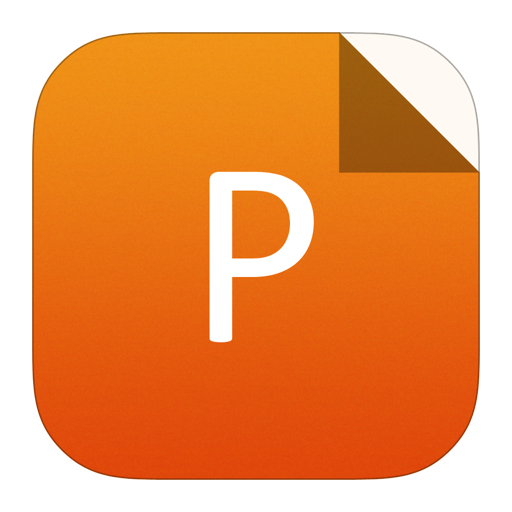
PowerPoint slide
Figure3.
(Color online) Fabrication procedure for the in-plane SiNWs, which involves (a) H2 plasma treatment for catalyst droplet formation, (b) a-Si:H layer coating as precursor and (c) in-situ annealing in vacuum for in-plane growth. (d) shows the typical SEM image of the free in-plane SiNWs, while (e) summarizes the overall thermal budget for each fabrication step. Adapted from Ref. [36].
The consequence of replacing the SiH4 gas feeding with a solid a-Si precursor layer is non-trivial. As indicated in Fig. 2(b), during the in-plane growth of SiNWs, both the front absorption and the rear deposition interfaces are solid/liquid boundaries, which are usually considered as hard interfaces that can collaborate and exert significant pulling or squeezing forces to deform the liquid droplets sandwiched in between. As a consequence, the morphology of the as-produced SiNWs can be engineered to a large extent. This is in a strong contrast to the soft gas feeding environment encountered for the liquid catalyst droplet during a VLS growth of vertical SiNWs, where the top absorption gas/liquid interface has little, if not absolutely no, force to distort the droplet residing over the tip. The strong interface interaction, happened during an in-plane growth of SiNWs, provides thus an unprecedented new control dimension to tailor the line-shape geometry of c-Si NWs, which is enabled by taking a-Si thin film as precursor.
3.
Line-shape engineering by dynamic interface interaction
During a stable in-plane growth of SiNWs, the mass conservation or balance condition demands that the Si atom inflow from the front absorption liquid/a-Si interface,
m{in}}}} = {v_{
m{a}}}{d_{
m{c}}}{h_{
m{a}}}$

m{out}}}} = {v_{
m{d}}} d_{{
m{nw}}}^2$

$${J_{{ m{in}}}} = {J_{{ m{out}}}} to frac{{{v_{ m{a}}}}}{{{v_{ m{d}}}}} = frac{{d_{{ m{nw}}}^2}}{{{d_{ m{c}}}{h_{ m{a}}}}} = ffrac{{{d_{ m{c}}}}}{{{h_{ m{a}}}}} to eta equiv frac{{{v_{ m{a}}}}}{{{v_{ m{d}}}}}~frac{{{d_{ m{c}}}}}{{{h_{ m{a}}}}}, $$ ![]() | (1) |
where
m {nw}}}/{d_{
m c}} sim 0.4;{
m{to}};0.6$

m{c}}} > {h_{
m a}}/f$


class="figure_img" id="Figure4"/>
Download
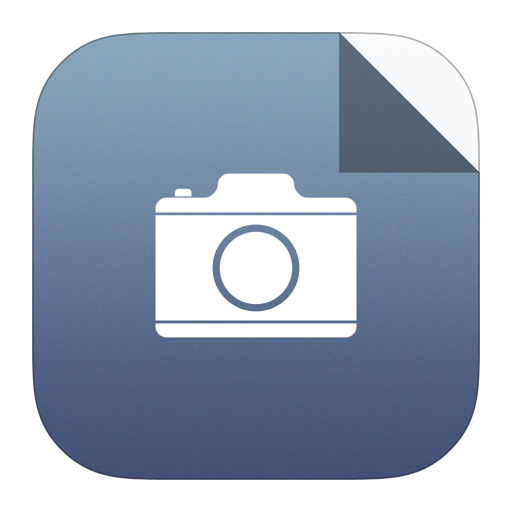
Larger image
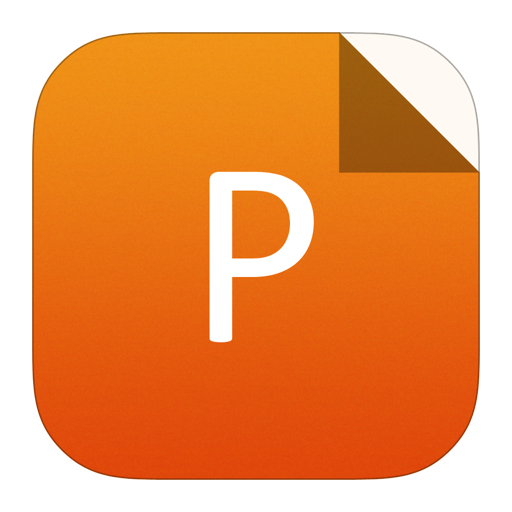
PowerPoint slide
Figure4.
(Color online) (a)–(c) show the typical SEM images of the in-plane SiNWs, grown with different balance condition gauged by a factor of
m c}}/{h_{
m a}}$
Though this interface-interaction geometry control has been first explored in In-mediated in-plane growth of SiNWs, a stable zigzag line-shape engineering and a batch-manufacturing of regular zigzag SiNWs has been achieved only recently by taking Sn droplets as catalyst[42]. The typical SEM images of the Sn-catalyzed zigzag SiNWs are shown in Figs. 5(a) and 5(c), where the zigzag SiNW springs grew out of the edge of pre-patterned Sn pads, and extended over a length of tens of micrometers into the open area covered with a-Si:H. Their initial diameters usually ~200 nm but gradually shrink to the end and split into tiny tails, as revealed after selective etching of the remnant a-Si:H layer by a H2 plasma in the enlarged SEM inset of Fig. 5(a). The white regions on both sides of the in-plane SiNWs are empty of a-Si:H, left by a wider absorption swath of the leading Sn catalyst droplets. Meanwhile, it is also interesting to note that, while the zigzag SiNW springs are produced with growth condition of η < 1, the Sn-catalyzed growth SiNWs with a balance condition of η > 1 can introduce, instead, another novel island-chain SiNW structure, as a result of the Plateau-Rayleigh instability and periodic deformation happened in the leading liquid Sn droplet under large interface stretching [43].

