1.
Introduction
Fiber-shaped materials have recently been considered potential candidates for smart textiles, wearable electronics, microprobes, and flexible electronics and sensors, among other devices. Micro- or nano-structured fiber materials minimize the fabrication challenges posed by their individual, small, constituent components while preserving their components’ intrinsic properties[1]. The definition of hybrid functional microfiber can be stated as hybrid functional microfibers belong to a kind of flexible biomaterials to create varieties of functional template containing one dimensional to three-dimensional features with various components including metal oxides, ceramics, alginate, chitosan, graphene oxide and nanotubes etc. By taking advantage of fiber materials, researchers have developed various fiber-based devices. In addition, with the development of flexible electronics, devices such as lithium-ion batteries (LIBs), flexible solid-state supercapacitors (SCs)[2–4], foldable displays[5], and soft photodetectors[6, 7], which are considered promising candidates for advanced devices, have also been widely improved to satisfy the requirements for lightweight and flexible devices[8–18]. One of the remarkable features of fiber-based devices is that they can be weaved or integrated into yarns of different colors to form a large variety of complex, two-dimensional (2D), or three-dimensional (3D) patterns without sacrificing their deformability. These fibers and textiles have also been considered potential substrates for low-cost flexible electronics and multifunctional fabrics, commonly referred to as electronic fibers (E-fibers) or electronic textiles (e-textiles or smart textiles)[19, 20]. In addition, E-fibers or e-textiles are attracting attention for use in wearable devices, which are garments with embedded electric devices. Fiber electronics, which refers to electronic devices on textile fibers, are a critical component for wearable technology, particularly for future portable and wearable electronics[21–23]. The ability to produce electronic components such as transistors[24–26] and displays[27] and energy storage and harvesting devices such as solar cells[28–30], thermoelectric generators[31], batteries[32, 33], and capacitors[34, 35] on fiber electronics is not an essential requirement for future wearable devices with integrated systems.
The progress of electronic skin (e-skin) is the basic requirement for the intuition of artificial intelligence that direct contacts with humans as well as biomedical application. The e-skin, which can mimic pressure, humidity and temperature sensing capabilities of human skin, has obtained great attention due to its superior abilities to detect slight pressure changes[36–39]. It has probable application in human health monitoring, medical diagnostics, tactile sensor, and artificial intelligence etc. Pressure sensor can perform as transducers that convert the changes in external force to an electrical signal or their recognized signal is a fundamental part of e-skin[40–43]. Lou et al. also fabricated a self-assembled 3D films platform that combines viscoelastic property materials with an electrically conductive material rGO (reduced graphene oxide)[44]. They introduced a simple and efficient way to fabricate rGO nanosheets wrapped P(VDF-TrFe) (Poly vinylidene fluoride trifluoroethylene copolymer) nanofibers (NFs) (PVDF@rGO), forming into a uniform 3D networks conducting film, which can be used to fabricate flexible pizeoresistive pressure sensor. It can be also acted as high performance e-skins to measure other complex forces like bending, torsion, human physiological signals, ranging from blood pressure pulse and the muscle movement. These admirable sensing properties allowed the device to monitor human body's signal (pulse and muscle movement) with the potential applications for monitoring of disease and speech recognition. Similarly, Sun et al. also reported that flexible tactical sensor is key element of electronic skin, which can simulate the comprehensive properties of human perception of electronic products[45]. Till now, lots of reports have been published on the understanding of a high performance large-scale tactical sensor arrays employing novel microstructures and electronic materials. Recently, Lou et al, fabricated a ultrathin, flexible multimodal sensing capability of e-skin sensor, which is based on polyaniline hollow nano spheres composite films (PANI-HNSCF)[46]. These unique properties of the polyaniline hollow nanospheres (PANI-HNSs) act as an active component for the piezo-resistive pressure-sensor. Similarly, Park and Jang reported a simple fabrication method for the development of a new class of graphene/free-standing nanofibrillar PEDOT/P (VDF-HFP) nanohybrid e-skin device. This e-skin exhibits enhanced contact surface area and piezo-resistivity with an extremely high gauge factor (320) under tensile strain, which combine to yield very high sensitivity to pressure (limit of detection = 0.5 Pa)[47].
Fabricating textile electronics entails using conducting and/or semiconducting materials to impart textile fibers with electronic capabilities. Conventionally, metallic wires have been usually used as a core material to operate transistors and energy converters[48]. Although metallic fibers have more advantages as a core electrode because of their high conductivity and availability, their disadvantages such as affinity to oxidize under ambient conditions, high density, and poor bendability limit their use in wearable electronics. By comparison, hybrid fiber materials could have electrical functions in addition to excellent mechanical deformability of the fibers themselves. Other functionalities can also be added to metal wires to produce fibers with computational properties[49]. Thus far, fiber-based electronics have paved the way for a revolution in practical and socioeconomic fields because they can be imparted with various functionalities via simple methods[50]. During the past few decades, carbon-based nanomaterials, composites with metallic nanomaterials, and biomaterials have offered additional prospects toward the development of fiber electronics with improved properties. E-fibers based on carbon nanomaterials and composites with metallic nanomaterials enable the fabrication of electronic fibers with both mechanical deformability and electrical conductivity. Carbon nanomaterials such as graphene, carbon nanotubes, and carbon nanoparticles exhibit outstanding properties for electronic fibers with excellent mechanical strength, low weight, and good electrical conductivity. In addition, through hybridization with other nanomaterials, the performance of carbon-nanomaterial-based E-fibers can be further improved[51–58]. In addition, composites with metallic nanomaterials also show extraordinary performance as a result of optimized mechanical properties of the fibers and electrical properties of the metal. By embedding nanoparticles or coating metallic films using the layer-by-layer method, metallic nanomaterials can be embedded as fillers into the polymer matrix of fibers, resulting in a metallic-nanomaterial-based composite fiber[58–67]. Furthermore, various metals can be used to functionalize the electronic fibers. Electronic-fiber-based biomaterials, which have potential applications in the biomedical field, are being used as nanobiochip materials, interface biomaterials, nanobiosensors, nanomotors, and nano-drug-delivery systems. The biomaterial-based fibers obtained when biopolymeric materials are used as fibers or when a hydrogel technique with nanowires is used have potential applications in the biomedical field[68–71].
In this review, in Section 2, we briefly introduce the recent developments in fiber-shaped materials, with particular focus on the fabrication of flexible fiber electrodes and on the structural design of devices that can be used as electronic textiles. We also overview the possibility of combining nanofiber and medical technologies. The implications of integrating nanofiber technology into biomedical applications such as drug delivery, tissue engineering, and bone regeneration are also discussed. In Section 3, we summarize the development of various key components required for e-textiles as wearable devices. The most fundamental element is the conductive textile, which is required for interconnection to transfer power and data. We expect this article to encourage relevant research in the field so that the hybrid cell can be distinct and disciplinary in the field of medical science and technology.
2.
Classification of hybrid functional microfiber
2.1
Nanofibers composed of metals, metal oxides, ceramics and composites with organic polymers
The preparation of nanofibers is generally comprised of pure metals respectively, ceramics or composites of such materials with organic polymers. These types of nanofibers exhibit in general specific electronic, photonic and magnetic properties. For examples nanofibers displaying properties such as fluorescence, electroluminescence, enhanced ion or electron conductivities, photovoltaic effects, photocatalytic effects, ferromagnetism, super paramagnetism. Applications of such nanofibers investigated in the literature and reviewed include optical and electronic sensors, lithium ion batteries, fuel cells, solar cells, as well as energy harvesting and storage systems. Main reasons for choosing nanofibers as elements for such applications are the one-dimensional nature of the nanofibers affecting opto-electronic properties, the large specific surface coming with the nanoscale, short electronic diffusion distances across the fiber diameter.
2.2
Electrospun fibers exhibiting specific photonic and magnetic functions
Nowadays, one dimensional nanostructures displaying photoluminescence, and electroluminescence have brought excellent attention in particular in the view of potential applications in light emitting diodes, full colour displays, lasers, data storage[72].
