1.
Introduction: Si photonics overview
Si photonics, also widely known as group IV photonics, refers to integrated optical circuits on Si platform to achieve electronic-photonic integration. The key driving force behind Si photonics is synergistically integrating the advantages of photons in energy-efficient, high bandwidth data transmission with those of electrons in high-speed data processing, thereby overcoming the interconnect bottleneck and achieving high functionality extension of Moore’s Law. The investigation on Si photonic integration dated back to 1980s, when the first study of single crystal intrinsic Si ridge waveguides were reported on doped Si substrates via homoepitaxy[1], followed by Si on sapphire (SOS)[2] and Si on insulator (SOI) substrates. Due to the large refractive index contrast between Si (core) and SiO2 (cladding), single-mode Si waveguide with tight bending radius and high optical confinement offers the possibility of integrating a greater number of microphotonic devices on the SOI platform. These Si waveguides are often referred to as “photonic wires”. SOI wafers with 220 nm-thick top Si layer is increasingly adopted as the standard platform for Si photonics[3]. Inverse nanotaper couplers and grating coupler are often used to implement low-loss coupling between optical fibers and Si waveguides[3].
The early work from the late 1980s to the mid-1990s was mainly focused on the fundamental studies of the building blocks of Si photonics[1, 4, 5], while the past two decades witnessed an exponential growth in the worldwide integrated photonic device research and commercialization efforts in this field[6, 7]. Fig. 1 shows the forecast of the silicon photonics market for the coming decade (2015–2025) from Yole Développement Group[8], a "More than Moore" market research & strategy consulting company. The market is projected to grow from less than US$40M in 2015 to US$1.5B in 2025 with a compound annual growth rate (CAGR) of 43.6%. Si photonics products are mainly applied in power-efficient, high-speed data communication (datacom) and high-performance computing (HPC). Emerging optical data centers from big Internet companies, such as Google, Microsoft, and Facebook, will be triggering the market growth in 2018. The metrics for large-scale deployment of Si photonics in these markets are low cost per data lane (~$1/Gbps), low power consumption per data lane (a few pJ/bit), high reliability and high fabrication yield.

class="figure_img" id="Figure1"/>
Download
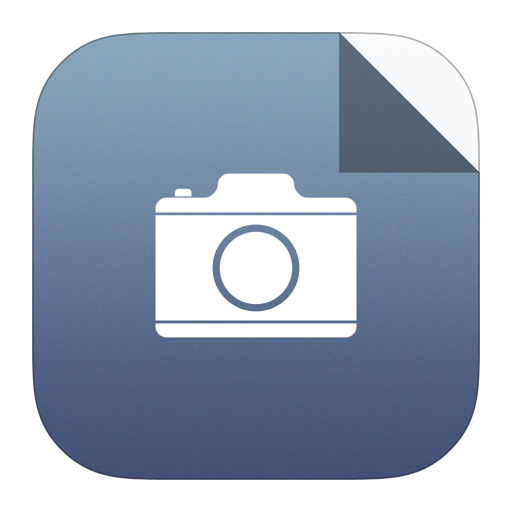
Larger image
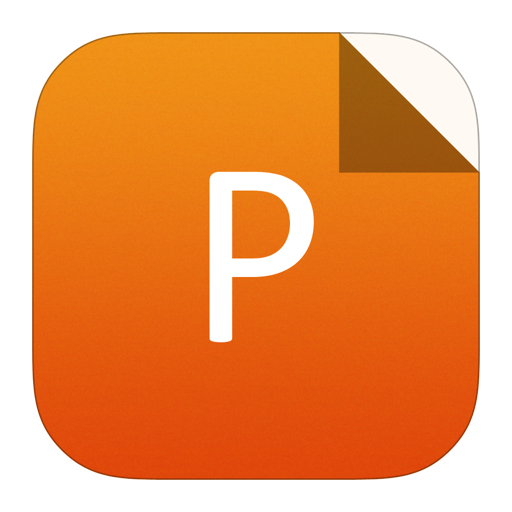
PowerPoint slide
Figure1.
(Color online) Silicon photonics 2015–2025 market forecast. Reprinted from Ref. [8]. Silicon Photonics Report with permission from Yole Développement. ? Yole Développement 2016.
Over the years, it has been highly debated whether Si monolithic integration or hybrid integration with III–V semiconductors is the optimal route for the applications in datacom and HPC. This debate largely arises from the difficulty in implementing monolithic active photonics on Si, i.e. devices for photon generation, amplification, modulation, manipulation, and detection. It is undoubtedly true that ultimately monolithic integration takes advantage of the mature Si integrate circuit infrastructures (foundries) for high-volume, high-yield production and low fabrication cost[9]. However, even with years of attempts and efforts, some active photonic components for monolithic integration are still not superior or comparable to their III–V counterparts. Particularly, III–V lasers are still commonly used either by chip bonding or external coupling to Si waveguides through precise assembly. On the other hand, many other Si photonic components are highly monolithically integrated for 100 Gbps Si photonics transceivers shipped by the key players (e.g. Mellanox-Kotura, Luxtera and Intel)[10–12]. In the recent decade, it is also interesting to note that the attention is turned gradually from the heated debate over monolithic versus hybrid integration to the exploration of the possibilities of new materials and innovative approaches to meet the challenging metrics of high performance and low power consumption at a low cost.
This paper reviews recent efforts and progress in implementing active photonic devices on Si since they will largely determine the integration schemes and performance of Si photonics. In addition, the paper will distinguish itself from other reviews in this field by focusing on emerging technologies beyond conventional Si photonics devices that have already entered industrial foundries. The paper is organized as following: in Section 2 we will summarize the development, challenges, and emerging directions of active photonic components for silicon photonics. Section 3 discusses the latest advances in group IV active photonics in detail. Section 3.1 reviews monolithic and hybrid lasers on Si, including band-engineered Ge, direct gap GeSn, and hybrid InAs quantum dot (QD) lasers on Si. Novel modulator materials and devices beyond the free carrier plasma dispersion effect in Si will be presented in Section 3.2, including GeSi and graphene electro-absorption modulators (EAMs) and plasmonic-organic electro-optical modulators (EOMs). Emerging photodetectors (PDs) beyond epitaxial Ge p–i–n photodiodes, including GeSn mid infrared (MIR) PDs, all-Si plasmonic Schottky infrared (IR) detectors, and photon counting Si quanta image sensors (QIS), will be presented in Section 3.3. We will also discuss electronic-photonic integration strategy and schemes throughout Section 3. Finally we will conclude with a brief outlook on these emerging technologies in Section 4.
2.
Development and challenges of active devices in Si photonics
Over the past two decades, an almost complete set of passive and active photonic components have been developed on the SOI platform, including waveguides, couplers, splitters, filters, polarizers, (de)multiplexers, modulators, switches and photodetectors[6, 7]. Many of these devices are being standardized in Silicon Photonics Process Design Kits (PDK) under the American Institute for Manufacturing Photonics (AIM Photonics)[13], as shown in Table 1. This is an important step towards photonic standardization for large-scale integration.
PDK passive components | Qty | Selected performance |
Waveguides (Si & SiN), curves, etc. | 16+ | Si : < 2.2 dB/cm, SiN : < 1 dB/cm |
Edge couplers (Si & SiN) | 2 | < 2.5 dB/facet loss |
Vertical couplers (Si & SiN) | 2 | < 3 dB loss |
3 dB 4-port couplers (Si & SiN) | 2 | loss < 0.5 dB, deviation < 1% |
Y-junctions (Si & SiN) | 2 | loss < 0.25 dB, deviation < 1% |
Directional coupler (Si & SiN) | 2 | loss < 0.5 dB, deviation < 1% @ 1550 nm |
Si-to-SiN coupler (escalator) | 1 | loss < 0.1 dB |
Crossing (Si) | 1 | loss < 0.25 dB, crosstalk < ?60 dB |
PDK active devices | Qty | Selected performance |
Digital Ge photodetector | 1 | > 30 GHz, < 20 nA dark |
Analog Ge photodetector | 1 | > 25 GHz, < 80 nA dark |
Digital Mach-Zehnder modulator | 1 | > 15 GHz, > 25 Gb/s, push-pull < 2 Vpp per arm, > 5 dB extinction, < 5 dB loss |
Analog Mach-Zehnder modulator | 1 | > 15 GHz, ?10 V < Vs < 0 V, 25 dB lin., 1500–1600 nm |
Thermo-optic phase shifter (Si) | 1 | 0.25 dB loss, < 50 mW, range 0π < Δθ < 2π |
Thermo-optic switch (Si) | 1 | < 1 dB loss, 25 mW |
Tunable filter (Si) | 4 | < 0.5 dB loss, 26 nm FSR, > 1 nm/mW tuning efficiency |
Microdisk switch (tunable) | 4 | < 2 ns switch time, > 200 GHz EO tuning @ 1.2 V |
Microdisk modulator (tunable) | 4 | 15 GHz, 25 Gb/s, 1.2 Vpp, 1 dB loss, 8 dB extinction |
Table1.
Silicon photonics process design kits (PDK) components and typical performance under AIM Photonics. Table retrieved from http://www.aimphotonics.com/pdk/[13].
Table options
-->
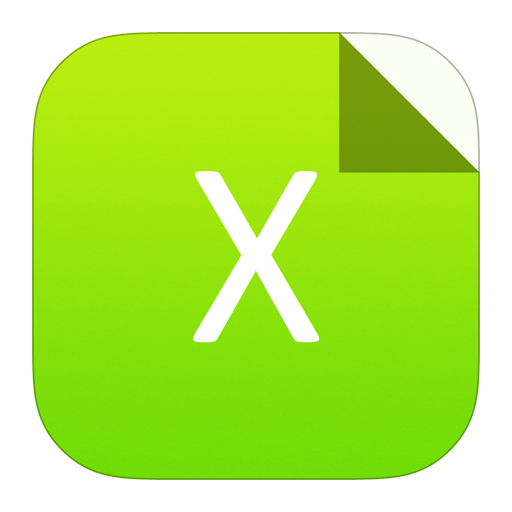
Download as CSV
PDK passive components | Qty | Selected performance |
Waveguides (Si & SiN), curves, etc. | 16+ | Si : < 2.2 dB/cm, SiN : < 1 dB/cm |
Edge couplers (Si & SiN) | 2 | < 2.5 dB/facet loss |
Vertical couplers (Si & SiN) | 2 | < 3 dB loss |
3 dB 4-port couplers (Si & SiN) | 2 | loss < 0.5 dB, deviation < 1% |
Y-junctions (Si & SiN) | 2 | loss < 0.25 dB, deviation < 1% |
Directional coupler (Si & SiN) | 2 | loss < 0.5 dB, deviation < 1% @ 1550 nm |
Si-to-SiN coupler (escalator) | 1 | loss < 0.1 dB |
Crossing (Si) | 1 | loss < 0.25 dB, crosstalk < ?60 dB |
PDK active devices | Qty | Selected performance |
Digital Ge photodetector | 1 | > 30 GHz, < 20 nA dark |
Analog Ge photodetector | 1 | > 25 GHz, < 80 nA dark |
Digital Mach-Zehnder modulator | 1 | > 15 GHz, > 25 Gb/s, push-pull < 2 Vpp per arm, > 5 dB extinction, < 5 dB loss |
Analog Mach-Zehnder modulator | 1 | > 15 GHz, ?10 V < Vs < 0 V, 25 dB lin., 1500–1600 nm |
Thermo-optic phase shifter (Si) | 1 | 0.25 dB loss, < 50 mW, range 0π < Δθ < 2π |
Thermo-optic switch (Si) | 1 | < 1 dB loss, 25 mW |
Tunable filter (Si) | 4 | < 0.5 dB loss, 26 nm FSR, > 1 nm/mW tuning efficiency |
Microdisk switch (tunable) | 4 | < 2 ns switch time, > 200 GHz EO tuning @ 1.2 V |
Microdisk modulator (tunable) | 4 | 15 GHz, 25 Gb/s, 1.2 Vpp, 1 dB loss, 8 dB extinction |
The most challenging building block in Si photonics is the high efficiency on-chip lasers since IV group materials are not good light emitters due to the nature of indirect bandgap. Worldwide efforts are made to search different approaches of monolithic Si lasers, such as low dimensional Si (porous Si, Si nanocrystals, ultrathin Si quantum wells), rare earth ions-doped Si, defects in Si, Si Raman laser, Si/SiGe terahertz quantum cascade laser (QCL), as well as Ge and GeSn lasers on Si. Earlier reviews of lasers for Si integrated photonics based on interband transitions can be found in Refs. [9, 14, 15]. Significant progress has been made in the theoretical modeling and electroluminescence of intersubband SiGe quantum cascade structures, although optical gain and lasing remain a big challenge. Readers are referred to Ref. [16] for a review of progress toward SiGe QCLs. A detailed review of band-engineered Ge-on-Si lasers can be found in a corresponding book chapter in Ref. [17]. The ongoing research in this direction is focused on the more promising direct bandgap emission from band-engineered Ge and GeSn. The first electrically pumped Ge lasers at room temperature was reported in 2012[18], followed by the most recent advance showing ~10–50 × threshold current density reduction via tunneling injection into the direct conduction valley[19]. The first optically pumped GeSn laser was demonstrated in 2015[20], but still limited to low operation temperatures up to 180 K so far[21]. Section 3.1 reviews the principles and methods of Ge and GeSn lasers to meet the considerable challenges ahead for light sources with high efficiency, high-temperature operation and high reliability. A brief update on the latest progress of hybrid InAs quantum dot (QD) lasers emitting at 1310 nm wavelength for data centers will be presented at the end of Section 3.1. The readers are also referred to an earlier review of III–V hybrid light source in Ref. [22].
Another key active photonics component is the optical modulator. The discovery of free carrier plasma dispersion (FCPD) effect makes Si promising in switching and modulating optical signals even though it lacks the strong linear electro-optical (EO) effect. Earlier studies on refractive index change Δn (up to ~10?3) as a function of electron/hole concentration ΔNe, h (up to ~1 × 1018 cm?3) laid solid foundation for the design of Si EOMs[1]. A review of Si modulators can be found in Ref. [23]. Mach-Zehnder interferometers (MZI) are generally used in these EOMs to convert phase modulation into amplitude modulation. They have advantages of high modulation depth and high speed (Table 1), but often suffer from either a large footprint or a high driving voltage greater than the standard of CMOS electronics (~1 V) even with the best modulation efficiency of VπL = 2 V·mm reported so far (without photonic cavity)[24]. The micro-resonator EOMs are much more energy-efficient due to the greatly reduced footprint and capacitance, but they require precise fabrication/trimming/thermal tuning, have very limited operation spectral range (~1 nm) and tend to suffer from resonant peak shifts due to thermo-optical effects. Therefore, new materials and technologies are needed to meet the challenges of high energy efficiency, high speed and reasonable broad operation spectral range simultaneously.
A promising ultralow power modulation mechanism is electro-absorption effect (EAE), which employs the absorption edge tilt/shift of a semiconductor material under an applied electric field. This includes Franz-Keldysh effect (FKE) in bulk semiconductor and Quantum Confined Stark Effect (QCSE) in quantum well (QW) structures. The FKE and QCSE from the direct gap transition of GeSi material is a promising candidate with typical changes of absorption coefficients (Δα) in the order of 100–1000 cm?1 under an applied electric field of 50–100 kV/cm. The strong EAE enables a very short device length of 10–100 μm compared to mm length scale of MZIs, leading to high speed and ultralow power consumption. With similar power consumption of ~10 fJ/bit, the operation spectral range (~30 nm) of GeSi EAMs is much broader and less susceptible to temperature fluctuations than Si microring modulators. Recent advances speed up the commercialization of GeSi EAMs, driven by the power efficiency requirement in datacom (<100 fJ/bit photonic data links). In addition, modulators based on novel materials such as graphene and organic nonlinear chromophores have achieved rapid progress in recent years. These new materials could break through the limits of Si modulators in the future. Section 3.2 will therefore mainly focus on new results on GeSi EAMs, graphene modulators, and plasmonic-organic EOMs.
In terms of photon detection, Si is transparent in the telecommunication wavelength window so it is not directly suitable for detectors in integrated photonic circuits. Earlier work showed that p–n junction with a heavily doped region in Si was sensitive up to λ = 1310 nm, which was used to monitor optical power in Si photonics[6]. Recently, engineered defects in Si enables photon detection at λ ~ 2000 nm[25]. However, in both cases the absorption efficiency tends to be low, and a high driving voltage is often needed to achieve a reasonable responsivity. Epitaxial Ge-on-Si with tensile-strain introduced by the thermal expansion mismatch between Ge and Si enables high optical responsivity covering the entire telecommunication wavelength range (1310–1620 nm)[26]. The development of waveguide-integrated Ge PDs and free-space Ge/Si avalanche photodiodes (APDs) has achieved great success in the past decade[27, 28], with performances comparable to III–V PDs. The epitaxial growth of Ge film and fabrication process of Ge PDs have been interweaved into the modified CMOS process flow in some foundries. For example, digital Ge-on-Si PDs with > 30 GHz 3 dB bandwidth and < 20 nA dark current has been incorporated into the Silicon Photonics PDK under AIM Photonics using Albany NanoTech Complex ( Table 1). It is also possible to extend the detection to the MIR range by alloying Ge with Sn to address new applications including sensing, spectroscopy and pyrometry. In addition, Schottky detectors enhanced by surface plasma polariton (SPP) are emerging as another facile approach to achieve photon detection at optical communication wavelengths in Si photonics. Very recently, Si-based PDs have also remarkably achieved non-avalanche, room-temperature single photon detection (SPD) using quanta image sensor (QIS) structures that greatly benefit from CMOS scaling. These devices offer interesting inspiration for the future of electron-photonic synergy in Si photonics. The most recent development beyond Ge-on-Si PDs will be discussed in detail in Section 3.3.
3.
Emerging technologies in Si-based active photonic devices
3.1
Light sources for Si photonics
3.1.1
Band-engineered Ge-on-Si lasers
(A) Modeling of Ge gain media
While Si is far from a direct gap semiconductor with > 2 eV difference between the direct and indirect gaps, Ge is much closer to a direct gap semiconductor with an energy difference of only 136 meV between direct gap at the Γ valley and indirect gap at the L valleys. This inspires band engineering to tailor Ge for efficient direct bandgap light emission. The first design paper on tensile-strained, n-type Ge as a gain medium paved the way for monolithic Ge laser on Si [29]. Tensile strain is employed to reduce the energy of the direct Γ valley faster than that of the indirect L valleys, thereby reducing the difference between the direct and indirect bandgaps. Moreover, extrinsic electrons from n-type doping fill the L valleys to the level of the Γ valley to compensate the remaining energy difference. The optical gain was evaluated from the population inversion of the direct gap transition, while the free carrier absorption (FCA) loss was approximated using the classical Drude model. The first modeling shows that, with a combination of 0.25% tensile strain and an extrinsic electron density of n = 7.6 × 1019 cm?3, a net material gain of ~400 cm?1 can be obtained from the direct gap transition of Ge despite of the FCA losses[29]. Large optical gain from Ge with > 0.3% tensile strain has also been theoretically studied. Chang et al. concluded that a large optical gain of ~7500 cm?1 can be obtained from 0.51% tensile-strained n-type Ge QWs with an n-type doping of 2 × 1019 cm?3 at λ = 1550 nm for transverse magnetic (TM) modes at an injected areal carrier density of 1013 cm?2[30]. The threshold is comparable to III–V QW lasers. With an elaborate 30 band k·p modeling, El Kurdi et al. obtained a net gain of 3000 cm?1 at λ = 3060 nm with 3% tensile strain at an injected carrier density of Δn = 1 × 1018 cm?3, exceeding the gain coefficient of GaAs at the same injection level[31]. All these theoretically studies suggest that band-engineered Ge is a promising gain medium for monolithic lasers on Si.
Five years after the first design paper, the first electrically-pumped, engineered Ge-on-Si laser was demonstrated[18] with a net optical gain remarkably larger than the original theoretical prediction. A net gain >500 cm ?1 was observed from the direct gap transition of 0.2% tensile strained Ge with n~ 4 × 1019 cm?3 doping. The n-type doping level for such a large gain coefficient is only half of the design value. Later it was found that the Drude model used in the first design paper actually significantly overestimated the free electron absorption (FEA) in tensile strained n+ Ge-on-Si, as shown in Fig. 2[32]. The absorption spectrum of n+-Ge (Fig. 2(a)) is similar to that of n+-GaAs[33] (Fig. 2(b)), consisting of interband absorption, intervalley scattering absorption (IVSA), and intravelly FEA. A first-principle model taking into account the band structure of Ge as well as acoustic phonon, optical phonon and impurity scattering is much more consistent with the experimental results, as shown in Fig. 2(a)[32]. The intravalley FEA loss is determined to be < 20 cm ?1 for n ~ 4 × 1019 cm?3 at λ ~ 1500–1700 nm, an order lower than the results from the Drude model (Fig. 2(c))[32]. Another interesting feature in Fig. 2(a) is the strong L → Γ intervalley scattering in tensile strained, n+ Ge, which favors electronic occupation of the direct Γ valley. This factor, combined with lower FEA loss, is used to explain why the first electrically pumped Ge-on-Si laser achieved a higher net gain than the theoretical prediction. Later we improved the model to calculate the net gain of n+-Ge with 0.25% tensile strain by considering the doping-induced band gap narrowing (BGN) and the corrected FEA loss calculated from the first principle[34]. As shown in Fig. 2(d), net gain at 1700 nm can be achieved at an injection level of Δn = 1.0 × 1019 cm?3. At higher injection levels, the net gain can be extended to shorter wavelengths. For example, at Δn = 5.0 × 1019 cm?3 net optical gain can be achieved in a broad wavelength range of 1550–1700 nm, and the net gain at 1650 nm can exceed 1000 cm?1. Such a broad gain spectrum of engineered Ge is suitable for on-chip wavelength division multiplexing (WDM) applications.
(B) Optical gain and lasing under optical pumping
Inspired by the promising theoretical modeling results in Ref. [29], optical gain from tensile strained n+ Ge microdisks was first demonstrated by Liu et al. using 1480 nm CW pumping in 2009[35]. Selective growth was used to provide lateral carrier confinement and improved material quality[36]. Note that the 1480 nm pump laser was selected to prevent any absorption from the Si substrate itself in the transmissive pump-probe measurement. A net optical gain of 50 cm?1 was first observed at 1605 nm from 25 μm diameter Ge microdisks with a tensile strain of 0.25% and n-type doping level of 1 × 1019 –2 × 1019 cm?3.
Optical gain has also been observed in tensile-strained n+ Ge photonic wires grown on GaAs by de Kersauson et al.[37]. An optical gain of 80 cm?1 at ~1685 nm was measured by variable strip length (VSL) method. The n-type doping level was 3 × 1019 cm?3 with an average tensile strain of ~0.4% in the Ge region transferred by a Si3N4 stressor layer patterned on top of it. The use of GaAs substrate significantly improves the material quality of epitaxial Ge layers compared to Si substrate because there is almost no lattice mismatch between GaAs and Ge. Therefore, n-type doping, tensile strain, as well as Ge material quality, are key factors to achieve population inversion and net gain from the direct bandgap of Ge.
Ultrafast spectroscopy provides more information about the influence of the L?Γ intervalley scattering on the optical gain. Lange et al. performed ultrafast pump-probe spectroscopy on intrinsic Ge/SiGe QW structures at low temperatures and reported transient gain on the order of several hundred cm?1[38]. However, the Ge QWs are compressively strained, which increases the energy difference between Γ and L valleys and enhances the unfavorable electron scattering from direct Γ to indirect L valleys. Thus the gain lifetime is <100 fs for compressively strained Ge/SiGe QWs. Note that in the case of bulk Ge, the direct-to-indirect valley scattering time is about 230 ± 25 fs [39]. It is possible to reduce the Γ→L scattering rate with n-type doping and tensile-strain stressors to provide a longer gain lifetime, as suggested by the observation of the favorable L-to-Γ intervalley scattering in tensile-strained n+ Ge in Fig. 2(a)[32]. Wang et al. investigated the inherent optical gain from the direct gap transition of the wafer-bonded Ge-on-insulator (GeOI) and epitaxial n+ Ge-on-Si using femtosecond transmittance spectroscopy captured before the direct-to-indirect valley scattering (<250 fs)[40]. A large inherent direct gap optical gain of 2500 cm?1 was reported in GeOI at an injected carrier density of 1019 cm–3 before Γ-to-L intervalley scattering. For 0.25 % tensile-strained Ge-on-Si with n = 1 × 1019 cm?3 doping, it was found that the inherent gain from the direct gap transition is 25 times larger than the steady-state gain (1300 versus 50 cm?1). The gain spectrum was broader than GeOI although the peak gain was smaller. These results suggest that reducing Γ→L or enhancing L→Γ inter-valley scattering may significantly increase the optical gain of Ge lasers. Indeed, Xu et al.[41] observed a very large transient gain of 5300 cm?1 at 1700 nm with more heavily doped Ge of n = 4 × 1019 cm?3.