class="figure_img" id="Figure5"/>
Download
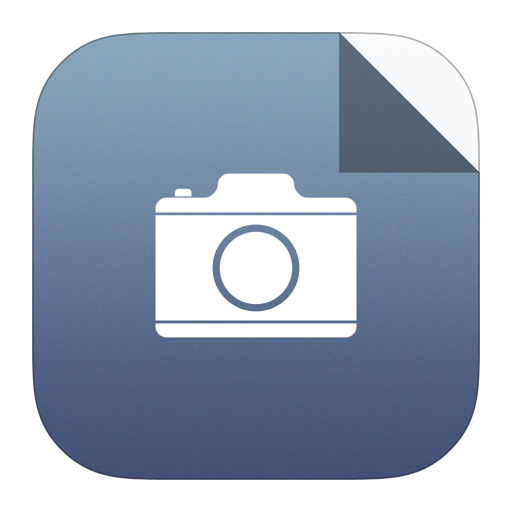
Larger image
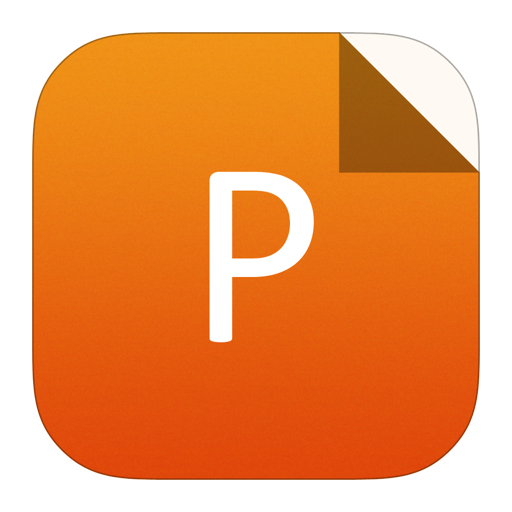
PowerPoint slide
Figure5.
(Color online) (a), (c)–(e) show the typical SEM images of the zigzag SiNWs, with an enlarged view of the final end of the in-plane SiNW presented in the inset of (a), where the remnant a-Si:H layer has been selectively etched off. (b) illustrates the in-plane turning twinning sequence in a Si [
Importantly, it has been found that the zigzag growth of SiNWs is highly reproducible with Sn as a catalyst metal, which is an important basis for carrying out a systematic investigation of the mechanical properties of the zigzag SiNW springs. Compared to metal catalyst of In, Sn has a much higher solubility of Si and thus could provide a larger driving force for the lateral growth, and also a stronger interface interaction to trigger a regular zigzag growth dynamics. In addition, for a given a-Si:H thin film of 20 nm, only the Sn droplets with a diameter of 200–500 nm will successfully kick off. Smaller or larger catalyst droplets either diminish quickly into flat tail-like structures or suffer from an insufficient Si supply to activate the lateral growth, respectively. This leads to an effective self-selection of the SiNWs that can pull out from the Sn pad edge, making them all similar in initial diameters, being proportional to the catalyst diameter. Meanwhile, according to the experimental observations, the diameter of the in-plane zigzag SiNWs is basically proportional to the size of the catalyst droplets, and thus thinner SiNWs can be produced, in principle, with smaller Sn droplets and a matched a-Si:H layer thickness to guarantee a balanced in-plane growth.
Furthermore, a close scrutiny of the regular SiNW spring in Figs. 5(c) and 5(e) shows that the zigzag growth involves a sequence of regular orientation switching, as plotted in Fig. 5(f) in terms of the deviation angle from the main advancing direction (along Si [11-2]) against the length of SiNW. A high-resolution transmission electron microscopy (HR-TEM) characterization of a segment of such zigzag SiNW, transferred and mounted upon a copper grid, is shown in Fig. 6, where a regular and periodic self-turning dynamics has been superimposed upon an in-plane growth along Si [11-2] direction. Remarkably, there are at least four clearly resolved turnings in each complete cycle, which is in strong contrast to those relatively simple kinking geometries observed in the VLS nanowires with usually only a single type of turning angle[22, 23, 44, 45]. This indicates a very different mechanism for this in-plane SiNW spring structure, other than a simple twin-kinking dynamics. Meanwhile, it is noteworthy that a multi-step or continuous turning can better accommodate and dissipate the local stress and strain, and thus serving as spring than simple zigzag with sharp turning angles[22, 23, 44, 45].