2.2.1
Luminescent based hybrid functional nano fibers
Luminescent polymer nanofibers with diameters in the range from 250 to about 750 nm were fabricated from binary blends of a set of conjugated polyfluorene (PFO) derivatives with PMMA[73]. Homogeneously mixed nanofibers were observed for lower concentrations of the PFOs while core shell fibers were found for higher PFO concentrations. Phase separation caused by the lower solubility of PFOs in the common solvent was also suggested to be the origin of the core shell formation. Strong blue, green, yellow and red luminescence was observed depending on the type of PFO derivative. Blends were also considered for the conjugated polymer poly (2-methoxy-5-(2-ethylhexyloxy)-1,4-phenylene-vinylene) (MEH-PPV) with PVP[74, 75].
2.2.2
Polymers nanofibers doped with luminescent components
Components used to induce luminescence in polymer nanofibers include quantum dots as well as rare earth complexes, in specific europium and erbium complexes. Nanofibers prepared from poly(ethylene oxide) containing ZnO (Zinc Oxide) quantum dots showed a significant quenching of the visible emission of the quantum dots[76]. The quenching was attributed to the formation of O–Zn bonds at the surface of the quantum dots. In some other reports, fibers composed of CdSe/ZnS quantum dots incorporated into cellulose triacetate showed a substantial fluorescence already at small concentrations of the quantum dots[77]. Birefringence investigations revealed again a significantly alignment of the polymer chains along the nanofiber axis. To prepare CdS nanocrystals inside electrospun polymer fibers an approach based on the decomposition of cadmium thiolate precursor was proposed[78]. Strongly light emitting fibers are also reported for such composites. PPV/PVA (poly(phenylene vinylene)/ poly(vinyl alcohol) composite nanofibers into which Ag2S nanocrystals were introduced were achieved by spinning a solution containing in addition to PVA a sulfonium precursor of PPV and silver nitrate followed by a conversion step[79].
2.2.3
Inorganic luminescent nanofibers
A significant number of reports have considered inorganic electrospun fibers doped with rare earth complexes such as ones based on erbium (Er), europium (Eu), terbium (Tb), samarium (Sm), cerium (Ce), dysprosium (Dy)[80]. The rare earth metals lanthanides form a group of chemically similar elements, which have an open 4f shell in common. Lanthanides absorb radiation in very sharply defined bands (4f–4f transition). The f–f transitions of free lanthanide ions are forbidden. When the centrosymmetric symmetry of the ion is removed by an asymmetrical external crystal field, the transitions become allowed: the luminescence of complexed lanthanides is enhanced. The number of luminescent nanofibers electrospun from inorganic materials containing Europium complexes is considerable. The main theme is that these complexes control the emission properties to a major extent with modifications coming from the nanofiber matrix.
2.2.4
Nanofibers with specific magnetic properties
One dimensional magnetic nanomaterials are meeting with substantial interest in view of the unique phenomena observed for the case that the geometrical dimensions of the material become comparable to critical sizes specific for each ferromagnetic material (examples: Fe: 14 nm; Ni: 55 nm; Fe2O3: 128 nm). Single domain nanoparticles without domain walls are known to be stable at such sizes with the spins being strongly affected by thermal fluctuations. Isolated nanoparticles show super paramagnetism characterized by the absence of a permanent magnetization and a “hysteresis” with the coercivity being zero. Fibers composed of isolated nanoparticles tend thus to display super paramagnetism while fibers composed of particles with aspect ratios larger than 1 all the way up to single domain rods tend to exhibit ferromagnetism while intermediate states – simultaneous display of superparamagnetism and ferromagnetism can also occur.
2.3
Nanofibers as key elements in optoelectronic devices
2.3.1
Sensors
Nanofibers prepared via electrospinning have specific surfaces approximately one to two orders of the magnitude larger than flat films, which makes them excellent candidates for potential applications in sensors. Nanofiber based sensors are targeted, for this reason, to show much higher sensitivity and quicker responses compared to sensors based on flat films. Nanofiber designs have very frequently been developed to act as sensitive, fast, stable and reproducible gas sensors, which can easily be integrated into a multi-component array[81]. A gas sensor is a device, which detects the presence of different gases in a given area for monitoring environmental pollution focusing frequently on those gases, which might be harmful to humans or animals. Sensor performance features such as sensitivity, selectivity, time response, stability, durability, reproducibility, and reversibility are known to be largely influenced by the properties of the sensing materials used. It is for this reason that a broad range of materials such as semiconductors (semiconducting metal oxides such as TiO2, SnO2, ZnO), organic/inorganic composites, conducting polymers, carbon graphites have been investigated for sensing devices based on electrospun fibers.
2.3.2
Polymer composite sensors
Polymer microfibers encapsulating polydiacetylene (PDA) chain molecules known to be active as chemosensors and photonic materials were prepared via electrospinning and subsequent UV irradiation of self-assembled diacetylene monomers in the fibers yielding blue-colored PDA containing polymer fibers[82]. The feasibility of using PDA-embedded electrospun microfibers as potential sensor materials was demonstrated by fluorescence generation as controlled by specific ligand–receptor interactions. A composite comprising poly (vinylidene fluoride) (PVDF) and poly (aminophenylboronic acid) is reported to display an excellent linear response to the detection of glucose for the concentration range from 1 to 15 mM with a response time of less than 6 s[83]. Composite nanofibers of conducting polymers and hydrophobic insulating polymers, composed of polymers based on pyrrole and PS or PMMA respectively, were prepared and the sensing properties were studied relative to chloroform and ammonia[84]. Similarly, PAN/zinc chloride (ZnCl2) composite nanofibers were also observed to display an enhanced sensing response to hydrogen sulfide[85].
2.3.3
Inorganic composite fibers
Recently, few reports are concerned with ZnO nanofibers and their sensing properties relative to different gases. ZnO nanofibers with an average diameter of 150 nm were reported to exhibit excellent sensing properties against ethanol at an operating temperature of 300 °C, including a rapid response (about 3 s) and recovery (about 8 s) time and a high selectivity[86], ZnO nanofibers to be used in gas sensors for the detection of ethanol were also reported in other publications[87, 88]. The ZnO hollow nanofibers showed improved response to acetone at 220 °C with excellent selectivity and stability, which is attributed to the one dimensional hollow nanostructure. In addition to pure ZnO, SnO2 and TiO2 corresponding composite nanofibers, doped nanofibers were also prepared and their sensing properties were evaluated. Such combinations of nanofibers with other compounds were demonstrated aiming at higher sensitivities and lower driving temperatures. TiO2–ZnO core–shell nanofibers were prepared via a two-step process in which the TiO2 core nanofibers were made by electrospinning with the ZnO shell layers subsequently being prepared using a layer deposition[89].
3.
Strategies toward functional hybrid microfibers: materials and fabrication
Because of their high conductivity and ready availability, metallic nanowires (NW) have usually been used in fiber-based electronic devices. In addition, the development of highly conductive hybrid microfibers for different applications is critical for achieving enhanced mechanical and antistatic properties. Various materials have been explored, including polymer-based, carbon-based, and metal-based materials. Among these materials, carbon-based (carbon fibers (CFs), carbon nanotubes (CNTs), or graphene fibers (GFs)) metal–polymer composite materials are widely used for fabricating conductive fibers. In this section, the techniques for fabricating GFs and the latest developments in various fiber materials with metal–polymer composites are discussed. In addition, fiber materials based on biomaterials with unique functionality for biomedical and tissue engineering are introduced.
3.1
Carbon-based nanomaterials
Carbon-based materials, including CNTs, graphene fibers, and carbon nanoparticles, exhibit extraordinary properties such as high mechanical strength, light weight, good electrical conductivity, the ability to be integrated with various materials, good environmental stability, and superior thermal and electrical conductivity. The properties of functional hybrid fibers largely depend on their fabrication process. In most cases, CNT-based fibers have demonstrated superior mechanical properties and conductivity, whereas graphene fibers have a higher specific surface area and are easier to functionalize or hybridize with other nanomaterials[94].