class="figure_img" id="Figure2"/>
Download
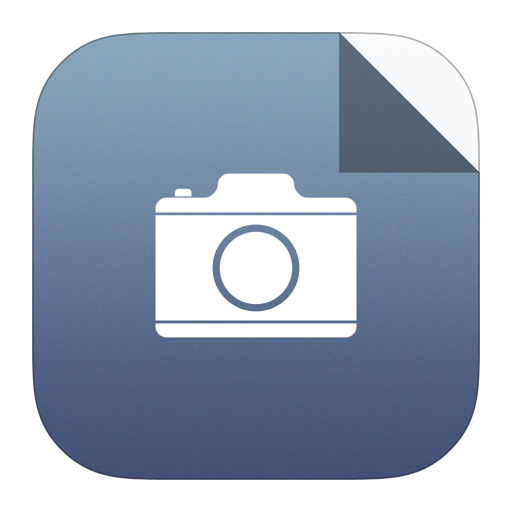
Larger image
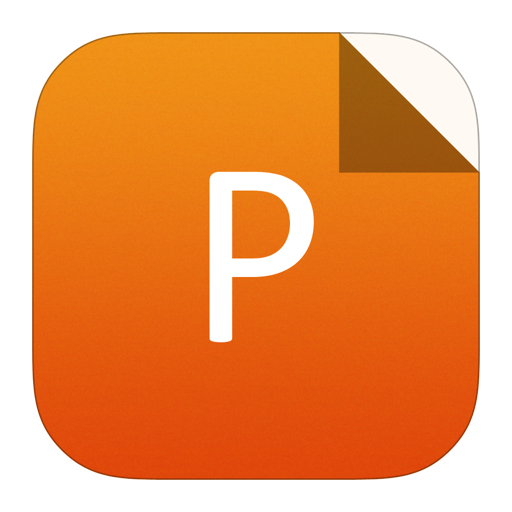
PowerPoint slide
Figure2.
(Color online) (a) Absorption spectrum of 0.25% tensile strained n+ Ge on Si, n = 1 × 1019 cm?3. Regions I–IV are dominated by intra-L-valley FEA, L-to-Γ intervalley scattering absorption (IVSA), indirect gap absorption, and direct gap absorption, respectively. The inset schematically shows intra-L-valley FEA versus IVSA from the L to the Γ valley. Reprinted from Ref. [32] under the Author’s (Wang and Liu’s) Copyright Transfer Agreement with the OSA. (b) Comparison to FEA in n-GaAs, which also demonstrates IVSA except that for direct bandgap GaAs the direction is from Γ to L valleys, opposite to Ge. Reprinted from Ref. [33] with permissions from American Physical Society (APS). (c) FEA coefficient αFEA as a function of injection level at λ = 1550 nm from the first principle model in comparison to the Drude model. The Drude model overestimates the FEA by one order of magnitude. (d) Refined modeling of net gain versus injection level for 0.25% tensile strained Ge with n = 4.5 × 1019 cm?3 at λ = 1700, 1650, 1600, and 1550 nm. The model considers band gap narrowing (BGN) and uses FEA from the first principle model instead of the Drude model. A broad gain spectrum from 1700 to 1550 nm is predicted for an injection level of > 5 × 10 19 cm?3. (c) and (d) are reprinted with permission from Ref. [34]. ? 2013 IEEE.
Optically-pumped, band-engineered Ge lasers have also been demonstrated at room temperature by different groups[42, 43]. Liu et al. demonstrated a device consisting of selectively grown multimode n+ Ge waveguides with mirror polished facets based on the same fabrication method as their Ge microdisk gain media[35]. The length of the waveguides was 4.8 mm to guarantee a mirror loss of

Very recently, Yako et al. achieved threshold behavior of edge emission from band-engineered Ge waveguides at a much lower optical pumping power density from a "reverse-rib" Ge epi-structure in combination with an ex-situ P diffusion for heavily n-type doping, shown in Figs. 3(c) and 3(d)[43]. The novel SiO2 patterning before Ge selective epitaxy greatly reduced the dislocation density. This improvement in material quality may have contributed to the lower threshold of 8 kW/cm2 compared to 30 kW/cm2 in Ref. [42]. The threshold is also in the same order as the theoretical modeling in Ref. [29]. The threshold behavior could be due to amplified spontaneous emission (ASE) or lasing. Yako et al later determined that the emission was ASE due to the relatively large mirror losses of the defective facets.
(C) Electrically pumped Ge lasers
The earlier studies of band-engineered Ge material lay solid foundations for the development of electrically-pumped Ge-on-Si lasers, the ultimate goals for practical applications in Si photonics. Ge-on-Si light emitting diodes (LEDs) based on direct gap transition have been demonstrated at room temperature since late 2000 s[44–47]. However, the electrical injection into the heavily-doped Ge active layer for lasing poses two key challenges: (1) to overcome the optical losses in heavily doped electrodes; and (2) to achieve high efficiency injection of holes into n+ Ge since the hole concentrations tends to decay rapidly with the distance from the junction due to recombination with electrons in n+ Ge. Adequate n+ Si/n+Ge/p+ Si double heterojuction (DH) structure is proposed to address these issues[28, 48]. For an active n-type doping level of 5 × 1019 cm?3, it is found that the n+ doping and applied forward bias creates a band bending that overwhelms the band offset effect, resulting in a pseudo Type I alignment. At a forward bias of 1.2 V on the junction, approximately 6 × 1018 cm?3 holes can be injected into the n+Ge material and the separation between quasi-Fermi levels of electrons and holes exceeds the direct band gap, indicating population inversion[28].
Based on the same DH device design structure, the first electrically-pumped laser with Fabry-Perot cavity was demonstrated in 2012[18]. To achieve the n-type doping level of >4×10 19 cm?3, delta-doping plus drive-in diffusion method was adopted without deteriorating the material quality[49]. As the current density increases, a clear threshold can be observed along with a sharp emission line with less than 1.2 nm linewidth. Figs. 4(a) and 4(b) show a representative emission spectrum and the corresponding L–Icurve of an electrically pumped Ge-on-Si laser diode (LD) at room temperature, respectively. The observed maximum output power of these lasers is 8 mW at room temperature. Depending on the thickness of the Ge layer and corresponding modal losses due to FCA in the electrodes, the clamping conditions of lasing vary accordingly and lasing wavelengths in a broad spectrum range of 1530–1700 nm have been observed (Figs. 4(c)–4(e)). Such a broad gain spectrum is consistent with the theoretical modeling shown in Fig. 3(d) that took into account the BGN effect and the corrected FEA value. The internal quantum efficiency (IQE) of this prototype Ge-on-Si diode is estimated to be
m IQE} = {eta _{
m slope}}left( {frac{{{alpha _{
m i}} + {alpha _{
m m}}}}{{{alpha _{
m m}}}}}
ight) sim 30% $