class="figure_img" id="Figure6"/>
Download
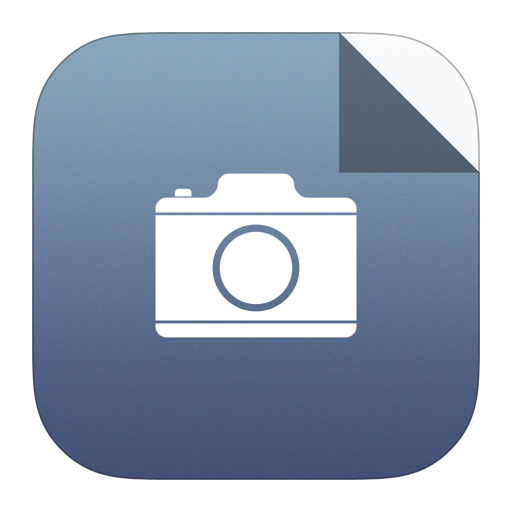
Larger image
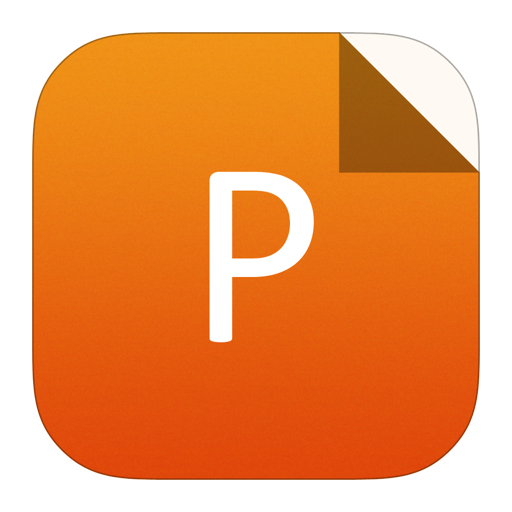
PowerPoint slide
Figure6.
(Color online) HR-TEM characterizations of a segment of a zigzag SiNW: Fourier transform diffraction patterns of different segments for Domain A are presented in (a) and (c) [or Domain B in (e) and (g)]; (b) and (f) show the local HR-TEM lattice images of the SiNW segment at places marked by the green or red spots, respectively; (d) shows the reconstruction of the whole SiNW by adding up local HR-TEM images, with a series of parallel white dash-lines marking the locations of twin planes, and green or red arrows indicating the local growth orientation in Domain A or B[42].
The internal lattice structure of the SiNW springs is also very interesting, which are composed of two interlaced crystal domain structures, labeled as Domain A (in red) or Domain B (in green) in the HR-TEM images in Fig. 6. Surprisingly, the mono-crystal domains are delimitated by a series of parallel twin planes at the middle of the turning arms, as highlighted by the white-dashed lines. The Domain groups, though separated from each other, are still found to be coherent, as inferred from their identical 2D Fourier transform patterns taken in the separated Domains. More strikingly, this self-turning sequence is also found to follow a specific crystallographic-index-lowering order, as summarized in Fig. 5(b). More discussions and experimental details of this intriguing new phenomenon are available in the reference[43].
As the in-plane growth of SiNW springs requires no gas feeding, the growth dynamics that leads to the formation of such zigzag SiNWs can be directly observed in a SEM system equipped with heating stage. For example, in Fig. 5(d), we present 3 snapshots of the Sn-catalyzed SiNW growth, while more growth details are provided in previous work[43]. It is shown that the turning direction of the catalyst/SiNW is always towards the side with a shorter droplet sideline. According to the HR-TEM characterization in Fig. 6, the continuous turning dynamics, in a crystallographic-index-lowering sequence, as it is energetically favorable, will not trigger any twin formation and thus leaving a high quality mono-like Si lattice in the domains. Rather, when the growth proceeds to a critical point, where the catalyst droplet touches the empty trench region (without a-Si:H coating) produced by itself in the last cycle, the turning direction is forced to reverse. At this moment, the rear deposition interface just rests at the midway of the turning arm, exactly the same place where parallel twin planes are observed in the HR-TEM characterizations in Fig. 6. This seems to indicate a direct link between the twin plane formation and the change of turning direction during the in-plane SiNW growth, which is fundamentally different to those behind the kinking phenomena observed in the gas-feeding VLS growth of SiNWs[22–24, 44, 45].
Then, the mechanical properties of these self-assembly SiNW springs are also tested and shown in Fig. 7, where a single spring segment has been picked up by using a nano-manipulator and mounted between an AFM cantilever and a probe tip[46, 47]. The zigzag spring was subject to tensile stretching up to 12%, but showing very linear stress-strain response as seen in Fig. 7(b). This kind of testing has been repeated several times until a rupture happened at a turning corner of the zigzag SiNW, not in the spring segment. This greatly enlarged stretchability of 12% has been recorded with a rather thick SiNW of around 200 nm in diameter, which is supposed to break easily under stretching < 1% [16]. Therefore, an order of magnitude enhancement has been achieved thanks to the 2D spring geometry engineering. Meanwhile, in view of practical device applications, a thicker SiNW channel is also advantageous in order to carry a high current to drive the subsequent fan-out circuits. Compared to the top-down fabrication, such a self-automated bottom-up growth of regular zigzag Si nanowire springs represents a rather simple, low cost and low temperature nano-structuring method that requires no high resolution lithography nor multi-steps processing. Importantly, this self-assembly growth leads to a high crystal quality and naturally strain-relaxed turnings without any pattern-etching or buckling damages. The only drawback for this self-automated line-shape engineering is the random distribution of the in-plane SiNWs, which are hard to position and align for device implementation. Solution to address this issue has to resort to a more precise growth control of the in-plane SiNW springs, as will be introduced in the following sections.