Current research in continuous spinning carbon nanotube fibers (CNTFs) has revealed the prospect of retaining the exceptional properties of individual CNTs at larger and more practical scales. Various methods such as wet spinning and dry spinning have been developed to introduce tiny CNTs into macroscopic fiber structures[90–95]. When CNTs are assembled into continuous fibers in which CNTs are highly aligned, the resultant fibers exhibit excellent mechanical properties and good electrical properties. Compared with the conductivity of various engineering fibers, that of CNTFs can reach 103 S/cm[96], enabling the CNTFs to be used as electrodes for dye-sensitized solar cells[97, 98] or as promising electromechanical actuators that convert electrical energy into mechanical energy[99]. Recently, Guo et al. fabricated spinnable CNT fibers by first synthesizing CNTs using a chemical vapor deposition method and then applying by a spin-drawing process[100]. These CNT fibers exhibit excellent electromechanical reversibility and reversal torsional actuation after 2400 cycles, which is critical for actuator materials. In addition, tens to hundreds of CNT fibers have been easily twisted into macroscopic fibers, which also exhibit lengthwise contraction and rotary torsion. These spun CNT fibers have become an important material in various fields, including energy, biomedicine, sensing, and electronic engineering.
Materials such as polyaniline (PANI) nanowire arr- ays[101] have been bound to the surface of CNT fibers to improve the electrochemical performance of the corresponding fiber energy storage devices such as super capacitors (SCs). The pure CNT yarn is highly flexible and can maintain its structural integrity after being bent, folded, or knotted hundreds of times. The PANI nanowires are uniform and aligned in the direction perpendicular to the CNT yarn surface. For example, the capacitance of pure CNT yarn-based SCs is only 2.3 mF/cm2. By contrast, after being combined with PANI nanowire arrays, the fiber SCs exhibit a capacitance of 38 mF/cm2[101]. This increase is attributed to the PANI nanowires being uniform and aligned in the direction perpendicular to the CNT yarn surface.
Other methods such as dipping and coating methods have been used to immobilize CNTs on cotton[102]. CNTs were modified with poly(butyl acrylate) using a surface grafting technique, and the resultant composite was applied to cotton fabrics by dipping, drying, curing, and finishing. Another approach involved a polyelectrolyte-based coating of multiwalled carbon nanotubes (MWCNTs)[103]. The authors reported that further improvement of charge transport was achieved through the network of nanotubes because CNTs were modified with poly(butyl acrylate). They speculated that the substantially different electrical transport properties were a result of the uniformity of the nanotube distribution, which strongly affects both the strength and conductivity of the CNT composites.
In particular, GFs, which exhibit high strength and good electrical and thermal conductivities, represent another fiber electrode material of practical importance. GFs not only provide mechanical flexibility for textiles, but also possess desirable properties such as being lightweight and easily functionalized compared with conventional carbon fibers[104–106]. In addition, hybridization of GF-based electrodes has been studied to improve their performance. For example, bismuth oxide nanotubes were mixed with GF as an additional electrochemical component to enhance the capacitance of wet-spun GF-based SCs[107]. Recently, Kou et al. proposed a coaxial wet-spinning assembly method of continuous spun sodium carboxymethyl cellulose (CMC)-wrapped graphene core–sheath fibers[108]. Graphene-based woven fabrics (GWFs) have been fabricated by interlacing two sets of graphene microribbons (GMRs)[109]. Li et al. reported a simple method to fabricate GWFs by intersecting GMRs. The important characteristics of GWFs are high structural integrity and excellent mechanical performance compared with those of polycrystalline graphene films. In addition, they possess good gas/liquid permeability compared with monolayer graphene because of the presence of microholes. In the fabrication process, GWFs are grown by an atmospheric CVD method using copper mesh as the substrate. As shown in Fig. 1(a), the first step involves CVD growth of graphene onto copper mesh. In the second step, the copper mesh is removed with FeCl3/HCl aqueous solution. The third and final step involves the collapse of graphene to form double-layered GMRs. The resultant textile exhibits dimensional stability in both the warp and the weft directions. GWFs have also been synthesized through atmospheric chemical vapor deposition (CVD) using copper mesh substrates consisting of ~60 μm -diameter wires. Fig. 1(b) shows planar optical microscopy and scanning electron microscopy (SEM) images of a copper mesh before and after graphene growth. Optoelectronic properties such as the sheet resistance (Rs) and transparency of the GWF are strongly related to the geometrical parameters such as GMR width and spacing (which is related to graphene coverage density). As shown in Fig. 1(c), the film transparency of a sheet increases with the increasing sheet resistance. Similarly, as shown in Fig. 1(d), the conductivity of the GWFs (500–2500 Ω/sq) increases after treatment with HNO3. Although the resistance values still do not satisfy the criteria for a transparent conductor (e.g., they cannot be used as a replacement for the conventional indium tin oxide (ITO)[109]), the electrical and mechanical characterization of these fibers has shown that these yarns can be used as conductors that enable the operation of electronic devices in more varied forms. These studies demonstrate the feasibility of an electronic textile with both the mechanical and electrical performance of fiber materials and pave the way for future complete integration between electronics and textiles.

class="figure_img" id="Figure1"/>
Download
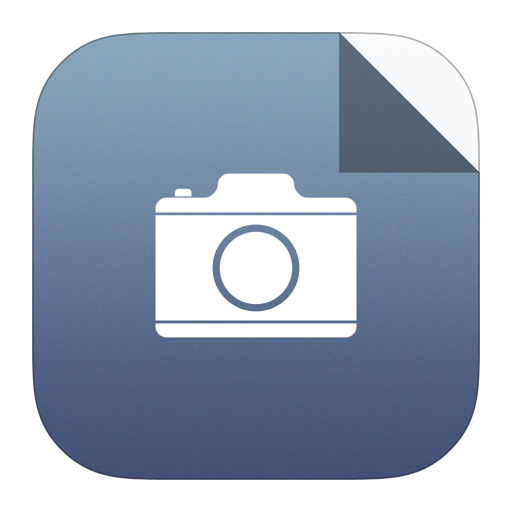
Larger image
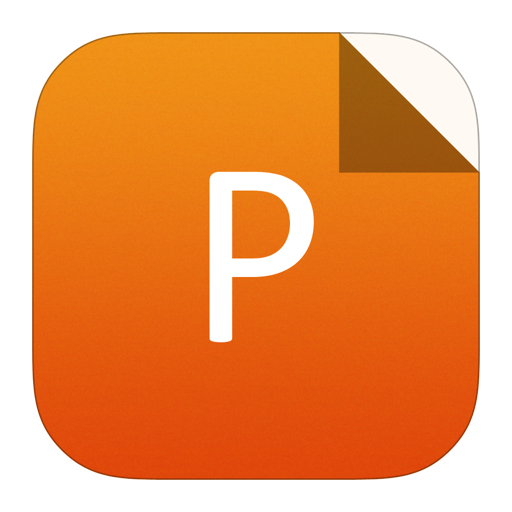
PowerPoint slide
Figure1.
(Color online) Fabrication of GWFs by CVD using copper wire meshes as substrates. (a) Schematic of the steps for GWF preparation. (b) Macroscopic optical images (left), top-view SEM images (right) of copper meshes before (top) and after (bottom) graphene growth. Scale bars, 200 mm. (c) Ultraviolet–visible–near infrared transmission spectra and (d) transparency (at 550 nm) versus sheet resistance plots. Reproduced from Ref. [109] with permission by Nature Publishing Group, Copyright 2012.