class="figure_img" id="Figure4"/>
Download
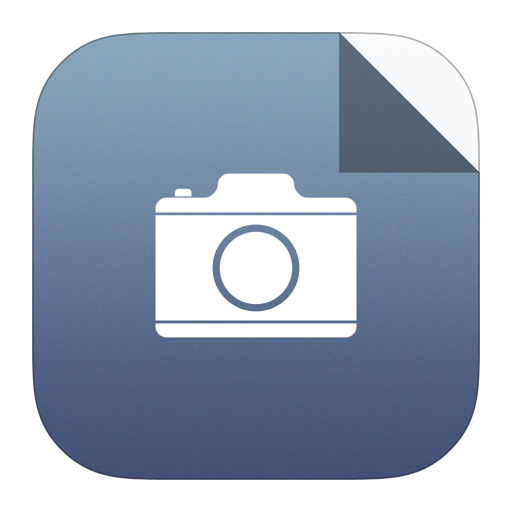
Larger image
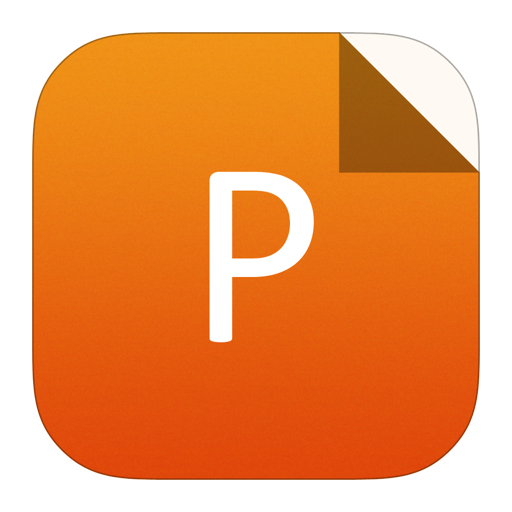
PowerPoint slide
Figure4.
(Color online) (a) A typical emission spectrum of an electrically pumped band-engineered Ge-on-Si laser diode. The inset schematically shows the cross-section of the device. The width of the Ge waveguide is 1 μm and the length is 270 μm. (b) L–I curve for the 270 μm long waveguide device. 40 μs-long electrical pulses with 1 kHz repetition rate was used for electrical injection. Spectra of Ge lasers with different Ge layer thicknesses are shown in (c), (d) and (e). All panels are reprinted from Ref. [18] with permission of OSA ?2012.

class="figure_img" id="Figure3"/>
Download
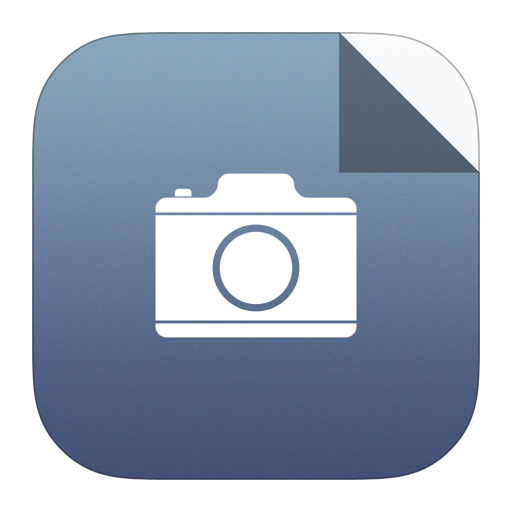
Larger image
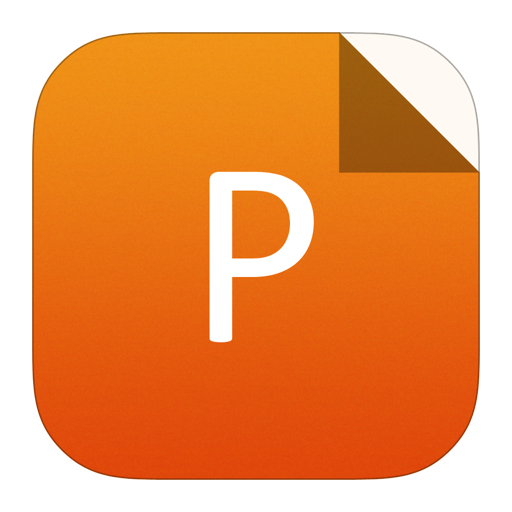
PowerPoint slide
Figure3.
(Color online) (a) Edge-emission spectra of a Ge-on-Si waveguide with mirror polished facets at different optical pumping levels. With the increase of pumping pulse energy the spectrum evolves from broad spontaneous emission to sharp lines of stimulated emission. The inset shows a cross-sectional SEM picture of the Ge waveguide. (b) Integral emission intensity from the waveguide facet versus optical pump power showing the lasing threshold. (a) and (b) are reprinted from Ref. [42] under the Author’s Copyright Transfer Agreement with the OSA (c) Schematic illustration showing optical gain measurement setup in Ref. [43]. (d) Typical edge-emitted optical power from the n+-Ge reverse-rib waveguide structure in Ref. [43] as a function of input pumping laser power. The inset shows a cross-sectional SEM image of the reverse Ge rib waveguide structure. (c) and (d) are reprinted with permission from Ref. [43]. ? 2016, the Electrochemical Society (ECS).
Recently, Li et al.[50] analyzed electrically-pumped edge emitting Ge laser structures in detail. Using modeling parameters that well fit the L–I curve in Ref. [18], they found that the threshold current density can be decreased by 20 × with optimized device structure and cavity design. Assuming a defect-limited carrier lifetime of 100 ns, a wall-plug efficiency of 15% and slope efficiency of 24% at 1 mW output is predicted, where the threshold current density of 2.8 kA/cm2 is comparable to III–V DH lasers. Here the 100 ns defect-limited carrier lifetime may be the biggest challenge since so far the lifetime measured by time-resolved photoluminescence (TR-PL) or RF photoconductivity decay (RF-PCD) is limited to a few ns at room temperature[51–53], meaning the threshold would increase by ~10× according to the modeling in Ref. [50]. This issue could be better addressed with improved growth methods aiming at reduced Ge/Si interfacial defects, as will be briefly discussed at the end of this section. It could also be improved by further optimizing the device structure for more efficient carrier injection. We will give such an example next.
While the first electrically pumped Ge laser was fabricated by chemical vapor deposition (CVD), in 2015 Koerner et al. further demonstrated electrically pumped Fabry-Perot n+ Ge-on-Si laser grown by molecular beam epitaxy (MBE)[54]. This demonstration confirms that the n-doping approach for optical gain is universal to Ge materials irrespective of the growth method. It also confirms the basic principles of band-engineered Ge lasers against some ambiguities in different theoretical considerations. Very recently, the same group demonstrated a semiconductor optical amplifier (SOA) as well as stimulated emission at a notably reduced threshold current density using a more elaborate p+-Si/p+-Ge/n-Ge/i-Ge/p-Si structure. In this device, Zener tunneling junction was formed between p+-Ge (with 1020 cm?3 B doping) and n-Ge (with 0.8 × 1019 ?2 × 1019 cm?3 Sb doping)[19, 55]. The aim is to achieve much more efficient electron injection into the direct Γ valley utilizing the much larger non-photon direct band-to-band tunneling (BTBT) probability compared to indirect BTBT[56]. During operation, the p–i–n junction is under forward bias, while the p+-Ge/n-Ge tunneling junction is under reverse bias. Holes are injected from p-Si through i-Ge to n-Ge, while electrons are tunneled from top p+-Ge to n-Ge (upper inset of Fig. 5(a)) mostly via direct BTBT to populate the Γ valley, thereby enhancing population inversion of the direct gap transition. The results of the electrically pumped Ge SOAs and stimulated emission based on Zener tunneling junction are presented in Figs. 5(b) and 5(c). In testing the SOA using a non-coherent broad band light source (1660–1672 nm) as an optical input, stimulated emission under electrical pumping was observed in a 1 mm-long and 1.6 μm-wide waveguide at a threshold of 90 mA (~5.6 kA/cm2), representing ~50× decrease in threshold current density compared to the first electrically pumped Ge laser in Ref. [18]. Koerner et al. also reported mono-mode lasing in a 3.2 mm waveguide under 110 mA electrical pumping and 0.4 mW non-coherent optical input, with an output power of 1.65 mW (or 4.7 dB optical gain) from the SOA[19]. These new results indicate that improvement in device structures for more efficient electron injection into the Γ valley greatly enhances the performance of Ge lasers and SOAs.

class="figure_img" id="Figure5"/>
Download
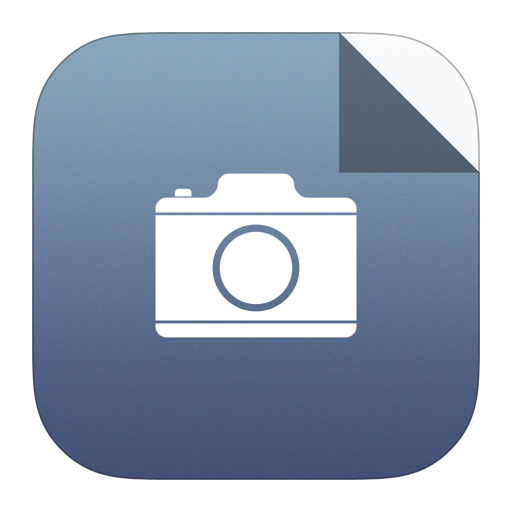
Larger image
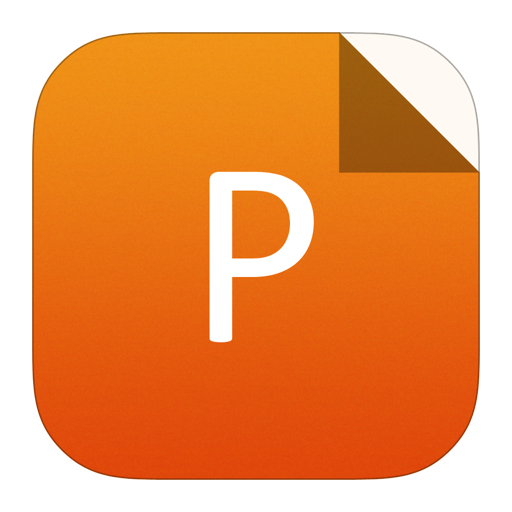
PowerPoint slide
Figure5.
(Color online) (a) Ge tunneling junction for direct Zener-BTBT injection. The direct onset is visible by a small kink under reverse bias. (b) Optical amplification by the Zener-Emitter under electrical pumping for a 1 mm long, 1.6 μm wide device. (c) Mono-mode lasing at 0.4 mW optical signal input, 110 mA electrical pumping (3.2 mm length) and 1.65 mW output power at room temperature. Reprinted with authors’ permission from Ref. [19].
The demonstration of Ge lasers is a breakthrough in the Si monolithic lasers. Based on the results from the prototype device, there are a number of approaches to further enhance the performance of Ge lasers in future research. (1) Optimize the balance among tensile strain, n-type doping, and Sn alloying. While each of these 3 band engineering methods has its limitation, an optimal combination of the 3 may provide an optimal solution. For 0.25% tensile strained Ge, simulations have shown that if the n-type doping is increased to >7 × 10 19 cm?3 the threshold current density can be decreased below 1 kA/cm2 at 1600–1700 nm, comparable to III–V double heterojunction lasers[34]. If a few percent Sn is incorporated, the n-type doping level can be further decreased and the performance improved by reducing the FEA and Auger recombination process. Note that there should be an optimal Sn alloying concentration for this purpose, since too much Sn incorporation could drastically reduce the direct band gap and increase the Auger coefficient. (2) Bufferless selective growth of high quality n+ Ge in narrow trenches ≤1 μm in width[57, 58]. This method prevents dopant segregation and improves the material quality at the Ge/Si interface by eliminating the highly defective low-temperature buffer layer, thereby enhancing the carrier injection efficiency in Ge LDs. As shown in Ref. [52], Ge/Si interfacial defects is the dominant factor reducing the carrier lifetime in thin Ge layers. Therefore, combining bufferless selective growth with the novel SiO2 patterning method in Ref. [43] may greatly increase the defect-limited carrier lifetime beyond 10 ns and approach the prediction for optimized edge emitting Ge lasers in Ref. [50]. Nanopatterned Ge microdisk laser is another possible route, considering that Ge/Si epitaxy without misfit dislocations has been achieved using this method very recently[59]. (3) Novel laser structure or configuration for efficient carrier injection, particularly for heavily-doped active layer. The direct BTBT injection mentioned earlier is such an example. In the long run, Ge/SiGe QW structures are necessary to achieve low threshold lasing. To compensate the detrimental compressive strain in QWs, stressors such as Si3N4 can be applied, as has been demonstrated in Ref. [37] on Ge waveguides.
Another interesting possible approach is to exploit the optimal carrier dynamics between Γ versus L valleys for on-chip laser sources without active cooling[9]. Different from direct bandgap III–V semiconductors, the pseudo-direct gap configuration of band-engineered Ge can enhance the direct gap emission efficiency at high temperature and high injection levels due to enhanced L-to-Γ valley excitation or scattering, as evidenced from the increase in the integrated PL intensity with the increase in temperatures[17, 60] and L-to-Γ intervally absorption[32]. Since the Auger recombination rate in indirect valleys is orders of magnitude smaller than that in the direct valleys due to the momentum mismatch, the same reason as their lower radiative recombination efficiency, it may be beneficial to “store” the injected electrons in the L-valleys and supply it to the direct Γ valley via the fast intervalley scattering process (sub-ps). This approach makes full use of the unique bandgap structure of the band-engineered Ge to optimize the efficiency as well as the high temperature performance. Recent progress in Sn alloying and tensile strain will allow us to systematically change the relative energy positions between L and Γ valleys for this investigation, as will be discussed in the next section.
3.1.2
GeSn lasers
A promising approach to completely overcome the indirect band gap nature of Ge is alloying with Sn for transformation into a direct bandgap semiconductor. The realization of GeSn lasers could also unlock the potential opportunities of Si photonics in MIR applications, including sensing/environmental monitoring, free-space data transmission (e.g. in the atmospheric window of λ = 3–5 μm), and RF photonics in the >2000 nm optical band to avoid two-photon absorption (TPA) in Si waveguides [25, 61]. GeSn alloys with sufficient Sn concentrations were predicted to be a direct bandgap semiconductor dating back to 1980s[62]. But the large lattice mismatch with Si, low equilibrium Sn solubility (~1 at.%), and the tendency of Sn surface segregation of epitaxial GeSn films impede the development of GeSn active photonic devices. It was only in the past two decades that GeSn research gains momentum thanks to the improvement in film quality via advanced deposition tools and techniques. Recently, optically pumped GeSn lasers at low temperatures were fabricated with Fabry–Perot[20, 21, 63] and microdisk cavities[64]. Yet it is still very challenging to achieve room-temperature electrically pumped GeSn lasers. This section covers recent progress in GeSn epitaxy, material characterization and laser performance. The obstacles and methods for electrical GeSn laser are discussed as well.
(A) Effect of Sn composition and strain on the indirect-to-direct bandgap transition
The Sn concentration x in Ge1–xSnx for its transition from indirect to direct bandgap semiconductor is somewhat inconsistent in literature, ranging from 0.065 to 0.10 depending on the strain in the films[65–68]. Fig. 6(a) maps the energy difference between the indirect and direct gaps (EgL?EgΓ) as a function of tensile strain and Sn composition for (100) oriented Ge1–xSnx based on the methods and parameters described in Refs. [9, 17]. The corresponding direct band gap EgΓ is mapped in Fig. 6(b). When (EgL?EgΓ) becomes positive, the material becomes a direct gap semiconductor. Note that the effect of strain could be underestimated in Fig. 6 since our recent measurement on (111) oriented GeSn crystallized on silica indicated a larger dilatational deformation potential of a = ?12.8 ± 0.8 eV for x ~ 0.08[69], larger than the deformation potential of ?8.97 eV in the modeling. Even with this conservative estimate, 10 at. % Sn alloying is sufficient to achieve the indirect to direct gap transition for relaxed GeSn. However, this is still 10×larger than the equilibrium solubility limit of ~1 at.%, which poses a challenge for the material growth.