class="figure_img" id="Figure7"/>
Download
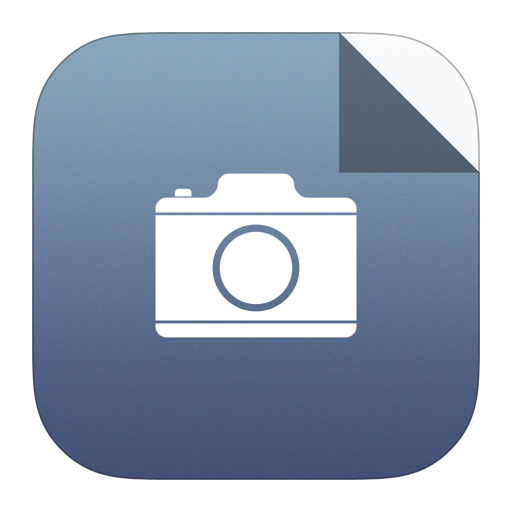
Larger image
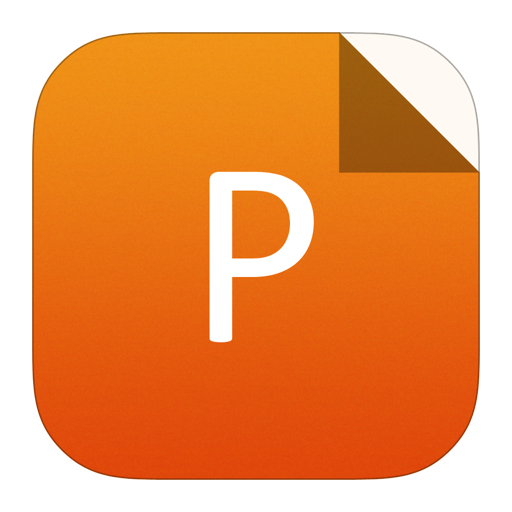
PowerPoint slide
Figure7.
Stress-strain testing of the zigzag SiNWs spring: (a) SEM image of in-situ stress-strain testing setup with of a segment of zigzag SiNWs mounted on the tip of a nano probe and to the end of a cantilever, while (b) shows the linear elastic response[42] .
4.
Guided growth of in-plane SiNWs
Though the SiNW springs above have demonstrated a successful line-shape engineering that imparts extra stretchability to c-Si nano channels, a more deterministic and reliable geometric control is still required to enable a reliable, and yet scalable, batch-manufacturing and device implementation. Fortunately, the in-plane growth mechanism of SiNW has another unique capability to offer, that is, the growth routine of the SiNWs led by a surface-running catalyst droplets can be precisely controlled or guided by pre-design simple step edge[48–51]. Indeed, this guided growth strategy resembles very much, as depicted schematically in Figs. 8(a) and 8(b), to the applying of rulers or compass in our daily lives to draw straight lines or regular circles on paper sheet. The only difference is that we have to apply a little pressure to press the pencil tip against the rule edges to ensure that it will follow the edge track to draw a straight line, while here the running catalyst droplet is actually attracted by the extra a-Si supply on the vertical sidewall of step-edge to go along the step, and thus produce in-plane SiNWs exactly at the root of the step edge. It is worthy to note that the simple step-edges are easy to pattern without the requirement of any high resolution lithography, and more importantly, the geometry of the step edge line can be easily programmed, in principle, into whatever 2D single-touch-draw pattern. This guided growth control thus hints at a deterministic and even programmable line-shape engineering of the in-plane SiNWs into extremely stretchable 2D spring shapes.

class="figure_img" id="Figure8"/>
Download
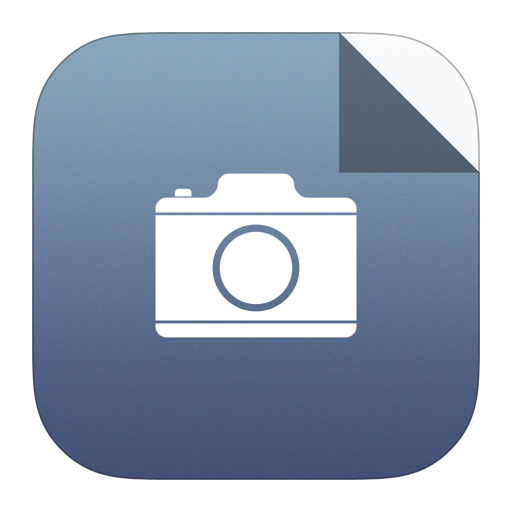
Larger image
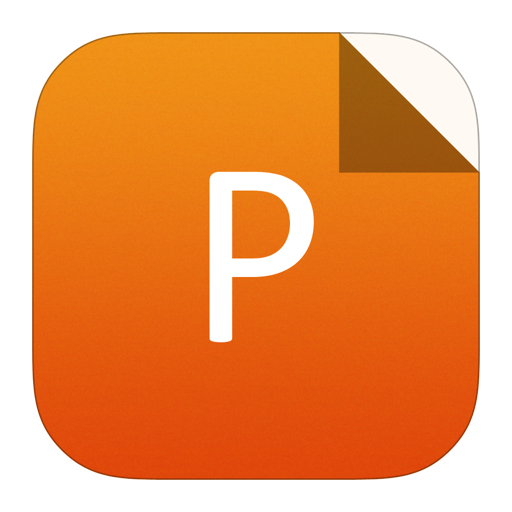
PowerPoint slide
Figure8.
(Color online) (a) and (b) show a comparison of the guided growth of in-plane SiNWs, with the aid of simple step edge to the drawing of straight lines or regular circles with the use of rulers and compass. The SEM images show that, while the free SiNWs are irregular, the guided SiNWs faithfully follow the straight and turning edges of SiNx steps. (c)–(e) illustrates the implementation steps of the guided growth of SiNWs, where the step edge is provided by patterning SiNx dielectric layer crossing the indium stripes. Adapted from Ref. [48].
To implement the step-edge guided growth, parallel guiding edges will be first formed by etching 80 to 180 nm into the underlying SiOx or SiNx dielectric layer, as illustrated in Fig. 8(c), which are lying crossing to the pre-patterned In or Sn stripes. Then, standard in-plane growth procedure will be carried out that includes usually the H2 plasma treatment to form discrete catalyst droplets (now lying close to the guiding edge crossing the metal stripes), the a-Si layer coating as precursor, and at last the annealing growth of in-plane SiNWs following the guiding edge lines.
As explained above, during an in-plane growth of SiNWs, the liquid In droplet could be subject to a strong interface interaction force, caused by the relative position of the front and the rear solid/liquid interfaces, which can deform the droplet from its initial sphere shape (that is the energetically favorable geometry with the minimal surface energy of Gmin). Any geometric deformation can be described as adding an extra Gibbs surface energy (δG) with respect to that of a spherical one. The introduction of a step-edge, coated with a layer of a-Si:H on both the planar and sidewall surfaces, will also alter the local growth balance condition for the catalyst droplet, or in view of surface energy, the Gibbs surface energy profile seen by a catalyst droplet growing along the step edge is different. This differentiated energy profile can thus drive the catalyst droplet to follow, or be repelled from the step-edge under different contacting configurations. In seeking a reliable guided growth and thus line-shape engineering of in-plane SiNWs, a stable guided growth along the step-edge is desirable. While a detailed formulation of the extra Gibbs surface energy seen by the catalyst under different contacting configurations, for example the 4 distinct contact situations depicted in Fig. 9(b), cannot be addressed systematically in this review, a comprehensive theoretical modeling and experimental verification has been presented in the previous work[48]. Here, we only recount the most relevant concave (CC) contact situation, as depicted in Fig. 9(b), where the droplet contact to both the bottom and the sidewall interface. In this situation, the overall Gibbs energy of the concave contact has been rewritten as
$${G_{{ m{cc}}}} = left( {{w_{ m{c}}} + {h_{ m{c}}}} ight){l_{ m{c}}}left( {{sigma _{ m{c}}} + {sigma _{{ m{in}}}}} ight) + 2{w_{ m{c}}}{h_{ m{c}}}{sigma _{ m{c}}}, $$ ![]() | (2) |
where wc, hc and lc are the width, height and length of the leading catalyst droplet, σc and σin are the surface energy of catalyst and the interface energy between catalyst and substrate, respectively. Note that, in addressing a realistic catalyst droplet deformation, the width and the height of the catalyst droplet are not necessary the same as assumed in Eq. (1). On the other hand, the mass conservation condition demands that
m c}}{h_{
m c}} = left( {{w_{
m c}} + {h_{
m c}}}
ight){h_a}$