3.2
Metal–polymer composites
Compared with pure polymer matrices, polymer-based composites with a small percentage of rigid (metallic) fillers can exhibit significantly enhanced mechanical, thermal, and barrier properties. Moreover, these improvements can be attained through conventional processing techniques without adversely affecting the processability, appearance, density, or aging performance of the matrix. Nanosized particles of Pd/Pt, Ag, and Fe can be applied to textiles to impart antibacterial activity, conductive/magnetic properties, and remote heating capability. Silver nanoparticles can be applied to textiles via a padding method, resulting in textiles with good laundering durability[110]. Lee et al. have reported that polyester nonwoven fibers treated with nanosized silver colloids and silver nanoparticles exhibit good bacteriostatic properties[111]. Mattana et al. developed a novel technique for modifying natural cotton fibers using gold nanoparticles. This process was used to fabricate two types of fiber materials: passive fiber materials such as resistors obtained from electrically conductive cotton yarns and active fiber materials such as organic electrochemical transistors (OECTs) and organic field-effect transistors (OFETs)[112]. These materials might lead to of a fully textile circuit, including passive and active elements, and paves the way for future complete integration between electronics and textiles.
Nanoparticles of TiO2, ZnO, Al2O3, and MgO are a group of metal oxides that possess photocatalytic ability, electrical conductivity, UV absorption, and photooxidizing capacity against chemical and biological species. Intensive research involving nanoparticles of metal oxides has been focused on antimicrobial, self-decontaminating, and UV-blocking functions for both military protective equipment and civilian health products. Nylon fibers reinforced with ZnO nanoparticles can provide a UV shielding function and exhibit reduced static electricity of the nylon fibers. A composite fiber with nanoparticles of TiO2/MgO exhibits self-sterilizing behavior[113]. TiO2 and MgO nanoparticles can be incorporated into fiber materials via the spinning process or via normal textile finishing methods, with the resultant material exhibiting chemical and biological protective performance. Furthermore, cellulose fibers composited with metal oxides (such as TiO2) via in situ synthesis can be used as a catalyst in fuel cells[114].
Foldable nanopatterned wearable triboelectric nanogenerators are considered key materials for the textile industry. Kim et al. has demonstrated silver-coated textile and poly(dimethylsiloxane) (PDMS) nanopatterns based on ZnO[115]. The nanopatterned structures produced 120 V (open-circuit voltage, Voc) and 65 μA (short-circuit current, Isc), and four-layered triboelectric nanogenerators produced 170 V (Voc) and 120 μA (Isc). No significant drift was observed after 120 000 cycles. Similarly, Kwon et al. reported the development of carbon-activated cotton threads on textiles for energy generation[116]. ZnO nanowires and discharge films were incorporated into a textile to hybridize the electrostatic and the piezoelectric effects. Recently, pristine soft conductive yarns were produced via a twist-bundle drawing technique[117]. In addition, conductive yarns modified with reduced graphene oxide (rGO), MnO2 nanosheets, and polypyrrole (PPy) films were used to fabricate weavable supercapacitors. The weavable supercapacitive fabrics have been incorporated into garments. Cui et al. reported a garment prepared using cotton yarns coated with nanolayers of poly(3,4-ethylenedioxythiophene) poly(styrenesulfonate) (PEDOT: PSS) incorporated over an array of gold nanoparticles. These nanolayers imparted the cotton yarns with electrical conductivity and transferred energy from solar cells attached to the exterior of the garment[117].
Nanoparticles are commonly applied to textiles by coating methods involving a particular composition of nanoparticles, a surfactant, ingredients, and a carrier medium. Coating techniques such as spraying, dipping, and washing modify the surface characteristics of the textiles. Textiles are widely considered a universal interface and substrate for the integration of nanomaterials, electronics, and optical devices. Such integrated materials and technologies represent a platform with the ability to respond to mechanical, chemical, electrical, thermal, optical, or magnetic stimuli. Such wearable devices can include sensors and data transmission and processing units. These engineered materials can be seamlessly integrated into garments and be flexible and comfortable while triggering no allergic reaction by the body.
3.3
Biomaterials
Bionanomaterials represent a new, exciting field recognized as a new interdisciplinary frontier in the life and materials sciences. Great advances in nanobiochip materials, interface biomaterials, nanobiosensors, nanomotors, nanocomposite materials, nanoscale biomimetic materials, and nano-drug-delivery systems have led to enormous prospects for bionanomaterials in industrial, defense, and clinical medicine applications.
A biosensor typically consists of a transducer in combination with a biologically active molecule that converts the biochemical response into a quantifiable signal. In general, a biosensor comprises three basic components: (i) a detector, (ii) a transducer, and (iii) a signal processor. The transducer can be electrochemical, optical, acoustic, or calorimetric type depending upon the type of diagnosis and the physiochemical character of the analyte[118, 119]. Biosensors have been largely studied on the basis of various detection principles, including conductometric, amperometric, potentiometric, and voltammetric principles[120]. The selection of the biomaterial when designing a biosensing element is an important issue. DNA/RNA[121], aptamers[122, 123], antibodies[124], recept- ors[125], organelles[126] and animal cells/tissues[127, 128] have been widely used to develop different types of sensing systems. Bioimaging can be considered as the extrapolation of biosensing toward the detection of a specific type of biological components for diagnostic purposes. In addition to being highly specific to target molecules, materials used for bioimaging must be biocompatible and nontoxic.
The design of biosensors using various biomaterials involves an interdisciplinary approach that spans multiple scientific fields. Hence, in the last few decades, additional research has been conducted to develop efficient diagnostic systems using biomaterials. Kong et al. have studied an unconventional antenna-like heterostructure composed of arrays of nanoporous Prussian blue (PB) nanocube heads/TiO2 nanowire (NW) arms (PB-TiO2), which they developed for efficient three-dimensional interfacial sensing of small molecules and cellular activities. The optimized PB-TiO2 antenna NW biointerfaces exhibit remarkable sensing performance in detecting H2O2 with high sensitivity and selectivity, a broad detection range from 105 to 108 M, a low detection limit of 20 nM, and a response time shorter than 5 s[129].
Engineering functional tissues requires fabricating constructs that can deliver suitable biological, chemical, mechanical, and architectural features to direct cellular growth. In direct-write printing, polymeric or hydrogel fibers are extruded through a nozzle and deposited in a predefined pattern to form a desired structure[130]. In textile processes, by contrast, these fibers are fabricated and then assembled into 2D fabrics and 3D constructs with tunable properties. Textile-based structures were first used in tissue engineering to construct scaffolds with tailored mechanical properties[131]. Onoe et al. designed a microweaving machine to weave cell-laden hydrogel fibers[132]. In another example, hydrogel fibers were reinforced with sacrificial alginate templates that could withstand the mechanical stress applied during weaving and braiding, thereby enabling the fabrication of constructs with clinically relevant dimensions[133]. Recently, Akbari et al. reported the engineering of composite fibers with a mechanically strong core from synthetic polymers covered with a layer of cell-laden hydrogel[134]. The multistep fabrication of these composite fibers provides an opportunity to add functionalities to the engineered tissue. For example, polymeric fibers can be used for controlled release of biological factors and cues to direct cellular growth and differentiation[135]. To this end, drug-eluting polymer-based structures have been fabricated that can be used for many tissue engineering applications such as three-dimensional bio-printing and the repair/replacement or regeneration of bone, blood vessels, and organs such as kidneys and hearts[136–139]. Fibers and textile technologies can also be used in regenerative medicine and cell therapies as drug and cell carriers. Similarly, textile technologies can be used in regenerative medicine through engineering advances of surgical meshes with regenerative properties.
4.
Device configurations
Functional hybrid fiber-based electronic devices encompass electronic functionalities on fiber/textile materials with robust mechanical properties. Technical textiles are used primarily for their technical functionality in many different industries. Sensors can be integrated into textiles to monitor their functionality. Because textiles are made of yarns, two-dimensional structures, or three-dimensional structures, the sensor systems should be accordingly designed as a part of them. “Smart textiles” refers to textiles with mechanically and structurally integrated sensors. In this section, we discuss electronic devices based on fiber materials and their use in textile electronics and biosignal monitoring processes. In particular, we highlight recent developments in textile-based electronic devices and their applications.