class="figure_img" id="Figure6"/>
Download
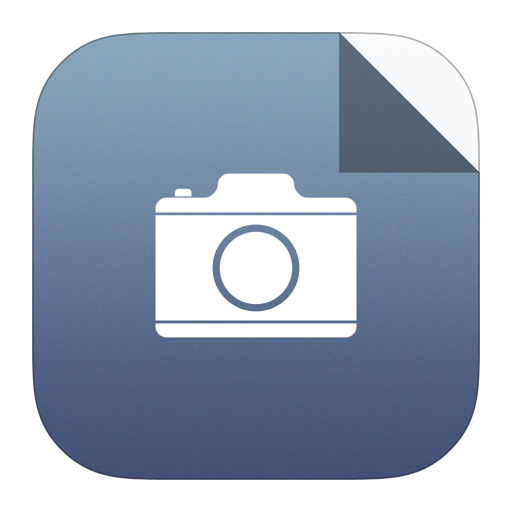
Larger image
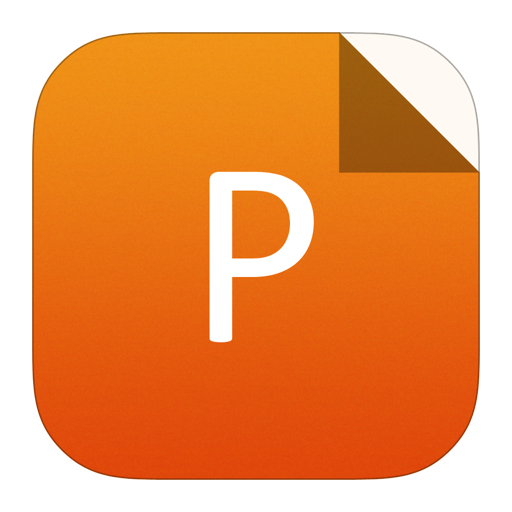
PowerPoint slide
Figure6.
(Color online) (a) Energy difference between the indirect and direct gap (EgL–EgΓ) versus biaxial tensile strain and Sn atomic percentage for (100)-oriented GeSn. (b) Direct band gap EgΓ as a function of biaxial tensile strain and Sn atomic concentration. Adapted from Ref. [9] with permission under MDPI’s Open Access Information and Policy.
(B) GeSn epitaxy: effect of CVD precursors and strain on Sn incorporation
Great efforts have been made to grow high-Sn, high quality direct bandgap GeSn films with different deposition tools and precursors. For laser applications, it is paramount to achieve both high Sn composition and strong PL emission at the same time. The development of SnD4 precursor played in important role in GeSn CVD growth in the early days[70]. A remarkable feature of this precursor is that the GeSn film can directly relax on the Si substrate at a deposition temperature of 200–350 °C without the need of a Ge/Si virtual substrate. Most recently, up to 17 at.% fully strained Ge1-xSnx films were demonstrated by combining high order Ge hydride compound Ge4H10 with SnD4 precursor to reduce the surface mobility and enhance the sticking coefficient[71]. The film quality is good enough to demonstrate the first prototypes of GeSn PDs and LEDs[72, 73]. Similar to epitaxial Ge, annealing has been shown to improve the optoelectronic properties of GeSn by reducing the defect density for both CVD and MBE grown GeSn materials[74, 75]. Rapid thermal annealing (RTA) is preferred in this case to remove the defects without causing Sn segregation.
Despite of its success, a significant drawback of SnD4 precursor is its instability, which requires great precautions in shipping, storage and utilization. In recent years, GeSn CVD growth on epitaxial Ge/Si virtual substrates using industrially available precursors has been successfully developed. However, in these cases the epitaxial GeSn alloy naturally incorporates compressive strain in the film due to the lattice mismatch between GeSn and Ge. The compressive strain enlarges the energy difference between Γ and L valleys, thus requiring more Sn incorporation than relaxed GeSn films for the indirect to direct gap transition.
Reduced pressure CVD (RPCVD) with Ge2H6 and SnCl4 precursor gases has been developed with a growth temperature below 400 °C to achieve high quality Ge1–xSnx on Ge/Si. It has been suggested the exothermic reaction between the hydride and the chloride precursors may have contributed to better material quality[76]. In the first report of optically pumped GeSn laser, the active GeSn films grown by RPCVD were as thick as 560 nm with 12.6 at.% Sn incorporated[20]. The films were 75% relaxed with ?0.57% residual compressive strain.
Margetis et al.[77] further demonstrated GeH4 as a feasible Ge precursor in their RPCVD chamber due to its lower cost and much broader application in the high-volume semiconductor foundries compared to Ge2H6. GeSn epitaxy on Ge/Si virtual substrates using GeH4 and SnCl4 precursors achieved up to 12 at.% Sn and ~1000 nm thickness with H2 and N2 carrier gases[77], compared to ~15% using Ge2H6 or Ge3H8 as precursor[78]. The 10 at.% Sn material grown with GeH4 precursor also achieved optically pumped lasing up to 110 K[63].
Interestingly, by further incorporating compositionally graded GeSn buffer layers on Ge/Si virtual substrate to relax the compressive strain, the highest Sn composition has reaches 17.4 at.% on top of 15.5 at.% Sn (2nd buffer) and 12 at.% Sn (1st buffer) layers[21] using GeH4 and SnCl4 precursors. This finding echoes the results in Ref. [69] showing that the increase in tensile strain can effectively reduce Sn segregation even at high temperatures >600 °C. Therefore, a highly important message from these recent studies is that Sn incorporation is not only related to the precursor chemistry, but also highly sensitive to strain. Strain relaxation or tensile strain could notably increase the incorporation of Sn in epitaxial GeSn thin films. Research is underway to check if there is an upper limit of Sn incorporation using the graded buffer layer approach.
(C) Optically pumped GeSn lasers
The high Sn composition, high quality direct gap Ge1-x-Snx film enables the breakthrough of optically-pumped GeSn lasers. Table 2 summarizes the recent progress in Ge1-xSnx lasers. The first edge-emitting Ge0.874Sn0.126 laser operates up to 90 K with a threshold power density of 325 kW/cm2 at 20 K[20]. A year later the same group led by Grutzmacher and Buca reported Ge0.875Sn0.125 microdisk lasers with the threshold reduced to 130 kW/cm2 at 20 K and the operating temperature increased up to 130 K[64]. Fig. 7 shows the temperature-dependent emission spectra and light output versus light input (L–L) curves of the GeSn microdisk lasers with 8.5 at.% (sample A) and 12.5 at.% Sn (sample B). The undercutting of Ge buffer layer increases the mode confinement within the GeSn gain media and helps to reduce the detrimental compressive strain from ?0.7% to ?0.4%, thereby improving laser performance compared to Fabry-Perot structures. The atomic layer deposition of Al2O3 layer also provides better surface passivation. In 2016, Yu’s team[63] also reported optically-pumped edge-emitting GeSn laser with a lower Sn concentration of 10.9% yet lower threshold. The factors leading to this achievement are: (1) the use of low cost GeH4 as Ge precursors provides a favorable strain relaxation, improving the material quality and directness of the bandgap; (2) the thicker GeSn film (~1000 versus 500 nm) and thinner Ge buffer layer (700 versus 2500 nm) compared to Ref. [20] offers a better optical confinement in GeSn layer and thus increases the modal optical gain; (3) the Ge/GeSn/Ge DH structure provides carrier confinement. In July 2017, Yu’s team further reported optically pumped lasing covering the entire spectral range of 2000–3000 nm from GeSn lasers of different Sn compositions from 8 to 17.4 at.%. Using the 17.4 at.% Sn active region mentioned earlier in the graded buffer layer growth, maximal lasing wavelength is pushed to 2950 nm at 180 K[21]. Higher Sn composition leads to higher operation temperature, most likely due to more directness in the bandgap. However, the threshold pumping power density is not decreased and the maximal lasing temperature is limited to 180 K.

class="figure_img" id="Figure7"/>
Download
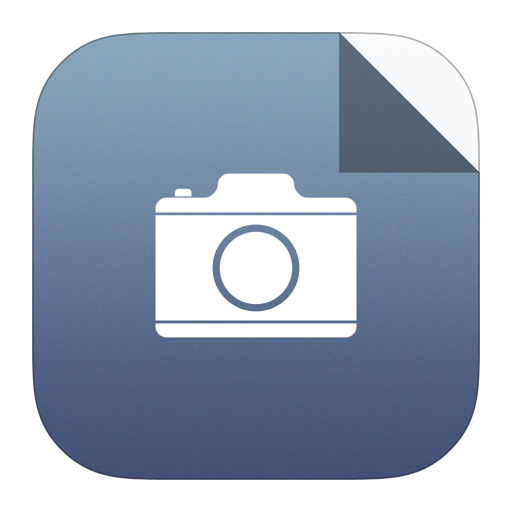
Larger image
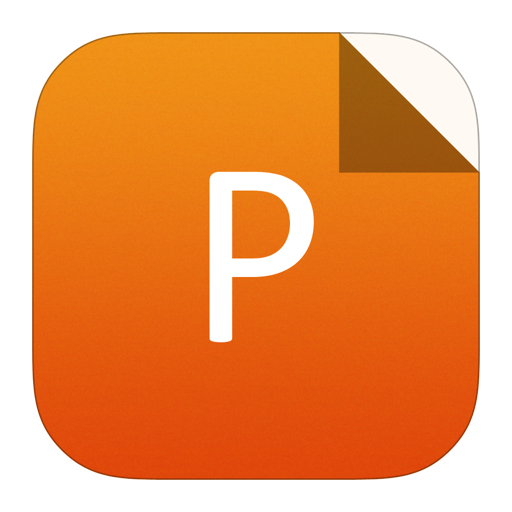
PowerPoint slide
Figure7.
(Color online) Power and temperature dependence of GeSn lasers for different Sn contents. (a) Temperature-dependent spectra of 8 μm diameter microdisks from samples A (8.5 at.% Sn) and B (12.5 at.%) at 820 kW/cm2 pump power density. L–L curves at different temperatures are shown in (b) for sample B and (c) for sample A. (d) Schematics of the GeSn microcavity structure. Reprinted with permission from ACS Publications ?2016[64].
Year | xSn (at.%) | Strain (%) | Laser structure | Threshold (kW/cm2) | Lasing wavelength (μm) | Operating temperature (K) |
2015 Wirths et al.[20] | 12.6 | ?0.71 | Fabry–Perot cavity | 325 at 20 K | 2.3 | ≤ 90 |
2016 Stange et al.[64] | 12.5 | ?0.4 | Microdisk | 130 at 20 K | 2.5 | ≤ 130 |
2016 Al-Kabi et al.[63] | 10.9 | – | Fabry–Perot | 68 at 10 K | 2500 | ≤ 110 |
2017 Margetis et al.[21] | 17.4 | ~0 for the top layer | Fabry–Perot | 171 at 77 K | Up to 2.900 at 77 K; 2.945 at 180 K | ≤ 180 |
Table2.
Recent progress in optically pumped Ge1-xSnx lasers.
Table options
-->
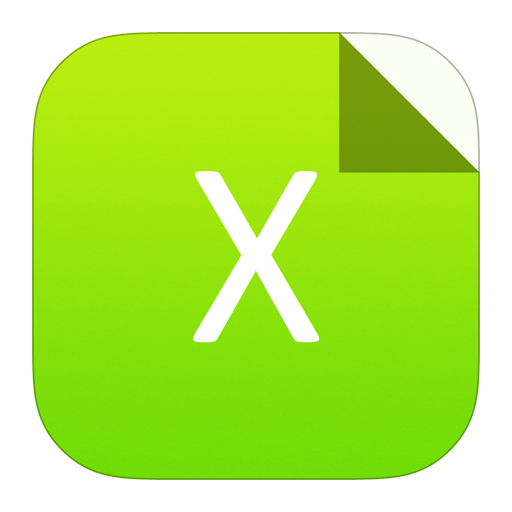
Download as CSV
Year | xSn (at.%) | Strain (%) | Laser structure | Threshold (kW/cm2) | Lasing wavelength (μm) | Operating temperature (K) |
2015 Wirths et al.[20] | 12.6 | ?0.71 | Fabry–Perot cavity | 325 at 20 K | 2.3 | ≤ 90 |
2016 Stange et al.[64] | 12.5 | ?0.4 | Microdisk | 130 at 20 K | 2.5 | ≤ 130 |
2016 Al-Kabi et al.[63] | 10.9 | – | Fabry–Perot | 68 at 10 K | 2500 | ≤ 110 |
2017 Margetis et al.[21] | 17.4 | ~0 for the top layer | Fabry–Perot | 171 at 77 K | Up to 2.900 at 77 K; 2.945 at 180 K | ≤ 180 |
The breakthrough in GeSn lasers is inspiring more interests and efforts to achieve room-temperature, electrically pumped lasers. However, several fundamental questions remain to be answered:
(1) Why is the threshold so high even at <20 K? At <20 K, the lowest threshold demonstrated so far is on the order of 50 kW/cm 2[63]. Converting to the number of injected electron-hole pairs for the pumping wavelength of 1064 nm, this roughly corresponds to ~50 kA/cm2 of electrical injection. This is 3 orders of magnitude higher than early homojunction GaAs lasers with a typical threshold of <0.1 kA/cm 2 at <20 K [79]. This high threshold could be related to insufficient directness in the bandgap of GeSn since the difference between the direct and indirect gaps are tens of meV at best in existing GeSn lasers. For example, drastic increase in threshold current density has been observed in GaAs1–xPx lasers at 77 K for x > 0.35, which is still direct gap (for x < 0.45) but near the indirect-to-direct transition [80]. In this case more complicated carrier distribution among indirect, direct, and deep donor/defect levels could occur, which drastically impact the threshold current density. On the other hand, recent results in Ref. [21] show that although the increase in Sn composition improves the directness and the maximal lasing temperature, the threshold is actually not decreased. Therefore, other factors such as material quality and enhanced Auger recombination with the decrease of direct bandgap has to be taken into account.
(2) What is the limit of Sn incorporation in GeSn epitaxy? As discussed earlier, strain relaxation and tensile strain seem to play a very important role in enhancing Sn incorporation into the GeSn thin films. Graded buffer layers has been applied to achieve 17.4 at.% Sn recently. It is interesting to investigate how far we can go using this approach. While higher Sn composition transforms GeSn more towards direct gap semiconductor, it is also more thermodynamically unstable. Can we achieve high enough Sn composition such that GeSn is truly comparable to III–V semiconductors such as InAs? This question needs further investigations to answer. Our understanding of the fundamental band structures of GeSn also needs to be tested against experiments for Sn composition >20 at.%.
To further enhance the performance of GeSn lasers, possible approaches include improving the material quality of high Sn composition thin films, incorporating tensile strain to enhance the indirect-to-direct bandgap transition, leveraging n-type doping, and incorporating SiGeSn/GeSn QW or QD structures. Currently, optically pumped SiGeSn/GeSn QW lasers have been demonstrated yet the threshold reduction is not as significant as one would expect from preliminary studies (i.e. not an order of magnitude improvement) presumably due to insufficient quantum confinement[81]. Considering the success in integrating large lattice-mismatch InAs QD lasers on Si, as will be discussed in Section 3.1.3, eventually GeSn QD may also be a promising solution in the future although one has to be aware of the possibility of enhanced Sn surface segregation in this case.
The fundamental understanding of the behavior of the direct bandgap transition and carrier dynamics in GeSn will also be helpful to GeSn laser development. Thus far, strictly speaking there is still little direct proof showing GeSn films are direct gap semiconductors at room temperature. The increase of the integrated PL intensity with decreasing temperatures is now applied as a compelling proof that the fundamental bandgap of epitaxial Ge1–xSnx (x > 0.07) is direct [20, 64, 82, 83], in contrast to the decrease in direct gap PL intensity with temperature in pure Ge. However, it is found that there are possible pitfalls associated with this claim, i.e., optically-active dislocations in Ge1–xSnx epitaxially grown on Ge buffer layers show a similar temperature-dependent PL behavior in spite of the indirect nature of Ge1–xSnx[84]. Therefore, the direct measurement of the carrier dynamics and gain lifetime is needed to resolve the directness of the fundamental bandgap of Ge1–xSnx without any ambiguity in near future. Recently, Wang et al. demonstrated an optical transient gain lifetime of ~100 ps from crystallized 0.2% tensile strained Ge0.91Sn0.09 at room temperature[85]. Considering that the gain lifetime is 3 orders longer than that of indirect gap Ge QWs[38] and bulk Ge[39] mentioned earlier (~100–200 fs), it is a good evidence supporting that 9 at.% Sn alloying and 0.2% tensile strain results in indirect-to-direct gap transition. More carrier dynamics studies are needed to undoubtedly reveal the details about indirect-to-direct transition and its impact on optical gain and threshold.
3.1.3
1310 nm InAs QD lasers on Si platform
Since Group IV lasers are still in the early stages of development, for practical applications in the near future III–V lasers are still the dominant choice so far. Hybrid III–V lasers on Si has achieved great progress within the past decade, where III–V wafers/chips are bonded to Si for laser processing and coupling to Si waveguides[15, 86]. To achieve large scale integration, though, this approach is limited by the wafer size mismatch between III–V (maximal 150 mm diameter) and Si wafers (200 or 300 mm diameter in CMOS foundry). Therefore, in recent years the research in this field has shifted towards growing 1310 nm InAs QD lasers on Si wafers[87–89], potentially allowing 300 mm diameter bonding between the laser wafer and the CMOS wafer.
III–V QD lasers have demonstrated much higher thermal stability as well as lower threshold compared to their QW counterparts due to the discrete energy levels and lower density of states, as has already been commercialized by Fujitsu[90]. The confinement of carriers in QDs also reduces their interaction probability with defects[89]. This is ideal for on-chip laser applications, which requires low threshold, high efficiency, and stability up to 80 °C. The first integration of 1310 nm InAs QD lasers on Si was achieved by Arakawa’s group, the inventor of semiconductor QD lasers, using wafer bonding approach .
Over the past few years, great progress has been made in growing InAs QDs on off-axis Si wafers. The choice of off-axis Si is made to avoid antiphase boundaries upon III–V epitaxy. Note that these substrates are not on-axis Si (100) substrates used in electronics, so the grown InAs QD laser need to be bonded to CMOS electronics wafer later on. The QDs usually require MBE growth to achieve high quality, which may need more considerations in process integration, as will be discussed later. The first demonstration of InGaAs QD lasers grown on Si was achieved in 2006[92], yet the emission wavelength was around 1000 nm instead of optical communication wavelengths. As an intermediate step towards integration on Si, InAs QD lasers were first monolithically grown on Ge substrates by Liu et al.[93] since Ge/Si virtual substrates had already achieved significant progress in 2000s. A very low threshold of 55 A/cm2 was demonstrated. Around the same time, the same group also attempted direct integration of InAs QD lasers on Si via GaAs nucleation layer, buffer layers and InGaAs/GaAs dislocation filter layers[87]. Lasing at 1302 nm was successfully demonstrated with output power up to 26 mW and operation temperature up to 42 °C, yet the threshold current density of 725 A/cm2 is much higher compared to those grown on Ge substrates. A few years later, InAs QD CW lasers with emission wavelengths close to 1300 nm have been demonstrated on GaAs/Ge/off-axis Si (100) virtual substrates (Fig. 8(a))[88]. A trick borrowed from previous research is the thermal annealing of Ge to form biatomic step arrays on the Ge surface to promote proper GaAs nucleation[94]. A typical threshold current density of 400–500 A/cm2 was demonstrated, with output power up to 176 mW and lasing up to 119 °C. This achievement marks a significant step towards practical applications of InAs QD lasers grown on Si.