$$G_{ m {cc}}^{ m {min}} = frac{{Vfleft( {{h_{ m a}} - f{h_{ m c}}} ight)left( {{sigma _{ m c}} + {sigma _{ m {in}}}} ight) - 2h_{ m a}^2h_{ m c}^2{sigma _{ m c}}}}{{{h_{ m a}}left( {{h_{ m a}} - f{h_{ m c}}} ight)}},$$ ![]() | (3) |
with
m c}}{w_{
m c}}{h_{
m c}}$

Similarly, the Gibbs surface energy analysis and formulation can also be carried out for the other three planar, convex-corner and striding-over-contact situations, as depicted in Fig. 9(b) (See Ref. [48] for more derivation details). For a typical a-Si:H coating layer thickness of ha = 15 nm, the variation trends of the minimum surface Gibbs energies
m flat}^{
m min}$

m cc}^{
m min}, G_{
m cv}^{
m min};{
m {and}};G_{
m so}^{
m min}$

m flat}^{
m min}$

m cv}^{
m min}$

m cc}^{
m min}$

m c}}/2 gg {h_{
m s}}$


class="figure_img" id="Figure9"/>
Download
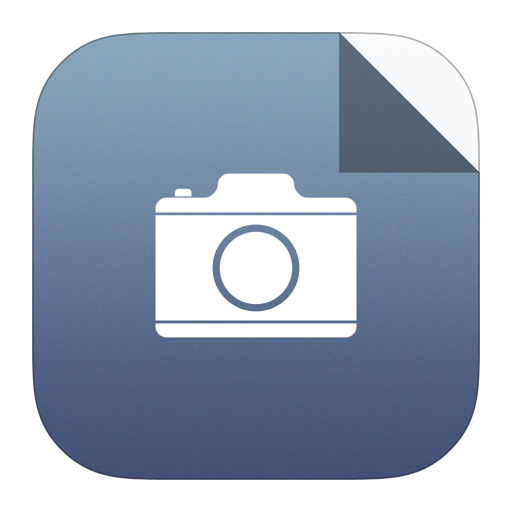
Larger image
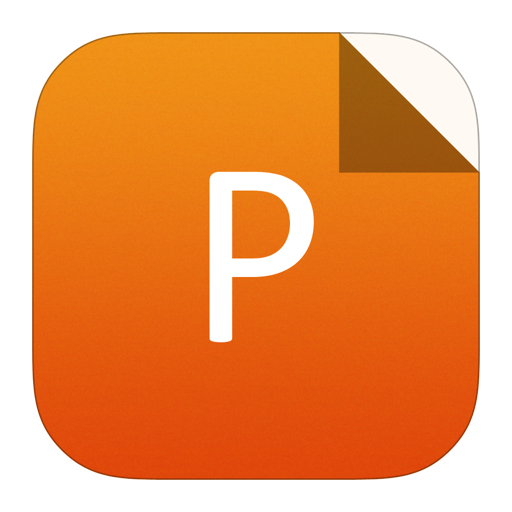
PowerPoint slide
Figure9.
(Color online) (a) shows the key dimensions of a liquid catalyst droplet in a SEM image, while (b) depicts the 4 different contact profiles during a guided growth along simple step edge. (c) plots the evolution of the surface Gibbs energy of an In droplet with four different contacting profiles at the step edge. Adapted from Ref. [48].
This scalable guided growth of in-plane SiNWs has been first explored to batch-manufacture orderly parallel SiNWs array for field effect transistor (FET) application, with a particular perspective for high performance thin film transistor (TFT) in flat panel display[52]. Fig. 10(a) shows a 45°-tilted SEM image of an in-plane SiNW grown along the simple step edge, with a bright In catalyst droplet detached from the SiNW tip during the final cooling. Top SEM view of the SiNW array is presented in Fig. 10(d), where the vertical thicker lines are the In stripes, while the pre-defined guiding edge lines are patterned in perpendicular direction. A series of zoom-in SEM images in Fig. 10(d) reveal tiny SiNWs grown at the roots of the step lines, with a diameter of around 70 nm and a full length larger than 50 μm. Importantly, at each guiding side line, there is always one and only one SiNW, with a remarkably high success-rate > 98% as confirmed by examining 100 different places over one inch wide sample. These precise alignment, positioning and channel number controls are all crucial aspects for industrial manufacturing and device applications. More importantly, despite of a low temperature growth (< 350 °C), the SiNWs boast still a high crystallinity as confirmed by transmission electron microscopy (TEM) characterizations presented in Figs. 10(e)–10(g). According to the high-resolution TEM lattice image and corresponding diffraction pattern, the in-plane SiNWs are usually aligned to Si <211> direction.