4.1
Textile electronics
Over the past decades, great effort has been devoted to the development of smart textiles, which generally are fabricated by integrating electrical circuits into traditional textiles to modify their functionalities. In general, these textiles are defined as “smart” because they offer functions that enable them to directly respond to environmental stimuli such as mechanical, thermal, chemical, electrical, and magnetic stimuli. To realize the “smart” functions of a textile, conductive textile yarns or fibers are generally used to interface with other electronic devices integrated into the textile. Textile engineering, information technology, and electronics manufacturing have flourished in attempts to introduce advanced electronic textile solutions into vastly different applications. Indeed, some products that originated from the field of smart textiles are already found in the market. Nevertheless, many concepts remain at their initial stage of research because of various technological and socio-economic barriers. One of the key issues in the development of electronic-based smart textiles is the investigation of how to bring textile technologies and the desired electronics together. The integration of electronic devices into textiles at the fiber level plays a major role in the fabrication of future of wearable electronics. Many factors must be considered before incorporating electronic devices into a textile-based system; these factors include the materials, structures, and the compatibility of electronic devices with textile fabrics[140, 141].
OFETs have received significant attention because of their flexibility, compatibility with flexible electronics, lightweight, biocompatibility, low cost, and compatibility with low-temperature processing. Because of their flexibility, easy fabrication process, and comparable electrical performance (as compared with that of inorganic thin-film transistors (TFTs)), organic-based transistors are required in the textile industry[142–144]. Several types of fiber-based organic thin-film transistors (OTFTs) such as fiber organic field-effect transistors (F-OFETs) and organic electrochemical transistors (OECTs) have been reported thus far[145]. Maccioni et al. reported an OFET characterized by a textile process with fully compatible size and geometry. Their device was fabricated using cylindrical metal fibers with a diameter of 45 μm, shielded by a uniform layer of polyimide approximately 1 μm thick. These fibers enhance the flexibility of yarn and can be employed, after being twisted into a cotton fiber, in textile processes. The obtained transistor has shown very interesting performance characteristics, with typical electronic parameters (i.e., mobility and threshold) very similar to those of planar devices. Such fibers are very promising in view of innovative applications in the field of smart textiles. In particular, the realization of distributed transistors and sensors in a textile network is the natural, most promising perspective for various applications[146].
A light-emitting diode (LED) is a semiconductor device that emits visible light when electron– hole recombination occurs inside the device. Since the commercial infrared inorganic LED was first developed by Holonyak et al. in 1962[147], various LEDs have been developed around the world, including visible, ultraviolet, and infrared LEDs[148–152]. Although LEDs have limited functionality in textiles, they are a reliably simple tool to add desirable optical effects to textiles. For these applications, technical embroidery is a beneficial technology that ensures efficient and reliable manufacturing. For example, a Swiss company, Forster Rohner, has developed an embroidery-based approach to manufacture luxury interior textiles and clothing outfitted with integrated LEDs[153]. ZSK Stickmaschinen and the research institute TITV Griez have also demonstrated efficient approaches to manufacturing light-emitting textiles via embroidery technology and the application of LED sequins, respectively.
Sensors are extensively used as electronic devices that detect the characteristics of their surroundings; they are also used in a wide variety of applications such as electronics, aerospace, healthcare, robotics, and machinery[154–160]. In this review, we focus on textile-based pressure sensors because sensing pressure is one of the most important functions of wearable electronics. Shimojo et al.[161] fabricated a tactile sensor using pressure-conductive rubber via the development of a thin, flexible, attachable, tactile sensor. They measured the change in resistance under constant strain, observing the change in resistance over a period of 100 s. Their tactile sensor, when properly operated on a robot hand, demonstrated good durability under repeated bending tests. Recently, Lee et al. fabricated a textile-based pressure sensor using Ag-SBS (styrene-block-butadiene-block-styrene) composite conductive fibers[162]. A capacitive-type textile-based sensor was fabricated using a PDMS coating on a conductive fiber followed by cross-stacking of two PDMS-coated fibers, as shown in Fig. 2(a). By stacking the PDMS-coated conductive fibers perpendicularly to each other, they fabricated a capacitive-type textile-based pressure sensor at the cross point of the fibers. The photograph in Fig. 2(b) illustrates the textile-based pressure sensor fabricated on a flexible polyethylene terephthalate (PET) substrate, which shows that the textile-based pressure sensor not only has a simple device fabrication process but is also highly flexible because of the textile materials. Fig. 2(c) illustrates the relative changes in capacitance of fiber-based pressure sensors via increasing applied pressure loads. In addition, the capacitive response of the pressure sensor shows that it generates noise-free and stable continuous responses under the various loads. In this operation, the conductive-fiber-based pressure sensor showed stable responses against various loads (Fig. 2(d)). This pressure sensor detected the loading and unloading of grains of extremely low weight. This work further demonstrates e-textile applications of conductive fiber-based pressure sensors[162].

class="figure_img" id="Figure2"/>
Download
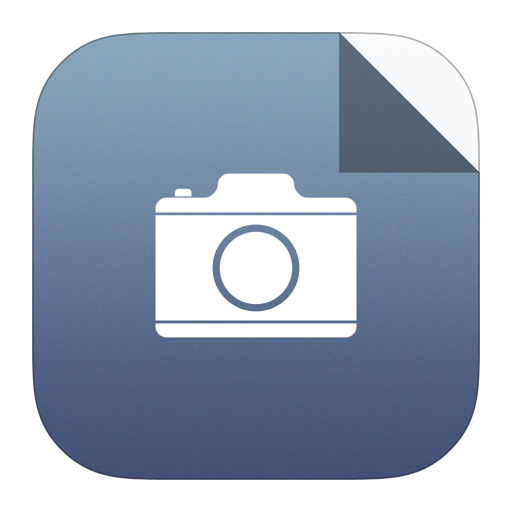
Larger image
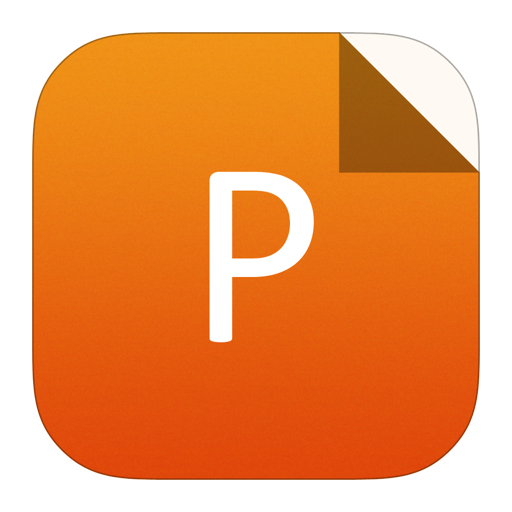
PowerPoint slide
Figure2.
(Color online) (a) Schematic of the fabrication of the pressure sensor. (b) Photograph showing the fabricated pressure sensor on a PET substrate using 2 × 2 conductive fibers. (c) Capacitive response of the pressure sensor for various applied loads of 0.05, 0.1, and 0.5 N. (d) Response and relaxation curves for the device under repeated application and removal of a 0.5 N load. Reproduced from Ref. [162] with permission by John Wiley and Sons, Copyright 2015.