class="figure_img" id="Figure8"/>
Download
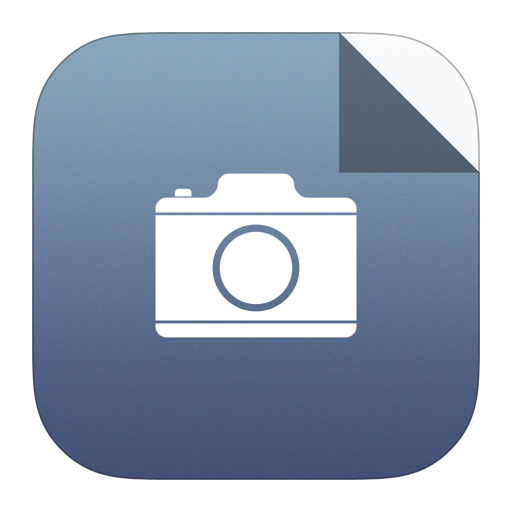
Larger image
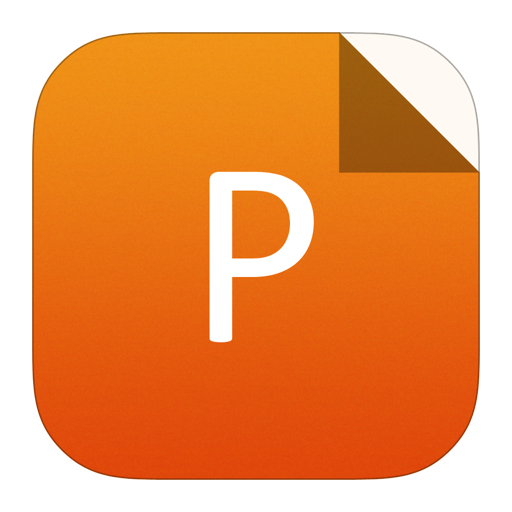
PowerPoint slide
Figure8.
(Color online) (a) InAs QD laser structure grown on Ge buffer layer on offcut Si (100) substrate[88]. Reprinted with permission from AIP Publishing, LLC. (b) High resolution cross-sectional transmission electron microscopy (HR-TEM) image of the ultrathin AlAs nucleation layer on off-axis Si(100) substrate. (c) HRTEM image of the strained-layer superlattice structures (SLSs) to filter the threading dislocations. From positions 1-6, the threading dislocation density is decreased from 109 to 105 cm?2. (b) and (c) are reprinted from Ref. [89] with permission from Nature Publishing Group.
In 2016, low threshold InAs QD CW lasers on off-axis Si with >3000 h lifetime has been demonstrated using a different growth approach [89]. As shown in Fig. 8(b), this approach uses a 6.5 nm thick AlAs nucleation layer grown by alternating Al and As4 flux at 350 °C. This is followed by GaAs buffer layers, grown sequentially at 350, 450 and 590 °C with the last stage contributing to the majority of the buffer layer. To enhance dislocation motion and annihilation, strained-layer superlattices (SLSs) comprising 5 periods of 10 nm In0.18Ga0.82As/10 nm GaAs are introduced as dislocation filters, as shown in Fig. 8(c). The relaxation of SLS applies in-plane strain to the threading dislocations, thereby enhancing their gliding and annihilation effectively. Four SLSs separated by 300 nm-thick GaAs spacers are introduced with 4 corresponding in-situ annealing at 660 °C to enhance dislocation filtering. Indeed, from position 1 to 6 (Fig. 8(c)), the threading dislocation density drastically decreases from 109 to 105/cm2, offering high quality template for InAs QD growth. This results in a low threshold current density of 62.5 A/cm2, comparable to InAs QD lasers grown on III–V substrates. An output power up to 105 mW was demonstrated at room temperature, and lasing can be sustained up to 120 °C. This achievement is another milestone in InAs QDs lasers monolithically integrated on Si.
In 2017, CW InAs QD lasers monolithically grown on on-axis Si have been demonstrated using a GaAs buffer layer grown by metalorganic CVD (MOCVD) system[95]. The on-axis Si wafers were baked at 900 °C in purified H2 to promote the formation of 2 × 1 surface reconstruction, followed by a critical rapid cooling to 700 °C within 30 s to preserve the 2 × 1 surface before GaAs epitaxy. This method achieved antiphase boundary free GaAs buffer layers on Si. The rest of the growth was similar to the report in Ref. [89]. Room-temperature CW lasing has been demonstrated with 43 mW single facet output and operation up to 102 °C, yet the threshold is much higher than those grown on off-axis Si (425 A/cm2 versus 62.5 A/cm2).
The rapid progress of high performance InAs QD lasers grown on Si shows great promise for integrated laser sources in the near future. On the other hand, two potential issues need to be considered and addressed. (1) So far all high quality QD lasers are grown by MBE, which tends to have a much lower throughput than industrially standardized CVD epitaxy tools. MBE systems capable of handling 300 mm Si wafers are also rare in industry. It is an open question as to whether MBE would become a limiting factor for throughput, or whether similar techniques could be transferred to industrial standard CVD tools. (2) Growth on CMOS wafers versus bonding to CMOS wafer. While in principle the demonstration of InAs QDs lasers on on-axis Si allows direct growth on CMOS wafers, the complicated buffer layers and thermal process makes it hard to directly incorporate into the start-of-the-art CMOS processing flow. Wafer scale bonding from QD lasers grown on off-axis Si seems to be more attractive due to the higher performance. Yet there has been no demonstration of 300 mm diameter bonding of QD lasers to CMOS wafers with working photonic and electronic circuits so far. The solution of these two potential issues will lead to the practical applications of InAs QD/Si lasers in datacom.
While the Ge, GeSn and InAs QD lasers discussed in this section are still discrete devices, they can be readily integrated with Si-based waveguides using existing coupling methods, such as evanescent coupling demonstrated in hybrid III–V lasers[86] and inverse taper mode couplers/converters. Compared to off-chip, fiber-pigtailed laser sources, the on-chip lasers take advantage of the mature Si fabrication process for high-volume, high-yield electronic-photonic integration at low cost. They also offer the flexibility of wavelength division multiplexing in future generations of multicore processors for high performance computing.
3.2
Optical modulators
3.2.1
GeSi and Ge EAMs
(A) FKE versus QCSE in Ge and GeSi
As mentioned in Section 1, EAMs have been developed in the past decade for Si photonics based on the EAE of the direct gap transition of Ge or GeSi, which includes FKE in bulk materials and QCSE in QW structures. For FKE, the applied electric field causes band tilting (comparing Fig. 9(a) with 9(b)), which allows a photon with hν < Eg to excite an electron from the valence band to the conduction band through a cooperative tunneling process across a triangular energy barrier (Fig. 9(b)). Therefore, the absorption coefficient at hv < Eg increases compared to the case without the electric field (Fig. 9(c)). On the other hand, for hv slightly greater than Eg the absorption curve shows oscillating behavior near the band edge (Fig. 9(c)), known as Franz-Keldysh oscillations, because the probability to cross the triangular barrier is determined by the interference between the electron wavefunction and the barrier. This phenomenon is well known in quantum tunneling (Fig. 9(d)) and it is similar to the Fabry–Perot oscillations in optics, both resulting from the nature of waves.

class="figure_img" id="Figure9"/>
Download
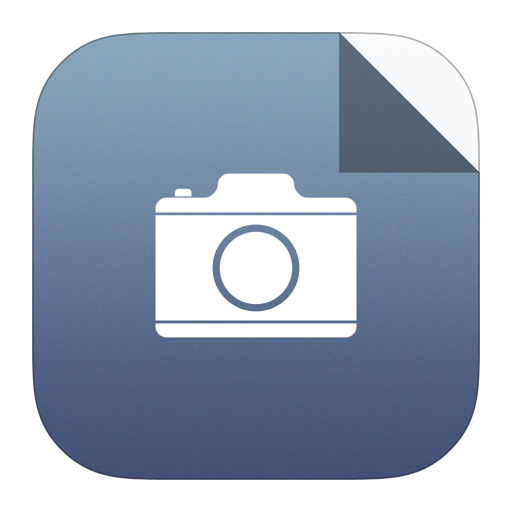
Larger image
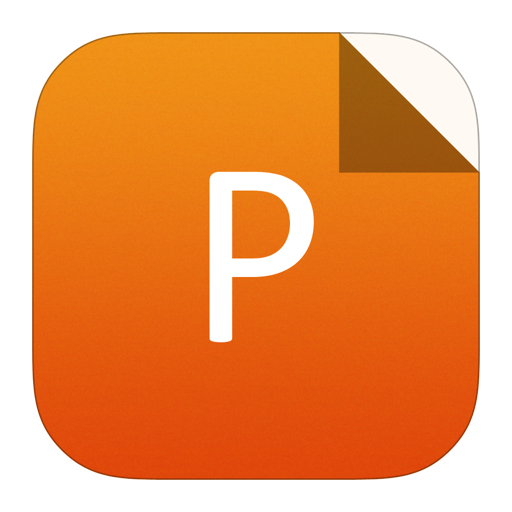
PowerPoint slide
Figure9.
(Color online) (a) Schematics of band-to-band optical absorption in a bulk semiconductor material (a) without an applied electric field; and (b) under an applied electric field (Franz-Keldysh effect). (c) Corresponding absorption spectra of the bulk semiconductor material with and without an electric field. (d) The transmission probability through a rectangular potential barrier with a height of V0 = 0.1 eV and a width of 4.5 nm as a function of the electron energy normalized to V0. The finite tunneling probability at E < V0 and oscillations at E > V0 closely resemble the features of the absorption spectrum under an electric field in (c). Panels (a–c) are reproduced from Ref. [9] with permission under MDPI’s Open Access Information and Policy. Panel (d) is reproduced from Ref. [98] under Wiley Author’s (Liu’s) Rights of Reuse, License Number 4193800778386.
In QCSE, the absorption spectrum redshifts under the applied electric field due to the redshift of the discrete exciton peaks and the discrete sub-bands of the QWs. A feature of QCSE is that the peak absorption coefficient decreases as the applied field increases, arising from the less overlap of electron and hole wavefunctions and the tendency of exciton dissociation under the electric field. The figure-of-merit (FOM) for EA materials and devices is the absorption contrast, Δα/α, i.e., relative change in absorption coefficient when an electric field is applied. A rigorous analysis of the FKE can be obtained by solving the Schr?dinger’s equation for an electron-hole pair in the presence of a one-dimensional electric field[96]. For quantitative modeling of QCSE, the readers are referred to Chapter 13 of Refs. [96, 97]. A review on Ge and GeSi FKE and QCSE modulators can be found in Ref. [98]. In this section, we will focus on the key points of Ge/GeSi EAM design principles and their integration with datacom systems.
In designing Ge and GeSi EAMs, it should be noted that the contribution of the indirect gap absorption to the overall absorption coefficients α is not negligible, although its contribution to Δα is negligible[99, 100]. This is different from the case of direct gap semiconductors. The background of the indirect gap absorption leads to low FKE absorption contrast of Δα/α ~ 1 in bulk Ge. The first study on 0.2% tensile-strained Ge showed that the decreased energy difference between direct and indirect gaps induced by tensile strain can enhance the absorption contrast to 3 at λ = 1647 nm for F = 70 kV/cm[101], which agrees very well with the theoretical model (Fig. 10(a) and 10(b)). Based on the same model, Δα/α can exceed 4 at F = 85 kV/cm, and 5 at F = 105 kV/cm before Ge breakdown (see the inset of Fig. 10(b)). It is noteworthy that the real part of the refractive index also changes notably under an applied field (denoted by “Δn”) due to the Kramer-Kronig relation. The EO coefficient, Δn/F, is as high as 280 pm/V for F < 50 kV/cm at λ = 1647 nm, compared to 240 pm/V for InP and 164 pm/V for LiNbO3. To blueshift the optimal operation wavelength to 1550 nm, the most technically important wavelength in optical communications, tensile strained Ge can be alloyed with a small amount of Si to increase the direct band gap of FKE modulators[102]. The addition of Si tends to decrease Δα/α since it increases the difference between the direct and indirect gaps (Figs. 10(c) and 10(d)). Nevertheless, a Δα/α of ~3 can still be achieved at λ ~ 1550 nm for Ge1–x Six[102] under 100 kV/cm electric field.