class="figure_img" id="Figure10"/>
Download
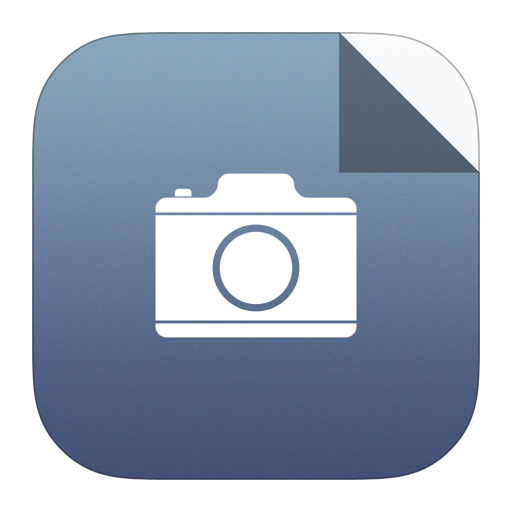
Larger image
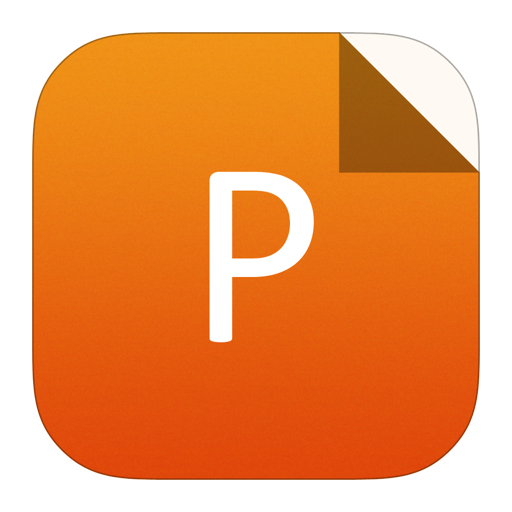
PowerPoint slide
Figure10.
(Color online) (a) SEM image of an in-plane Si nanowire (SiNW) channel, led by an indium (In) droplet ahead. (b) presents the transfer properties of the devices measured at different VDS conditions, while the hole mobility in the SiNW channel under VDS = 1.0 V is extracted and plotted against VGS by the pink line. (c) Optical transmission spectra of the clean SiNWs after remnant a-Si:H etching, compared to those of the reference glass substrate, with a-Si:H coating and SiNWs without remnant a-Si:H etching, while (g) shows an optical image of the transparent poly-Si channels grown on glass substrate. SEM characterizations of the self-aligned poly-Si nanowire channels along the step edge lines are shown in (d), while a series of detailed HR-TEM analysis of a single nano-channel, transferred and mounted onto a copper grid are presented in (e)–(f). Adapted from Ref. [52].
In order to testify the electrical properties and device performance of the in-plane SiNWs, top-gated Fin-TFTs were fabricated by choosing 30 neighbouring and parallel poly-Si nano-channels in a group to serve the channel for TFTs. A 30-nm-thick Al2O3 dielectric is grown by atomic layer deposition (ALD). The transfer curves of the poly-Si channel-based Fin-TFTs measured at different source–drain voltage (VDS) conditions are shown in Fig. 10(b), which indicates a p-type channel characteristics due to the dissolution and incorporation of indium catalyst atoms into the poly-Si channels that gives off p-type doping in crystalline silicon[53]. Under negative gate voltage (VGS), an accumulation of hole-carriers is induced in the semiconducting SiNW channels, and thus allowing a high current on-state in the nanowire channels. Remarkably, a steep subthreshold swing (SS) of about 163 mV/dec has been achieved at gating voltage of VGS = ?0.6 V, with an on/off current ratio of 6 × 105 under VDS = 1.0 V, which remains very stable with varying VDS. The hole mobility of the SiNW TFTs is calculated according to the trans-conductance equation dI/dVGS = μ (C/L2)V, where μ is the mobility of holes, C the gate-channel capacitance and L the length of SiNW. The capacitance is approximated by C = εS/d, where ε is the dielectric constant, S and d are the total channel areas and the thickness of Al2O3 gate dielectric layer, respectively. The hole mobility of the TFT device operating at VDS = 1.0 V is calculated and plotted as pink line in Fig. 10(b), which shows that the hole mobility in the poly-Si nano-channels is higher than 90 cm2V?1s?1 in the range of VGS = ?10 to ?8 V, with a peak hole mobility exceeding 100 cm2V?1s?1 at around VGS = ?7 V. Such a high hole mobility is closely related to the crystalline nature of the poly-Si nano-channels. More excitingly, the precise arrangement of the poly-Si nanowire channels, along with the selective etching of remnant a-Si:H thin film, makes them transparent to visible light and thus ideal for implementing transparent TFT circuitry. This can be inferred from the transmission spectra of samples of clean glass substrate, a-Si coated substrate and SiNWs with or without remnant a-Si etching after SiNW growth. It is remarkable that the SiNWs array alone do not hinder the passage of line, which is reasonable as the diameter of the SiNWs is only ~70 nm which is much smaller compared to the visible wavelength of 400 to 700 nm and thus the incident light can easily scatter around the SiNW array without many optical losses. These results could indicate a new high mobility, low temperature and readily scalable SiNW TFT technology for high performance flexible and transparent macroelectronics and displays.
5.
Deterministic line-shape engineering for stretchable electronics
The line-shape engineering of SiNWs represents a unique dimension of control in the 1D nanostructures, which can be accomplished on a 2D plane that is compatible to the planar electronic architecture. This is the very basis for achieving a new generation of scalable, reliable and integratable Si-based device applications, particularly for high performance thin film or large area electronics. Though the elastic line-shape design, in a form of zigzag or serpentine geometry, has been proposed and explored to pattern stretchable metal connection or c-Si ribbons etched out of the buried c-Si layer in SOI wafer, the full potential of this 2D engineering strategy is still limited by the spatial resolution of conventional photo-lithography. An ultimate solution for implementing a fully stretchable c-Si electronics could rely on a direct line-shape engineering of c-Si channel into nano springs with tiny diameter (for example in the range of 40 to 120 nm). But this seems to pose a formidable challenge to the conventional lithography fabrication.
Now, based on the precise location and grow routine control of the in-plane SiNWs, high quality c-Si channels can be grown continuously out of surface moving liquid catalyst droplets at only 350 °C, in a way quite similar to the crystal pulling out of molten Si at > 1400 °C (as depicted in Fig. 1(a)). Interestingly, the maneuverable movement of the catalyst droplets provides an unprecedented opportunity to grow the c-SiNW into desirable line-shape and place them exactly in the right places of need for subsequent electric connection, devising or transferring to flexible and stretchable substrate. Fig. 11 illustrates the fabrication procedures of manufacturing line-shape-engineered in-plane SiNWs, which are basically the same as those in guided growth of SiNWs for FET in Figs. 8(c)–8(e), except that here the guiding step edge lines are designed into serpentine shape, as a programmable template to shape the as-grown SiNWs. The typical SEM images of the in-plane SiNW springs are shown in Fig. 12. The thicker bright lines in Fig. 12(a) are the In stripes that provide the catalyst sources to form discrete In droplets upon reductive H2 plasma treatment. Then, upon the annealing in vacuum, the in-plane SiNWs grow from the In stripes and advance following the guiding edge lines faithfully, and thus duplicate the spring shape of the pattern design as seen in the close examination presented in Fig. 12(b). Remarkable, this guided growth line-shape engineering is also applicable to form basically any fractal or one-touch-draw patterns, as witnessed in Figs. 12(c)–12(d). This thus lays a fundamental basis to unleash the potential of 1D nanowire structure for novel functionalities. More experimental details, discussion and analysis are available in the Ref. [54].