A stretchable conductor is the most important requirement for fabricating integrated stretchable electronics. Matsuhisa et al. developed a high-performance stretchable and printable elastic conductor by the in situ formation of silver nanoparticles (AgNPs); the conductor was formed by simply printing an ink comprising fluorine rubber, fluorine surfactant, silver flakes, and methyl isobutyl ketone (MIBK) as the solvent (Figs. 3(a) and 3(b))[163]. As shown in Fig. 3(c), the influence of AgNPs on the conductivity is demonstrated by the conductivity–strain characteristics. The elastic conductors with a surfactant exhibited a high conductivity of 4919 S/cm without strain (0%) and 719 S/cm at a strain of 300%. Alternatively, elastic conductors without surfactant exhibited a conductivity of 3727 and 297 S/cm at strains of 0% and 300%, respectively. Furthermore, elastic conductors with surfactant prepared at a low temperature showed conductivities of 5.5 × 10?5 and 17 S/cm at strains of 0% and 300%, respectively. Similarly, the conductivity for various surfactant volume fractions at strains of 0% and 300% is shown in Fig. 3(d). The results clearly show that the conductivity is more strongly affected by the addition of surfactant than by low temperatures. The initial conductivity reached a maximum value of 4972 S/cm for 10 vol% of surfactant and reached 915 S/cm at 300% upon the addition of 5 vol% surfactant. In addition, the initial conductivity and stretchability for elastic conductors prepared at different annealing temperatures (80–150 °C, 1 h) after an initial 80 °C, 1 h drying step are illustrated in Fig. 3(e). The initial conductivity increases from 5.5 × 10?5 to 4919 S/cm when the annealing temperature is increased to 120 °C. The conductivity at 300% strain is also improved from 17 to 719 S/cm. Annealing temperatures greater than 130 °C result in conductivities greater than 104 S/cm at the expense of stretchability. Hence, the effect on stretchability of introducing AgNPs is clearly revealed. The fabrication process for the proposed elastic conductors is highly compatible with large-area printing processes. The in situ formation of AgNPs in the elastomer matrix enhances the conductivity because uniformly dispersed AgNPs improve the percolation between micrometer-sized silver flakes and because crack formation is suppressed by the reinforcement effect of the AgNPs. This development represents a new approach to further improving elastic conductors. The electrical and mechanical performance of these types of composites is expected to be further improved through investigations of elastomers, surfactants, silver flakes, and diffused silver ions[163].

class="figure_img" id="Figure3"/>
Download
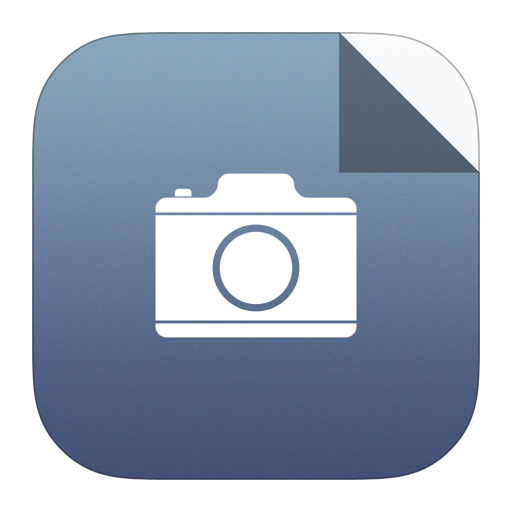
Larger image
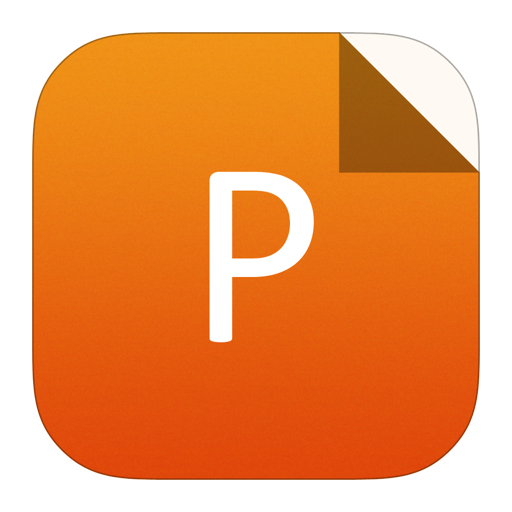
PowerPoint slide
Figure3.
(Color online) Printable elastic conductors by in situ formation of silver nanoparticles (AgNPs) from silver flakes. (a) Fabrication process. (b) AgNPs are in situ synthesized just by mixing four components and printing. Electrical characteristics of elastic conductors with different formulations. (c) Conductivity–strain characteristics of elastic conductors with and without surfactant and with and without high-temperature treatment. (d) Dependence of conductivity on the volume fraction of fluorine surfactant. (e) Dependence of conductivity and stretchability on the post-process annealing temperature. Reproduced from Ref. [163] with permission by Nature Publishing Group, Copyright 2017.
4.2
Bio-signal monitoring
Technological advances in the fabrication of smart materials and sensors have increasingly changed the landscape of healthcare by enabling the development of devices that can continuously monitor a patient’s health status[164–170]. Devices such as implantable diagnostic devices (IDDs) and smart wearable systems (SWSs) are capable of in situ sample collection and integration with the complex three-dimensional (3D) structure of biological tissues; these capabilities offer vital opportunities in the diagnosis and treatment of diseases. The development of such devices requires overcoming challenges associated with the mismatch between the mechanical and topographical properties of semiconductor-based electronics and biological tissues. Flexibility and biocompatibility are other key characteristics needed for devices in these applications. However, device microfabrication on such materials is critical because it requires clean-room facilities and specialized processing.
Paper has emerged as a promising substrate for implantable devices and wearable electronics because of its universal availability, low cost, environmental friendliness, and ease of fabrication[170–172]. Mostafalu et al. have fabricated thread-based microfluidic networks that interface cordially with biological tissues in three dimensions, as presented in Fig. 4(a). These authors have demonstrated the fabrication of threads with different physical, chemical, and biological functions to serve as sensors, microfluidics, and electronics and to be integrated as a thread-based diagnostic device (TDD)[173]. A thread-based pH sensor comprising conductive threads and a microfluidic splitter with three channels for the delivery of a sample to the sensing chambers are illustrated in Figs. 4(b) and 4(c), respectively. The microfluidic splitter was fabricated by developing patterns of hydrophilic threads on a hydrophobic woven fabric. To measure pH, a potentiometric approach was used. Similarly, a schematic of pH measurement in an in vitro skin model is shown in Fig. 4(d). The stability of the pH measurement under different acidic/basic conditions is shown in Fig. 4(e). A rapid response time shorter than 30 s was achieved as the signal, which was stabilized further within a few seconds after a change in the pH. As shown in Fig. 4(f), the voltage was linearly dependent on the pH value, with a slope of ?59.63 mV per pH, which indicates near-ideal Nernstian behavior. To evaluate the long-term signal stability, the voltage drop was measured in a solution with a pH of 7.4 for 4 h. A 2.5 mV/h drift was observed, indicating high stability of the fabricated pH sensors (Fig. 4(g)). The primary benefit of this TDD is that threads are inexpensive and biocompatible, having been widely used in the clothing industry. These devices have the potential to act as a part of human skin or clothing and to even be implanted. The ability to suture TDD intimately into a tissue or organ in three dimensions adds a unique feature that is not available with other flexible diagnostic platforms. In addition, this TDD could eventually find a wide range of applications, such as smart sutures for surgical implants, smart bandages to monitor wound healing, integration with textile or fabric as personalized health monitors and point-of-care diagnostics, and embedding into engineered tissue constructs for organ-on-a-chip platforms.

class="figure_img" id="Figure4"/>
Download
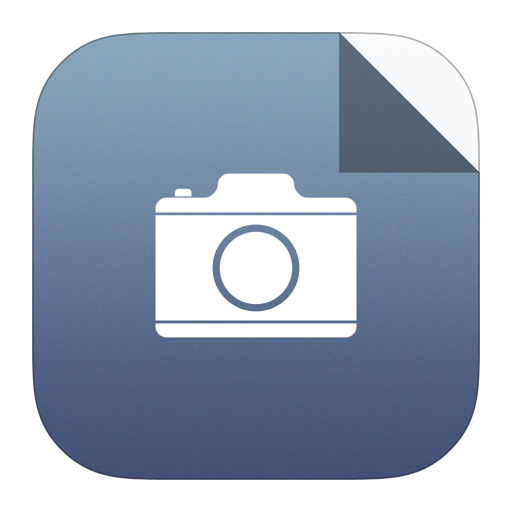
Larger image
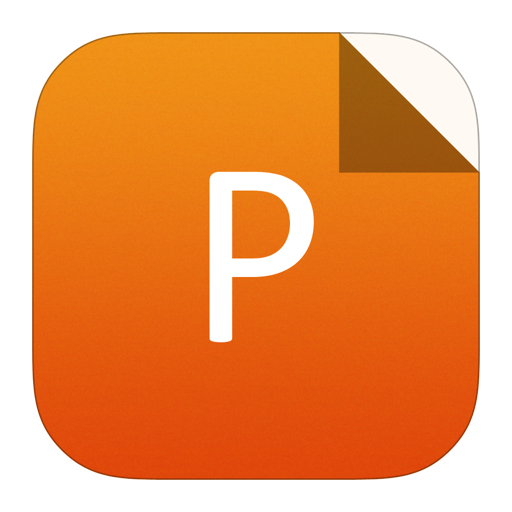
PowerPoint slide
Figure4.