class="figure_img" id="Figure10"/>
Download
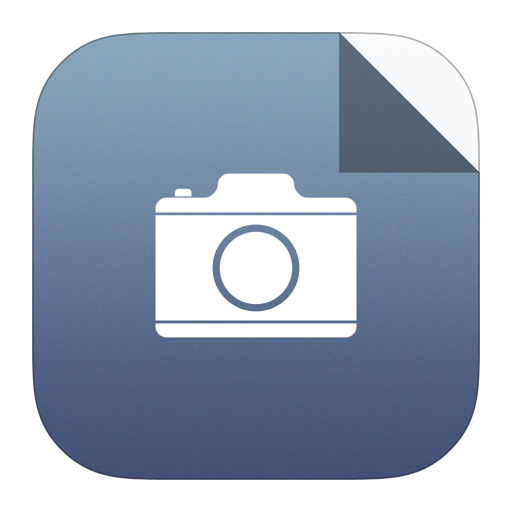
Larger image
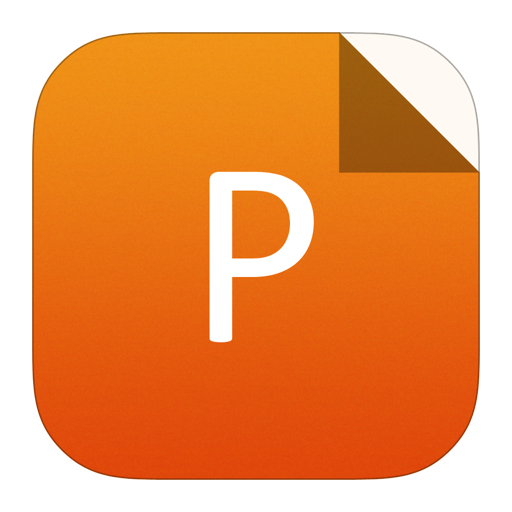
PowerPoint slide
Figure10.
(Color online) (a) Experimental and theoretical absorption spectra of 0.2% tensile strained Ge-on-Si under applied electric fields of 14 and 70 kV/cm. (b) Experimental and theoretical absorption contrast spectra (corresponding to (a)) for 0.2% tensile strained Ge-on-Si. The inset shows the calculated refractive index change Δn and absorption contrast Δα/α at λ = 1647 nm as a function of applied electric field. The inset is reproduced from Ref. [101] with permission from AIP Publishing LLC ?2006. (c) Absorption contrast, Δα/α, as a function of wavelength for GeSi alloys with different Si compositions. The GeSi is 0.26% uniaxially strained along the <110> direction. (d) Δα/α at λ = 1550 nm as a function of Si composition. In (c) and (d) we assumed an applied electric field of 100 kV/cm and a built-in field of 10 kV/cm at 0 V in the intrinsic GeSi region. Panels (a–c) are reproduced from Ref. [98] under Wiley Authors’ (Liu’s) Rights of Reuse, License Number 4193800778386. Panel (d) is reproduced from Ref. [102] under the Author’s (Liu’s) Copyright Transfer Agreement with OSA.
To further achieve QCSE in Ge QWs, it is critical to understand the band alignment in SiGe/Ge system. Earlier work from Reiger and Vogl has laid the foundation for Type I SiGe/Ge QW design, as shown in Fig. 11[103]. According to the definition labeled in the figures, a negative band offset in the conduction band and a positive offset in the valence band indicates Type-I alignment for the indirect gap of GeSi. One can find that Type I alignment for the indirect gap can be achieved for Ge-rich GexSi1–x with 0.75 ≤ x ≤ 1 grown on a barrier layer of 0.6 ≤ y ≤ 1. Based on these band alignment charts, Kuo et al. reported the first observation of strong QCSE in 10 nm Ge/16 nm Ge0.85Si0.15 QW structure, where Type I alignment for both the direct and indirect conduction valleys are obtained[104]. Note that the band offset from the direct Γ valley and the indirect L valleys should be evaluated separately. The band-offset of Si0.15Ge0.85 barrier in the direct Γ valley is as large as 0.4 eV[104], providing strong confinement for the demonstration of QCSE in Ge QWs. Clear exciton absorption peaks and redshift in absorption spectra were observed as the reserve bias increased from 0 to ?4 V, similar to the behavior of direct gap AlGaAs/GaAs QWs. The maximum absorption contrast Δα/α is ~4.7 at 1461 nm under an electric field of ~105 kV/cm (?4 V reverse bias). Several approaches were then adopted to redshift the operation wavelength to λ ~ 1550 nm for SiGe/Ge QCSE modulators. One could heat up the device (e.g. 100 °C) to reduce the band gap[105], apply a larger electric field to extend the QCSE spectral regime[106], or utilize some strain engineering approaches to convert the compressive strain in the Ge QWs into tensile strain. Tensile strain engineering is the most favorable method since it avoids additional power consumption induced by heating or increased reserve bias. It also helps to reduce the energy difference between the direct and indirect gaps in Ge QWs, thereby increasing Δα/α. By increasing the Si content in the SiGe barrier, the operation wavelength of Ge QCSE can also be blue-shifted to ~1300 nm[107], covering another important wavelength in optical communications especially for datacom applications.

class="figure_img" id="Figure11"/>
Download
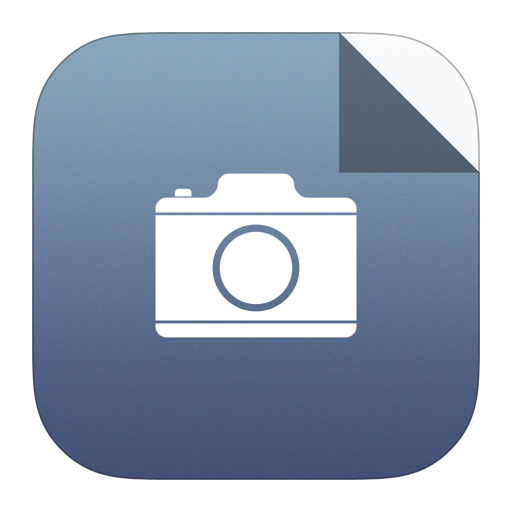
Larger image
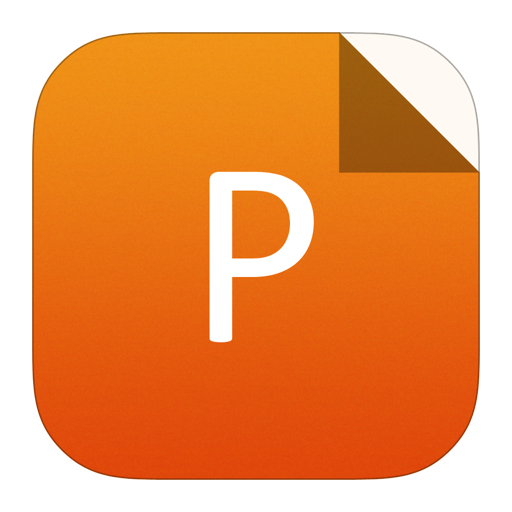
PowerPoint slide
Figure11.
(a) Conduction and (b) valence band offsets of pseudomorphic (lattice matched) Si1–xGex grown on <001> Si 1–yGey. Note that the conduction band offset is based upon the indirect conduction valley with the minimal energy. Reproduced from Ref. [103] with the permission from the American Physical Society.
It is interesting to compare the absorption contrast (Δα/α) and operation wavelengths of FKE versus QCSE in Ge. Overall, the Δα/α of Ge QWs is similar to that of FKE in tensile strained Ge under the same applied electric field of ~100 kV/cm, although the optimal working wavelengths are different (1461 nm versus 1647 nm). QCSE in Ge indeed shows a much larger Δα compared to FKE at the same electric field of ~100 kV/cm, i.e. Δα ~ 2500 cm?1 at 1461 nm[104] versus ~300 cm?1 at 1647 nm[101, 102]. However, two factors work against high Δα/α in Ge QCSE. (1) The quantum confinement effect itself increases the energy difference between the direct and indirect gaps of the Ge QWs, making the Ge QW more of an indirect gap semiconductor. This is because electrons in the direct Γ valley see a much deeper well compared to those in the indirect L valleys, and their effective mass is much smaller than that in L valleys (0.038 m0 versus 0.2 m0, where m0 is the mass of an electron). (2) The compressive strain in SiGe/Ge QWs due to the lattice mismatch between Ge and Si0.15Ge0.85 also increases the energy difference between the direct and indirect gaps. Consequently, the background absorption loss from the indirect gap transition of Ge QWs is also significantly higher than that of 0.2% tensile strained bulk Ge (500 cm?1 versus 60 cm?1). Overall, considering that the absorption contrasts are comparable while a single tensile-strained Ge epitaxial layer is much easier to grow than multiple Ge QWs, for integration with CMOS process it is more convenient to use tensile-strain enhanced FKE for waveguide-coupled Ge and GeSi EAMs. Moreover, the optical confinement in Ge and GeSi FKE modulators is typically larger than that in the Ge QWs. On the other hand, for applications at 1310 nm wavelength Ge QCSE is a better candidate since the quantum confinement effect helps to blueshift the band gap for operation at such short wavelengths.
(B) Ge and GeSi EAM device performance
For integration into Si photonic circuits, Ge and GeSi EAMs are coupled with waveguides via butt coupling or evanescent coupling. Interestingly, the same Ge/GeSi material and device structure can be applied to waveguide-coupled photodetectors, too[102, 108], which greatly facilitates high throughput monolithic integration. The first waveguide-integrated GeSi EAM was reported by Liu et al. in 2008[108] using tensile strained enhanced FKE in Ge0.992Si0.008 for optimal performance at 1550 nm, while the first waveguide-integrated Ge QCSE EAM working at 1450–1470 nm was reported by Ren et al. in 2012[119]. Table 3 summarizes the performance of Ge and GeSi EAMs reported in recent years. Butt coupling is widely used in Ge and GeSi FKE EAMs for better coupling efficiency, while low-loss taper-enhanced evanescent coupling are also applied occasionally. For FKE EAMs, extinction ratio of 3–8 dB and >30 GHz bandwidth can be obtained routinely nowadays. Recently Gupta et al.[114] and Srinivasan et al.[115] achieved a taper-enhanced, evanescently coupled Ge EAM with a bandwidth/data rate of 50 GHz/56 Gbps, low dynamic energy consumption of 12.8 fJ/bit and 30 nm operation wavelength range at a peak-to-peak voltage swing of Vpp = 2 V. These features are very close to the metrics required for modulators in datacom.
For QCSE EAMs, so far most of the devices are not directly integrated with waveguides due to the complication in fabrication. Nonetheless, waveguide-coupled Ge QCSE modulator indeed achieved a very compact footprint of 0.8 × 10 μm2 and an ultralow power consumption of ~1 fJ/bit utilizing the large Δα in QCSE[119]. In principle, the operation wavelength can be shifted to 1550 nm at a high reverse bias of ?7.5 V judging from the photocurrent measurement of the device, although direct modulation was not presented. Furthermore, the operation wavelength can be shifted to ~1300 nm using SiGe barrier layers with higher Si composition to enhance the quantum confinement effect. This covers another important wavelength range in datacom and telecom.
Overall, waveguide-integrated Ge and GeSi EAMs have achieved a 3 dB bandwidth greater than 50 GHz, a low energy consumption ~10 fJ/bit, and a reasonably broad (15–30 nm) operation wavelength range for multi-channel WDM applications. They can very well cover the S, C, and L bands in optical communications. The 3 dB bandwidth is only limited by RC delay since the field-induced EAE itself occurs in sub-ps time frame[120]. Due to their simplicity in fabrication and device integration, Ge and GeSi EAMs based on FKE have entered system level applications, as will be discussed in more detail in the next section. Ge EAMs based on QCSE have demonstrated great potential to achieve ultralow energy operation on the order of 1 fJ/bit in both 1310 and 1550 nm communication wavelength windows. The results in Table 3 indicate that Ge and GeSi EAMs are ideal candidates for high bandwidth, high energy-efficiency photonic modulators monolithically integrated on Si.
Modulator type | Optimal wave-length range (nm) | Coupling method | ER (dB) | IL (dB) | Band-width (GHz) | Vpp (V) | Active device area (μm2) | Average dynamic energy per bit $frac{1}{4}$ CVpp2 (fJ/bit) |
Ge and GeSi FKE modulators | ||||||||
2008[108] Liu et al. | 1539–1553 | Butt | 8 | 3.7 | 1.2 | 3 (?4 to ?7 V) | 0.6×50 | 25a |
2011[109] Lim et al. | 1580–1610 | Evanescent | 10@ 1600 nm | 9.6 | 1.25 Gb/s | 5 | 0.8×20 | 102–103 |
2011[110] Feng et al. | 1610–1640 | Butt | 6.3@ 1620 nm | 3.6@ 1620 nm | 30 | 4 | 1×45 | ~70 |
2012[111] Feng et al. | 1545–1581 | Butt | 6 | 5 | 40.7 | 2.8 | 1×55 | 60 |
2013[112] Feng et al.l | 1525–1560 | Butt | 3.8 | 4.8 | 34 | 2 | 0.8×50 | 70 |
2014 Krishna-moorthy et al.[113] | 1525–1555 | Butt | 3 | 3 | >40; >10 Gb/s for 9 channels | 2 | – | 50 for EAM; 570 including driverb |
2015[114] Gupta et al. | 1600–1630 | Evanescent (with taper) | 4.6 | 4.1 | 50 | 2 | – | 10 |
2016[115] Srinivasan et al. | 1600–1630 | Evanescent (with taper) | 4.8 | 4.6 | 56 Gbps | 2 | 0.6×40 | 12.8 |
Ge QCSE modulators | ||||||||
2007 Roth et al.[116] | 1441–1461 | Side-entry | 7.3@ 1457 nm | ~9@ 1457 nm | – | 10 | 450×450 | – |
2008 Roth et al[105] | 1535–1545c | Side-entry | ~6@ 1540 nm | – | – | 4 | 225×625 | – |
2011 Chaisakul et al.[117] | 1415–1440 | Fiber coupled | 7/10@ 1420 nm | 3/7 dB@ 1420 nm | – | 6 | L = 34 or 64 μm | – |
2012 Chaisakul et al.[118] | 1425–1446 | Fiber coupled | 9@ 1435 nm Vpp=1 V | 15@ 1435 nm, (?3 V); | 23 | 1 (?3 to ?4 V) | 3×90 | 108 |
2012 Ren et al. [119] | 1450–1470 @?4 V DC bias d | Butt | >3.2@ 1460 nm | ~15 | 3.5 | 1 (?3 to ?5 V) | 0.8×10 | 0.75 |
2014 Rouifed et al.[107] | 1290–1310 | Fiber coupled | 6@ 1293 nm | 2.5 | – | 7 | 3×150 | – |
a In Liu et al. 2008 a conservative estimate of 50 fJ/bit was reported based on $frac{1}{2}$ CVpp2, considering the most power-consuming scenario of flipping between “on” and “off” states in every operation. b Integrated with 40 nm technology node CMOS driver and wavelength multiplexer. c Operating at 100 °C to reduce the direct band gap of the Ge QWs. d The operation wavelength range could be redshifted up to 1550 nm at a DC bias of ?7.5 and 1 V voltage swing judging from the photocurrent measurement. The direct modulation data is only available at 1460 nm with ?4 V DC bias, though. |
Table3.
Summary of Ge and GeSi EAM performance, adapted from Refs. [9, 98].
Table options
-->
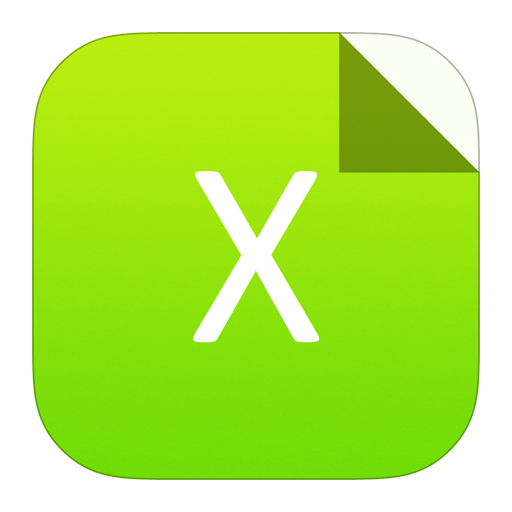
Download as CSV
Modulator type | Optimal wave-length range (nm) | Coupling method | ER (dB) | IL (dB) | Band-width (GHz) | Vpp (V) | Active device area (μm2) | Average dynamic energy per bit $frac{1}{4}$ CVpp2 (fJ/bit) |
Ge and GeSi FKE modulators | ||||||||
2008[108] Liu et al. | 1539–1553 | Butt | 8 | 3.7 | 1.2 | 3 (?4 to ?7 V) | 0.6×50 | 25a |
2011[109] Lim et al. | 1580–1610 | Evanescent | 10@ 1600 nm | 9.6 | 1.25 Gb/s | 5 | 0.8×20 | 102–103 |
2011[110] Feng et al. | 1610–1640 | Butt | 6.3@ 1620 nm | 3.6@ 1620 nm | 30 | 4 | 1×45 | ~70 |
2012[111] Feng et al. | 1545–1581 | Butt | 6 | 5 | 40.7 | 2.8 | 1×55 | 60 |
2013[112] Feng et al.l | 1525–1560 | Butt | 3.8 | 4.8 | 34 | 2 | 0.8×50 | 70 |
2014 Krishna-moorthy et al.[113] | 1525–1555 | Butt | 3 | 3 | >40; >10 Gb/s for 9 channels | 2 | – | 50 for EAM; 570 including driverb |
2015[114] Gupta et al. | 1600–1630 | Evanescent (with taper) | 4.6 | 4.1 | 50 | 2 | – | 10 |
2016[115] Srinivasan et al. | 1600–1630 | Evanescent (with taper) | 4.8 | 4.6 | 56 Gbps | 2 | 0.6×40 | 12.8 |
Ge QCSE modulators | ||||||||
2007 Roth et al.[116] | 1441–1461 | Side-entry | 7.3@ 1457 nm | ~9@ 1457 nm | – | 10 | 450×450 | – |
2008 Roth et al[105] | 1535–1545c | Side-entry | ~6@ 1540 nm | – | – | 4 | 225×625 | – |
2011 Chaisakul et al.[117] | 1415–1440 | Fiber coupled | 7/10@ 1420 nm | 3/7 dB@ 1420 nm | – | 6 | L = 34 or 64 μm | – |
2012 Chaisakul et al.[118] | 1425–1446 | Fiber coupled | 9@ 1435 nm Vpp=1 V | 15@ 1435 nm, (?3 V); | 23 | 1 (?3 to ?4 V) | 3×90 | 108 |
2012 Ren et al. [119] | 1450–1470 @?4 V DC bias d | Butt | >3.2@ 1460 nm | ~15 | 3.5 | 1 (?3 to ?5 V) | 0.8×10 | 0.75 |
2014 Rouifed et al.[107] | 1290–1310 | Fiber coupled | 6@ 1293 nm | 2.5 | – | 7 | 3×150 | – |
a In Liu et al. 2008 a conservative estimate of 50 fJ/bit was reported based on $frac{1}{2}$ CVpp2, considering the most power-consuming scenario of flipping between “on” and “off” states in every operation. b Integrated with 40 nm technology node CMOS driver and wavelength multiplexer. c Operating at 100 °C to reduce the direct band gap of the Ge QWs. d The operation wavelength range could be redshifted up to 1550 nm at a DC bias of ?7.5 and 1 V voltage swing judging from the photocurrent measurement. The direct modulation data is only available at 1460 nm with ?4 V DC bias, though. |
(C) Ge and GeSi EAMs for photonic datalink system integration
Based on the maturity of Ge and GeSi FKE EAMs, photonic datalink systems utilizing these modulators have been demonstrated in recent years. Krishnamoorthy et al. reported the first multi-channel GeSi EAM array integrated with 40 nm technology node digital CMOS driver and wavelength multiplexer, marking the entry of waveguide-coupled GeSi EAMs into system level integration[113]. Individual devices demonstrate a high bandwidth >40 GHz. Fig. 12(a) shows the transmission spectra of an unpackaged 10-channel device spaced at 100 GHz with an isolation >20 dB. Single channel operation up to 25 Gb/s and nine-channel operation at 10.25 Gb/s have been achieved at a low Vpp of 2 V (Fig. 12(b)). The energy consumption of the EAM itself is as low as 50 fJ/bit, and the total energy is 570 fJ/bit including the modulator driver. Clearly, electrical rather than optical components are the major limiting factor of energy efficiency.