class="figure_img" id="Figure11"/>
Download
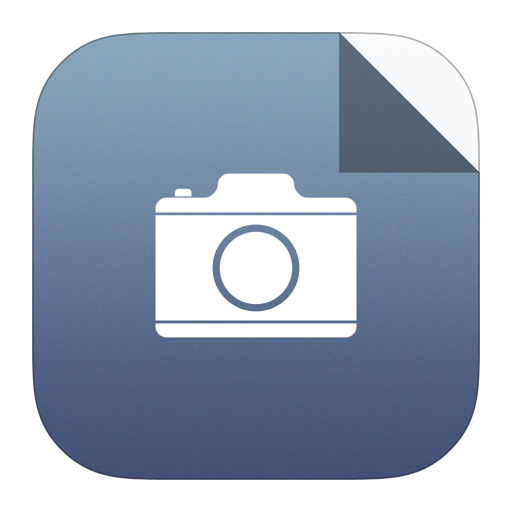
Larger image
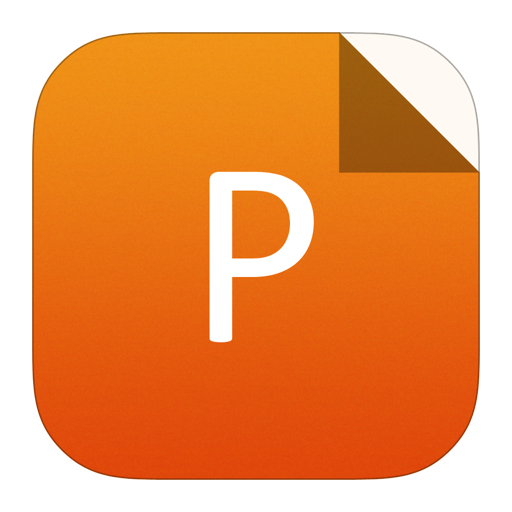
PowerPoint slide
Figure11.
(Color online) (a)–(d) show the fabrication procedure of line-shape engineered in-plane SiNWs.