(Color online) (a). A toolkit of thread-based chemical and physical sensors, microfluidic channels, and interconnects for the realization of a thread-based diagnostic device (TDD), shown here for transdermal health monitoring, characterization of chemical sensors. (b) and (c) Optical image of a multiplexed microfluidic pH sensor assay. (d) Schematic of the measurement of pH in an in vitro skin model. (e) Transient response of the pH sensor to different pH values. (f) Calibration plot of the pH sensor. (g) Continuous pH measurement for 4 h. Reproduced from Ref. [173] under the CC BY license.
5.
Importance of the electronic technology
The concept of clothing is continuing a revolution through innovation in wearable technologies. Thus, this concept brings static elimination, stain repellence, electrical conductivity, and wrinkle-freeness, to fibers without compromising their comfort and flexibility properties[174]. Intelligent clothing has an increasing demand in prominent fashion weeks in New York, London, and Paris. Now a day, fashion designers are creating functional materials and integrating emerging communication devices, flexible electronics, and nanomaterials to garments and designer clothes[174]. Cotton is a generally used as fiber, which exhibits high absorbency, softness, and breathability. However, the application of the use of cotton is limited since its fibers have relatively low strength, low durability, easy creasing and soiling, and flammability[175]. Synthetic fibers can be antimicrobial and stain/crease-resistant but generally lack comfort as compared to cotton[176]. Flexible electronics and optical devices can be integrated into textiles[175]. The applications of the functionalized textiles include medical monitoring of body function and metabolism[177], and electronic devices integrated into clothes[178]. Furthermore, these technologies allow integrating sensors into textiles[179]. Textile is an universal interface and ideal substrate for the integration of nanomaterials, electronics, and optical devices. Such integrated materials and technologies aid a platform that responds to mechanical, chemical, electrical, thermal, optical, or magnetic stimuli. These engineered materials should seamlessly integrate into garments and be flexible and comfortable while having no allergic reaction to the body[174]. A significant challenge in the textile industry is that conventional approaches to functionalize fabrics do not lead to permanent effects. In recent years, flexible electronic skin sensors and wearable electronics have demonstrated excellent potential for applications in medicine[180, 181], energy[182, 183], robotics[184, 185] and optical detection[186]. As the field quickly advances, a wide range of materials have been explored as sensing elements to fabricate e-skin devices, including organic/inorganic matrix arr- ays[187, 188], polymer composites[189], hybrid composites[190], and nanomaterial assemblies[191]. To date, the sensing capabilities of these devices have been limited by the compromise between selecting materials that are mechanically durable yet also highly sensitive to strain[192, 193]. Monitoring of physiological signals is an effective approach to the assessment of human health problems. This monitoring is currently limited to hospitals, as currently available devices, including infrared-based optical electronics and rigid multi-electrode pressure sensors, are not portable or wearable. Recently, flexible and stretchable artificial e-skin has intensively demonstrated due to its unique capability to detect subtle pressure changes, which may allow applications in wearable individual-centered health monitoring, sensitive tactile information acquisition, minimally invasive surgery, and prosthetics[194, 195].
6.
Application of hybrid functional microfibers
6.1
Application for e-textile
6.1.1
Power devices
All electronics, including fiber ones, need power to function. Fiber-shaped power devices can power wearable, mobile, and stationary electronic devices to communicate, lighten, cool, heat, and so on, by converting sunlight, mechanical motion, or chemical energy into the required energy forms. The current study mainly focuses on fiber solar cells and fiber-shaped nanogenerators.
6.1.2
Fiber-shaped solar cells
No a day, more studies on fiber-shaped photovoltaic (FPV) or fibershaped solar cells are primarily focused on DSSCs and organic thin film solar cells[196]. Reports on flexible fibershaped dye-sensitized solar cells (FDSSCs) have been few prior to the work of Zou and co-workers[197]. They fabricated FDSSCs by coating TiO2 nanoparticle thin films on a stainless wire and then directly sintering the electrodes at a high temperature. FPV technology fits all kinds of solar cell fiber substrates. Wang and coworkers[198] adopted ZnO nano wires grown on an optical fiber to prepare a new type of fiber DSSC based on the optical fiber substrate. They coated a layer of ITO on the optical fiber. The preparation of efficient fiber-shaped organic photovoltaic cells (FOPV) face many difficulties compared with that of FDSSCs. Some groups have tried to fabricate FOPVs, but the devices they formed have low performance. Fiber-shaped photovoltaic devices, including polymer solar cells (PSC) and dye-sensitized solar cells (DSSC), are light-weight, wearable, and integratable with other micro-devices[199, 200].
6.1.3
Fiber-shaped nanogenerator and composite fiber-shaped devices
Aside from solar energy, thermal, chemical, and mechanical energies such as vibration or disturbance originating from footsteps, heartbeats, ambient noise, and airflow from the environment are also studied. Wang and his co-worker[200, 201] proposed the use of textile fibers to convert these low-frequency vibration/friction energies into electricity using piezoelectric zinc oxide NWs wrapped around two entangling piezoelectric fibers. When one of the fibers is stretched, the NWs rooted on the fibers are brushed, and mechanical energy is converted into electricity because of a coupled piezoelectric–semiconductor process. The textile–fiber-based nanogenerator they fabricated reveals low power output but has an expected output density of 20–80 mW per square meter of the fabric with fiber-bundles. Mechanical energy can be harvested using a fiber-shaped nanogenerator to produce electrical power. Wang and co-workers demonstrated this concept by coating CFs with a thin layer of ZnO film[202].
6.1.4
Fabric antenna
Tao et al. reported that the most common of the research works on fabric antennas including rectangular micro strip patch antennas due to their advantages of miniaturization, ease of integration, and good radiation directions and low sheet resistivity. Highly conductive fabrics with outstanding flexibility and stretchability, as well as superior stability of the resistivity and homogeneous resistivity distribution under extreme mechanical deformation during their assemblage and wearing, are well-intentioned of further studies, not only for conductive layers of the fabric antenna, but also in great need by super capacitors in wearable energy storage. The materials with small moisture absorption and suitable for manufacturing of fibers and yarns are preferable for use as substrates and also as conductive components of the antenna, and can be further studied to guarantee of a stable fabric antenna[203].
6.1.5
Smart garments for healthcare applications
Smart garments for exhibiting physiological and biomechanical signals of the human body are decisive sensors for personalized health care. Wearable devices for healthcare applications like smart shirt can monitor vital signs such as heart rate, body temperature, etc. Highly sensitive, stretchable and wearable multifunctional sensors have been made to detect multiple stimuli such as stretch, strain, pressure, temperature and finger touch with high sensitivity, fast response time and good pressure mapping function. Flexible sensing devices have been reported for several wearable applications including monitoring thumb movement, sensing the strain of the knee joint and other human movements such as walking, running and jumping from squatting[204, 205].