class="figure_img" id="Figure12"/>
Download
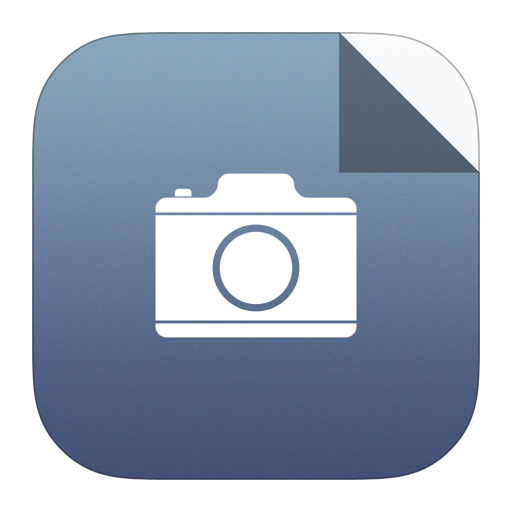
Larger image
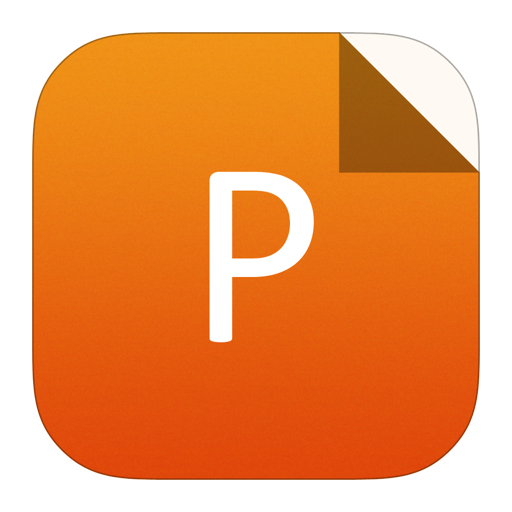
PowerPoint slide
Figure12.
(Color online) (a) Transmission spectra of an unpackaged 10-channel GeSi FKE modulator + multiplexer array chip reported by Krishnamoorthy et al. (Ref. [113]). (b) Eye diagrams at 10.25 Gb/s for a packaged 9-channel device chip. Center wavelength is 1532 nm and separation between channels is 1.6 nm. Reproduced from Ref. [113] with permission from OSA.
In 2015–2017, system integration of Ge/GeSi EAM has gained more momentum driven by the energy efficiency requirement in datacom. De Heyn et al.[121] demonstrated a 16 × 56 Gbps GeSi EAM-PD array coupled to multicore fibers to achieve 896 Gbps for short reach (1–10 m) optical links. The GeSi EAMs are 40 μm long to obtain a good balance between insertion loss and extinction ratio, while the GeSi PDs are 60 μm long to absorb more light for photocurrent generation. The monolithic integration of GeSi EAMs and PDs using the same material and device structure demonstrates the design principle proposed in Ref. [102]. Verbist et al.[122] have demonstrated the first 100 Gbps non return to zero (NRZ) on-off key (OOK) transmission using GeSi EAM for over 500 m distance through standard single mode optical fibers, and 2 km using dispersion shifted fiber. The system is integrated on a 200 mm SOI platform. All this success indicates that GeSi EAMs are well on the way for system integration in ultralow energy, high speed photonic datalinks.
3.2.2
Graphene modulators
Driven by intense interests in its unique optical modulation mechanism, graphene optical modulators have been demonstrated based on EA, EO, all-optical, thermo-optic, and magneto-optic mechanisms[123]. In this review, for the purpose of Si photonics application, we focus only on the graphene EAMs.
(A) Mechanism and fundamental tradeoff of graphene EAMs
Graphene, a single layer of hexagonally packed carbon atoms, is a promising material for application in optical modulators because of its extraordinary electrical, optical and thermal properties. It features a conical band structure (see Fig. 13(a)[124]), where the energy of the electron is proportional to its momentum, in sharp contrast to the (approximately) parabolic band structure of regular semiconductor materials. This special band structure leads to a different EA mechanism compared to conventional semiconductor EAMs. Instead of tilting or shifting the band edge by applying an electric field (recall Section 3.2.1), the EAE is achieved by accumulating or depleting electrons from the graphene layer via capacitive gating to modify its Fermi level (EF), and correspondingly, the band filling. The modification of optical transitions in graphene by changing its Fermi level through capacitive gating was first demonstrated in Ref. [125]. Using the Dirac points (where the conduction and valance bands meet) as a reference, for Fermi level
m{F}}}{
m{ }} gg {k_{
m{B}}}T$

$${E_{ m F}} = pm (hbar {v_{ m F}})sqrt {pi {n_{ m {e, ,,h}}}},,, , $$ ![]() | (1) |
where the positive/negative sign applies to the case of electron/hole carriers, respectively, and the Femi velocity vF is approximately 108 cm/s for pristine graphene. Upon capacitive gating under an applied voltage of ΔV, the areal charged carrier density is changed by
$$Delta {n_{ m e,,, h}} = CDelta V/Aq, $$ ![]() | (2) |
where C is the total capacitance of the gating device (e.g. a MOS device), A is the area of the active graphene region, and q = 1.6 × 10?19 C is the charge of an electron. Therefore, by charging/discharging the capacitive device, the Fermi level of graphene can be modified according to Eqs. (1) and (2).
When the Fermi level is near the Dirac point (i.e. nearly intrinsic graphene), the unique conical band structure gives a constant absorption of 2.3% for vertical incidence independent of wavelength[126]. This transfers to a very large absorption coefficient of 105–106 cm?1 in the entire optical communication wavelength regime, ~50× greater than that of GaAs. Therefore, graphene is opaque to photons at optical communication wavelengths when integrated with waveguides for long enough optical absorption path length in the plane of graphene (see Fig. 13(a), middle panel). When the Fermi level is increased above hv/2 due to electron accumulation or decreased below –hv/2 due to electron depletion in the graphene layer via capacitive gating, the material becomes transparent to photons with energy < hv. In the former case, all the quantum states that allow optical excitation by photons with energy < hv are fully occupied, therefore there is no optical absorption (Fig. 13(a), right panel). In the latter case, all the quantum states that allow optical excitation by photons with energy < hv are empty (Fig. 13(a), left panel), therefore there is no optical absorption, either. This way, the graphene material is turned from opaque to transparent for EAM applications.
Due to this unique EA mechanism, graphene EAMs shows the following features/advantages compared to conventional semiconductor EAMs: (1) Ultra broad, tunable operation spectral range: Unlike EAMs based on conventional semiconductors that can only work within ~30 nm wide spectral range near the band edge (as mentioned in Section 3.2.1), the Fermi level of graphene can be tuned to the order of 0.1 eV to achieve EAE in a broad spectral regime. The operation wavelength can be easily tuned by changing the gating voltage. (2) High modulation speed: Graphene has a carrier mobility as high as 200 000 cm2/(V·s) at room temperature, far exceeding most of the conventional semiconductors[127]. Therefore, the modulation speed is only limited by RC bandwidth. (3) Compatibility with CMOS back-end-of-line processing[128]. With the rapid progress of graphene layer transfer technology, it is becoming possible to integrate large-area graphene on Si wafers at low thermal budget and low-cost. There is no thermal mismatch, passivation or epitaxy issue as III–V hybrid integration since graphene is attached via Van der Waals forces and there are no dangling bonds on its surface. With these benefits, it is appealing that graphene could achieve broad spectrum, high speed, and low power modulation for integrated photonic circuits.
Despite of these great advantages, one needs to be aware of a fundamental trade-off between the RC-limited bandwidth fRC and the driving voltage/power consumption of graphene EAMs. This is because high bandwidth prefers lower capacitance C, so higher driving voltage ΔV is needed to achieve the same amount of change in the charged carrier density (Δne, h) and the corresponding Fermi level shift, according to Eqs. (1) and (2). For a given Δne, h, in order to achieve a certain extinction ratio and modulation spectral range based on the shift in the Fermi level, the dynamic power consumption of the photonic datalink in terms of energy/bit is given by:
$$(1/4)CDelta {V^2} = (1/4)C{(qADelta {n_{ m e, h}}/C)^2} = {(qADelta {n_{ m e, h}})^2}/(4C), $$ ![]() | (3) |
which is inversely proportional to C. Note that C is often not directly proportional to A since there is also parasitic capacitance outside the graphene area. Therefore, to increase the RC bandwidth of graphene EAMs by reducing C, the dynamic power consumption will also increase proportionally. We can defined energy per bit per unit 3 dB bandwidth to capture this trade-off:
$$begin{split}({ m Energy/bit})/3 { m dB};{ m bandwidth} = & frac{{{{(qADelta {n_{ m e,,, h}})}^2}}}{{4C}} ,,, 2pi RC =& frac{{pi R{{(qADelta {n_{ m e,,, h}})}^2}}}{2}, end{split}$$ ![]() | (4) |
where R is the resistance of the device (including load resistance and contact resistance). Clearly, reducing the Δne, h needed for optical transparency is an effective way to minimize this tradeoff. It also implies that intrinsic graphene EAMs suffers less power efficiency-bandwidth tradeoff when working at longer wavelengths (i.e. lower photon energy, thus lower |ΔEF| and Δne, h for modulation). Reducing the active graphene area is also effective, yet it is limited by the waveguide width to sustain the optical mode and the length to sample enough EAE for the targeted modulation depth.
Another challenge of graphene modulator is the very limited optical confinement in the atomically thin graphene layer. This means a longer optical path is needed. Efforts have been made to optimize the overlap between the graphene layer and the optical mode in the waveguide, as will be discussed shortly.
(B) Graphene EAM devices
In 2011, Liu et al.[124] demonstrated the first graphene EAM by placing a monolayer graphene sheet on top of a Si waveguide with a thin Al2O3 separation layer (Fig. 13(b)). The waveguide is designed so as to maximize the field at the interface between the waveguide and the graphene for optimal absorption and EAE efficiency in graphene. TM mode is utilized for this purpose. External gating voltage was utilized to control the Fermi level of graphene, resulting in changes of transmission in the waveguide structure (Fig. 13(a)). The transmission spectrum as a function of driving voltage is divided into three regions. In the middle region (?1 V < Vd < 3.8 V), the Fermi level ( EF, black dashed line) is close to the Dirac point and the interband transition is allowed from occupied states (red lines) to unoccupied states (green lines). This is the optical “off” state of the EAM. In the other two regions, the optical absorption is forbidden either due to electron depletion (Vd < ?1 V, left panel) or accumulation ( Vd > 3.8 V, right panel), as mentioned in the previous section. These are the optical “on” state of the modulator. A modulation depth per unit length of 0.1 dB/ μm was achieved. With a device footprint of 0.6 × 40 μm2, a 3 dB frequency of 1.2 GHz and a modulation depth of 4 dB was demonstrated at a driving voltage of ?3.5 V (depletion mode), together with a very broad operation spectrum from 1350 to 1600 nm. Such a broad operation spectral range is not available in conventional EAMs.
An issue noted in Fig. 13(a) is that there is hardly any EA modulation at low voltages between ?1 V and 3.8 V (middle panel), thereby requiring higher voltage to get considerable modulation depth. Later, the same team improved the modulation depth per unit length to 0.16 dB/μm with a modified double-layer graphene on Si waveguide structure[129] (Fig. 14). The two graphene layers, with an oxide layer in between to form a p-graphene (depletion)/oxide/n-graphene (accumulation) parallel plate capacitor structure, simultaneously achieve opaqueness or transparency for the incident light under modulation voltage swing. The overlap between graphene and the optical mode is also increased. As expected, the EAE is notably enhanced in the low voltage regime (region II) in Fig. 14 compared to that in Fig. 13. The modulation speed is 1 GHz at 2 V for the double layer graphene modulator, limited by the high series resistance due to the poor contact between metal and graphene.