class="figure_img" id="Figure12"/>
Download
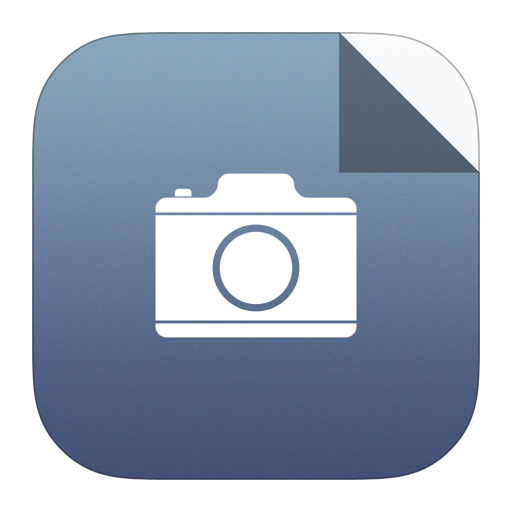
Larger image
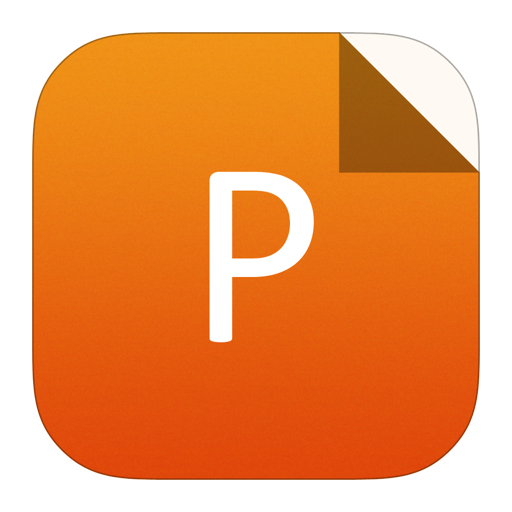
PowerPoint slide
Figure12.
(a)–(d) show the typical SEM images of the in-plane SiNWs grown into different step edge profile. The bright and thick vertical lines in (a) are the In stripes, which provide the source catalyst droplet formation. (d) shows a particular case that a single in-plane SiNWs grow to encompass an discrete island and leave it at the end following its entry path.
In order to testify the mechanical and electric properties of the SiNW spring channels, a portion of the SiNW spring segment, as seen in Fig. 13(a), has been picked up by nano-probe in SEM and mounted to another probe for stretching or compressing testing. As seen in Figs. 13(a) and 13(b), the SiNW spring with an initial length or span of 76 μm can be easily stretched to more than 140 μm, equivalent to subject to a tensile strain of > 80%, while still preserving an integral and elastic c-Si channel connection. According to the finite elemental analysis in Figs. 13(d) and 13(e), the maximum von Mises stress accumulated along the SiNW spring, with a diameter of 100 nm, is less than 25 MPa, which is much lower than the fracture limit of c-Si which is typically 2 to 10 GPa[16, 55]. As a consequence, the line-shape engineered SiNW can behave like an extremely soft but resilient spring, which is predicted by simulation to deliver a linear mechanic response following typical Hooke’s law and with a very low stiffness of k = 4.3 × 10?5 N/m, as shown in Fig. 13(g). Furthermore, primitive electric transport measurements were also carried out to verify the conductivity of the SiNW springs. The I–V curves recorded under different stretching or compressing (squeezing) status are shown in Fig. 13(f), where the poor electronic contacts resulted from the non-ohmic contact bonding between the SiNW spring and the holding tungsten probes. This is because, in this experiment, the SiNWs are fixed to the probes only by the amorphous carbon (a-C) coating in the SEM environment, not by any metal contact. Nevertheless, these results prove that the elastic SiNW spring channels are indeed conductive under large tensile strain loading, which is a critical foundation for future electric device applications.

class="figure_img" id="Figure13"/>
Download
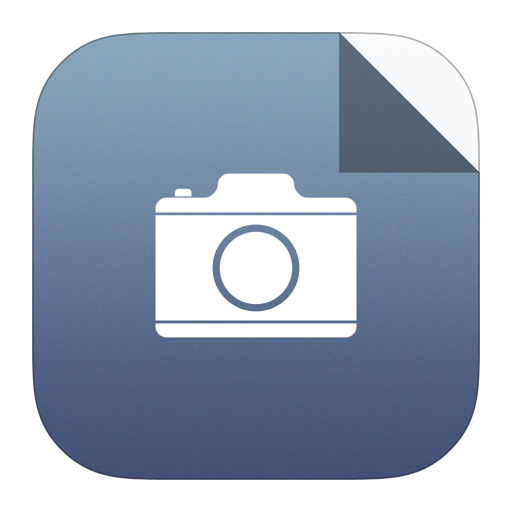
Larger image
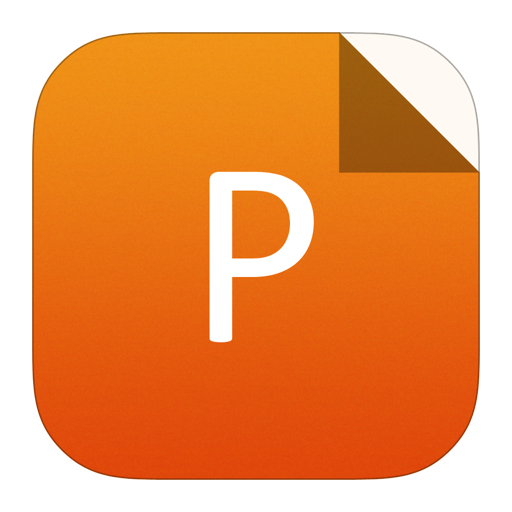
PowerPoint slide
Figure13.
(Color online) [54] (a) SEM image of the in-plane SiNW spring, where a segment has been successfully picked up and transferred by a tungsten nano-probe to mount on both end, for mechanical testing. (b) and (c) show the SEM images of the initial and the stretched SiNW spring, while (d) and (e) present the mechanical analysis of the strain distribution in the SiNW segment. (f) shows the I–V characteristics measured on the c-Si nano spring channels, under squeezing or stretching states. The electric contacts between the SiNW spring and probe were realized by simple amorphous carbon coating. (g) shows the simulated force-displacement relationship of the SiNW spring.
6.
Conclusion and perspectives
In-plane solid-liquid-solid (IPSLS) growth of SiNWs brings in two exciting opportunities to engineer the line-shape of 1D c-Si channels into elastic 2D springs: one is based on the strong interface interaction that leads to a unique growth balance dynamics and periodic zigzag SiNW line-shape, while the second is enabled by a deterministic guided growth of in-plane SiNWs along step edge lines with pre-design profile. Both of them hold a promise to develop highly stretchable c-Si electronics, but the latter one boasts a particular advantage that could enable a precise batch-manufacturing of regular SiNW spring, as a basis for implementing large scale stretchable electronics with a low cost and high reliability. The mechanical properties, as well as primitive electric transport, of the tailored SiNW springs are also presented. This in-plane growth and engineering strategy could help to extend the mature c-Si technology into the development of a new generation of high performance stretchable sensor, display and logic electronics.