6.2
Application for biosenosrs
Generally, a biosensor is an integrated device, which is designed to detect or quantify bacteria, viruses or a variety of biochemical molecules including specific DNA sequences, proteins, enzymes, antibodies and oligonucleotides. Electrospinning is one of the most easy, versatile and cost effective technique that can be used to produce sub-micrometre scale ultrafine fibres[206–208]. The use of this fabrication technique in areas of research such as filtration, biotechnology, protective clothing, drug delivery and tissue engineering has been widespread due to the ability to create fibres with high surface area[209–212]. In tissue engineering, the design of the electrospun ultrafine fibres has been used to mimic the fibrillar nature of the extracellular matrix (ECM), allowing for its use as a substrate for cell growth[212]. Electrospun ultrafine fibres show some promise for drug delivery applications due to the possibility of high drug loading capacity and potentially a lower initial burst release, particularly for core-shell coaxial geometries[213, 214]. Most of their intrinsic properties such as high surface area and porosity are advantageous for the loading and subsequent release of drug molecules. A number of studies using individual or a combination of surface modification methods have been carried out on electrospun fibers for drug delivery applications. For example, control over drug release characteristics was achieved via introduction of hydrophobic functional groups onto the surface of electrospun fibres through fluorination.
Functional hybrid microfibers have a wide range of applications due to their ability to generate a large evanescent field which is readily accessible, the ability to tightly confine modes to the microfiber surface, and the capability to form useful configurations with small losses to allow for the development of physically compact devices. Structural modification on optical microfiber is of great promise due to their compactness, large evanescent field effect, low stiffness, and good flexibility. A lot of studies related to the fabrication and the potential applications of structural modification on optical microfiber in sensing and communication have been conducted in the past. Nowadays, a variety of microfiber Bragg gratings have been successfully fabricated by introducing periodic variations of either the refractive index or fiber geometries, with a wide range of techniques. By fabricating fiber Bragg gratings (FBG) within optical microfibers, the advantages of microfibers and FBGs can be combined to develop sensors with improved compactness and faster responses. Similar to conventional FBGs, microfiber FBGs can be fabricated by periodically modifying the refractive index along the microfibers. FBGs can also be developed by direct precision milling on the surface of microfibers using a focused ion beams technique[215, 216]. Several microfiber FBG structures have been developed for use as refractive index sensors[217, 218] with diameters ranging from 2 to 6 μm. In addition to refractive index sensors, a microfiber FBG temperature sensor[217], and a force sensor[218] have also been developed. The advantage of using a microfibre tip as a biosensor head is the possibility of a spatial operation, especially in confined areas.
7.
Conclusion and future prospects
In this review article, a brief description of functional hybrid microfibers for textile and biosensors applications was presented, with emphasis on electronic components, where the various types of fibers and fabrication techniques using different materials such as polymers, CNTs, graphene, and metal polymeric materials were summarized. In particular, the integration of graphene nanosheets into macroscopic fibers provides a new material platform for developing unconventional smart systems and devices. Fiber-based electronic components (transistors, LEDs, and sensors) have also been described. Electronics based on fibers that are thousands of times smaller than textile microfibers, i.e., nanofibers/nanowires, is critical for the future development of organic electronics.
Although significant performance in textile-based electronic devices has already been achieved, additional efforts are required to further improve the performance of the electronic components. Fiber-based devices have distinct advantages in various applications such as wearable optoelectronics. In some cases, compromises must be made to balance different factors. In addition to the examples discussed here, we envision that the application scope of fiber-based devices will rapidly expand. Textiles are ubiquitous in human life and should be both lightweight and comfortable. Although fiber-shaped electronic devices have undergone substantial advancements with respect to the methods of fabricating fiber-shaped electronics and weaving fiber-shaped electronics into textiles, most of the reported fiber-shaped electronic prototypes are far from fulfilling the requirements for final application given that several key issues remain to be solved. The first challenge is the development to solid-state fiber-shaped electronic devices with high flexibility and performance. The second challenge is the further development of small-sized electronics on fibers, especially fiber-based transistors. Transistors are the critical component of circuits and play critical roles in various ultimate electronics applications, including sensors, charge-control systems, computing, and others. The third challenge is the integration of fiber-shaped electronic devices into textiles with a better and more comfortable human/textile interface design. The difficulty associated with weaving fiber-shaped devices is a certain problem that confronts scientists in this field. Some special, promising measures have been thoroughly studied by various researchers. However, most of these measurements are either still in the infancy stage or are confined in some particular material systems. Moreover, the human/textile interface is not taken into consideration. To address these problems, all polymer-based fibers should be used to prepare fiber-shaped electronics. Commercial fibers, both synthetic (carbon fiber, nylon, Kevlar fiber, etc.) and natural (silk, cotton, etc.), with excellent human/fiber interfaces, are readily encountered in our daily life. However, the major materials face problems because of their conductivity. With the exception of carbon fibers, many of them are poor conductors[219].
Despite the above-mentioned impressive achievements, there still remains a challenge to further develop high-performance E-fibers, which can concurrently confirm high energy and power capacities toward practical applications. According to the related publications, it can be noticed that current reports are still at the early stage and focused on basic electrochemical performance, while much less attention is paid to the mechanical properties. In particular, the lack of a unified evaluation system is a problem as reported values in terms of specific capacitance; power and energy density are quite confusing. It is better to provide the performance for the complete device when considering the practical application requirements. Furthermore, based on our foregoing discussions, we think that the following issues should be paid much attention in future researches on E-fibers. Thus, the practical applications require that E-fibers should be prepared using low-cost raw materials and relatively simple methods. Practically, excellent properties are sometimes built on expensive raw materials and complicated processes, therefore, the comprehensive performance (cost and electrochemical performances, etc.) of E-fibers is worthy of concern.
The future development of sensors in the field of flexible and wearable electronics, robotics etc. depends highly on the possibilities of various conducting polymer composites and a lot has to be derived in order to commercialize such products. It is certainly challenging to construct a sensor with superior sensitivity, better selectivity, long-term stability and better protection or package for practical applications. Furthermore, the sensors must be cost effective, portable, and conducive to commercialization with minimal false refusals. Achieving a reproducible manufacturing process for the sensors is one of the most important facts to be considered now days. Optimizing the dispersion and orientation of graphitic fillers improves structural and sensing performance. As far as fabrication process is concerned, electrospinning is a convenient and versatile technique to fabricate micro/nanofibers and nanofibrous scaffolds for tissue engineering applications. The main challenges encountered during fabrication is the inability to control fiber size and arrangement for optimized cell infiltration. Although graphene based fiber sensors have been applied to many applications, much effort remains to be done before commercial optical fiber sensors. The existing fabrication methods are still not suitable enough, for example, the size and thickness of the graphene are difficult to control and there will be some defects or pollutions in graphene. The repeatability and stability of the graphene sensors are not optimistic. Hence, much effort is also needed to be done, the future for graphene sensing devices looks very exciting.
Despite the impressive progress, there are still some challenges and opportunities in fundamental research and practical applications. Firstly, the evaluation methods of device performance necessity to be standardized. In the literature, the performance metrics are expressed in various forms based on different units. For example, the gravimetric/volumetric/aerial/ linear capacitance/energy density/power density, the mass/volume/area calculated from the active materials, electrodes or whole device should be clarified. Otherwise, it would be very confusing to compare the reported results and guide further researches. A possible solution is to set up standardized guidelines for reporting the performance of FSCs. The techniques for low cost and large-scale production of FSCs should be developed in view of the long-term goal for real applications. Although there are some pioneering works for continuous fabrication of FSCs, the huge market of wearable electronics requires more efficient production of high-performance devices. This is also the demand for future developing of power textiles based on fiber-level electronic building blocks, due to the necessity of large quantity and long length of FSCs during textile assembly process. The compatible weaving technology is yet to be developed to maintain the physical and electronic properties of the resultant textiles. Challenges also face nanomaterials in various important applications such as cancer diagnostics, detection of pathogenic organisms, food safety, environmental measurements, and clinical applications. Aptamer-based microarrays for the quantitation of multiple protein analytes as well as metal-enhanced electrochemical detection concepts have been demonstrated for the sensitive detection of Ab–Ag, DNA–drug, DNA–DNA, and DNA–toxin interactions. With simultaneous advances in textile technologies, applications of multifunctional hybrid fibers for electronic textiles and biosensors may soon be within reach.