class="figure_img" id="Figure13"/>
Download
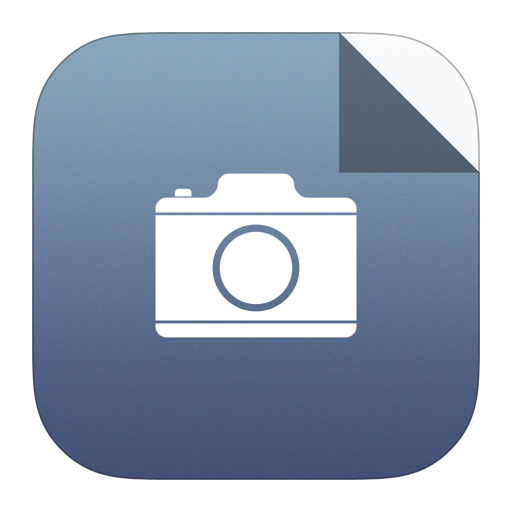
Larger image
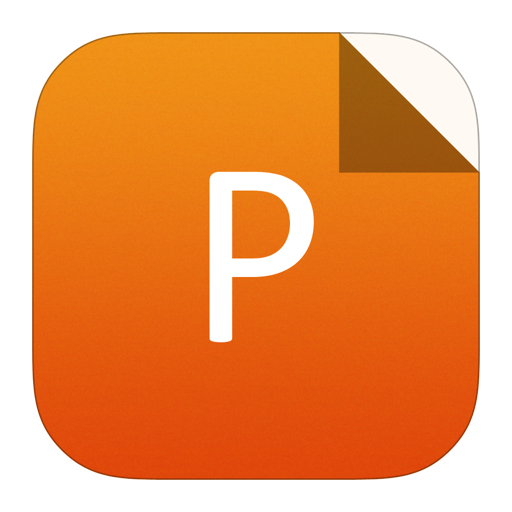
PowerPoint slide
Figure13.
(Color online) (a) Schematics of the band structures/band filling of graphene and the corresponding optical transmission at 1530 nm versus applied voltage through the EAM device reported in Ref. [124]. In the band diagrams, red parts refer to occupied states, while green parts refer to unoccupied sates. Left panel: under electron depletion (large negative voltage); Middle panel: under low bias (nearly intrinsic); Right panel: under electron accumulation (large positive bias). (b) 3D schematics of the graphene EAM device. (c) Cross-section of the graphene EAM device with an overlay of the optical mode (TM mode) calculated by finite element simulation. The waveguide was designed so as to maximize the field at the interface between the waveguide and the graphene for optimal EA efficiency. Reproduced from Ref. [124] with permission from the Nature Publishing Group.

class="figure_img" id="Figure14"/>
Download
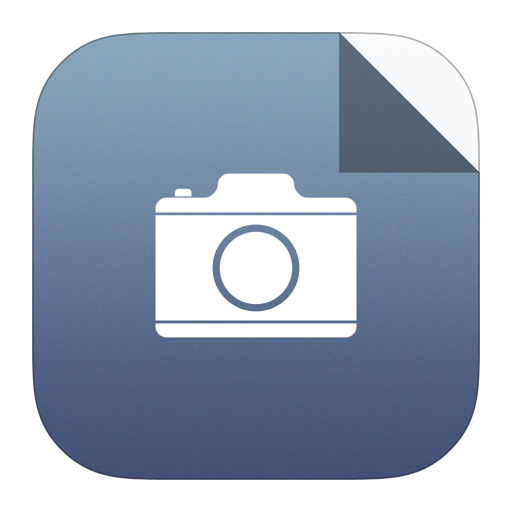
Larger image
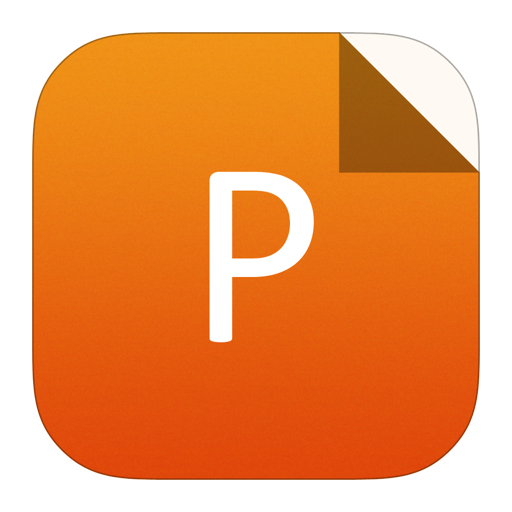
PowerPoint slide
Figure14.
(Color online) (a) Static response of a double layer graphene modulator with Al2O3 spacer in between. Modulation depth of ~6.5 dB can be achieved using a 40 μm long device (0.16 dB/μm) at 1537 nm wavelength. (b) The graphene band structure/band filling profiles (green for unoccupied state, red for occupied states) for regions I, II, and III in (a). The arrows represent the incident photons. The inset is a schematic illustration of the double layer graphene modulator. Reprinted from Ref. [129] with permission from ACS Publishing.
Mohsin et al. placed the double layer graphene on the standard 220 × 520 nm single mode Si waveguide in 2014[130]. This graphene based EAM exhibited 3.3 dB insertion loss and 16 dB extinction ratio (Δα/α = 4.8) at 1550 nm operation wavelength. The extinction ratio has not only surpassed Ge and GeSi EAMs, but also become comparable to the silicon MZI modulators. However, a hysteretic behavior is observed in the static measurement of the optical transmission as a function of the applied voltage, mainly due to charge traps generated by adsorbates such as O2/H2O redox couples at the graphene/dielectric boundary[131]. The modulation speed is only 670 MHz with a driving voltage swing of Vpp = 6 V, mainly because of the large device capacitance (2.3 pF).
More advanced structures are proposed and demonstrated for improving the performance of graphene EAMs in different aspects. Graphene microring EAMs have been demonstrated in recent years[132, 133]. The graphene layer is transferred onto the top of Si or silicon nitride microring resonators to implement the graphene/Al2O3/Graphene structures mentioned earlier. The EAE in graphene changes the critical coupling conditions of the microrings, thereby achieving optical modulation of the light transported through the bus waveguide. Ref. [133] demonstrated a high modulation efficiency of 1.5 dB/V between ?40 and ?50 V bias and a high bandwidth ~30 GHz, indicating the great potential of graphene EAMs. The drawback of high driving voltage is due to the small capacitance (55 fF) needed for high bandwidth, as discussed in the fundamental tradeoff between bandwidth and driving voltage in Part A of this section. In this case, a large |Δ EF| ~ 0.4 eV is needed for operation at ~1550 nm wavelength with 0.8 eV photon energy. This determines a fairly large Δne, h ~ 1.2 × 1013 cm?2 for graphene EAMs to operate at 1550 nm according to Eqs. (1) and (2). To help the readers appreciate its magnitude, it is equivalent to a change in carrier density of 1.2 × 1013 cm?2/(0.335 nm) = 3.6 × 1020 cm?3 in bulk materials considering 0.335 nm thickness of single layer graphene, or two orders larger than the case of Si modulators. Therefore, one has to either use a larger capacitance for low voltage operation yet low bandwidth as shown in Ref. [124], or high bandwidth but high driving voltage as shown in Ref. [133]. A possible method to alleviate this tradeoff is to chemically dope the two graphene layers n-type and p-type[134], respectively, such that the corresponding Fermi levels are much closer to 0.4 and ?0.4 eV in reference to the Dirac points, respectively. This way, a much smaller amount of charge accumulation/depletion (Δne, h) is needed to achieve transparency at 1550 nm, thereby greatly reducing the driving voltage.
In addition to graphene EAMs mentioned above, EOMs based on the refractive index change of graphene have also been investigated[135–137]. The change in the effective refractive index can be as large as 0.028[135] compared to 10?4 – 10?3 in conventional Si MZI modulators, which enables modulation with shorter device (< 30μm long) and less power consumption. However, due to the complex fabrication process involved, such as embedding graphene into dielectric waveguides for maximal modal overlap, most of the work is still at the stage of simulation and optimization.
Overall, the emerging compact graphene modulators offer a unique solution for broad spectral range, high bandwidth modulators for integrated photonics. The very broad and tunable operation spectral range is a unique advantage of graphene modulators compared to conventional modulators. In addition to photonic datalinks, they are well suited for modulation in the MIR (λ = 3–8 μm) regime for sensing and free space communication applications. As mentioned in Part A in this section, operation at longer wavelength is actually more energy-efficient for intrinsic graphene EAMs since less Fermi level shift is needed for transparency. The development of graphene fabrication technique towards large area device fabrication with chemical doping capability and high reliability will be the key factor for their future applications.
3.2.3
Plasmonic-organic modulators
The miniaturization of integrated photonic circuits below the diffraction limit in plasmonics research offers Si photonics an unprecedented opportunity for high-speed manipulation of information with low power consumption. Plasmonic Si modulators have been investigated theoretically and experimentally for some time by several research teams[138–140]. However, ultrahigh speed modulation beyond 50 GHz hasn’t been demonstrated in this type of plasmonic Si modulators yet.
Complementing the plasmonic slot waveguides with highly nonlinear organic material (e.g. nonlinear chromophores) offers ultracompact, high-speed modulators[141], even though plasmonic waveguides have a fundamental trade-off between their strong mode confinement and the short propagation length[142]. Plasmonic-organic modulator can exhibit an effective refractive index change of ~0.1, several orders of magnitude larger than typical Si MZI modulators based on FCPD effect. The main contributors to this ultrahigh index change are (1) the large EO coefficient of the nonlinear chromophore material and correspondingly, the large relative change in its refractive index,
m material}}/{n_{
m material}}$

m slow}}$

$$Delta {n_{ m eff}} = varGamma frac{{Delta {n_{ m material}}}}{{{n_{ m material}}}}{n_{ m slow}}. $$ ![]() | (5) |
Another advantage of the electro-optic effect of the organic nonlinear material is that the optical absorption of the material at the working wavelength is less affected by the refractive index change during phase modulation, compared to Si FCPD modulators.
Following the first demonstration of the plasmonic-organic hybrid modulator in 2014[141], a fast, compact, all-plasmonic MZI modulator was reported in 2015 based on highly nonlinear chromophores (DLD-164)[143], comprising splitters integrated with photonic-to-plasmonic converters and plasmonic nonlinear modulator sections (Fig. 15(a)). The working principle is as follows: light guided in the input single mode silicon waveguide is coupled to a symmetric plasmonic mode by tapering down the silicon waveguide. The symmetric plasmonic mode excites two in-phase SPP modes in the upper and lower metal-insulator-metal (MIM) slot waveguides, respectively. The voltage between the center metal island and the outer ground electrodes is used to shift the relative phase of the two SPP modes. Only in the on-state, the SPPs in two arms are in phase and light is guided out along the output silicon waveguide.

class="figure_img" id="Figure15"/>
Download
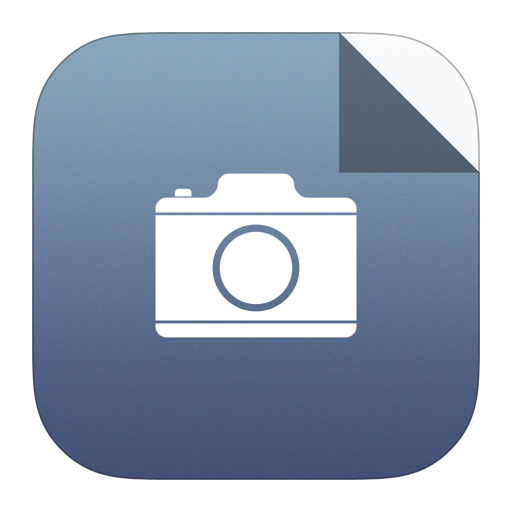
Larger image
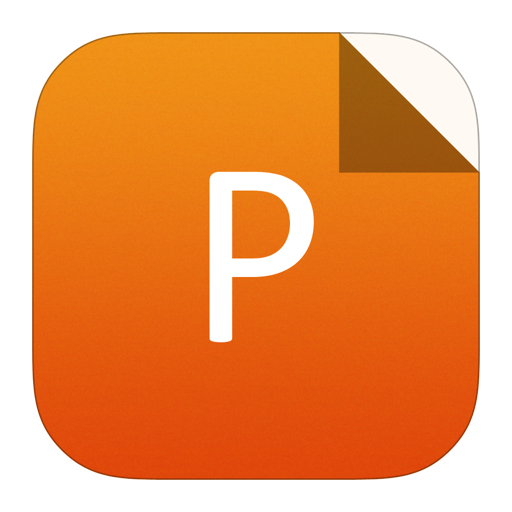
PowerPoint slide
Figure15.
(Color online) An all-plasmonic MZI modulator. (a) Colorized SEM image of the modulator. The plasmonic interferometer is formed by the metallic island and the metallic contact pads. An electrical signal can be applied to the island via a suspended bridge. (b) Finite element simulations showing the plasmonic modes in the off-state and the on-state. In the off-state, the fields are out of phase at the output, while they are in phase in the on-state. Light is coupled to guided modes of the silicon waveguide only when the modulator is in the on-state. Figures reprinted from Ref. [143] with copyright permission from Nature Publishing Group.
Right after the first demonstration of the principle, the plasmonic-organic modulators witness rapid progress. Recently, the best plasmonic organic MZI modulator has reached a 3 dB bandwidth of 110 GHz, a low energy consumption of 25 fJ/bit, a half-wave voltage-length product of VπL = 40 V·μm, an extinction ratio of 25 dB, and an insertion loss of 5 dB[142]. The footprint and VπL are reduced by two orders of magnitude compared with Si MZI modulators. Advanced modulation formats such as QPSK and 16-QAM are exploited in the plasmonic-organic hybrid modulators to further increase the data rate on a single channel, enabling 144 Gb/s photonic link[142]. The key challenge ahead is to introduce these nonlinear organic materials into CMOS compatible process flow and set up a reliable procedure for high-yield products.
3.3
Photodetectors
3.3.1
GeSn infrared detectors
Ge/Si PDs and APDs have achieved great progress in the past decade, with the performance competitive to that of III–V detectors. Waveguide-coupled Ge/Si PDs are successfully integrated into Si photonic circuits to convert optical signal to electrical signal, as discussed in Section 2. They have also entered semiconductor foundries with a CMOS compatible processing flow in a low cost, high volume, high yield manner. Some high speed, normal incidence Ge/Si APDs have been applied to commercial receiver optical sub-assembly (ROSA)[144, 145].
However, a disadvantage of free-space Ge/Si PDs is that the responsivity is relatively low for the wavelengths larger than 1550 nm, or photon energy below its direct bandgap. While tensile strain is used to shrink the direct bandgap of Ge to cover the L-band in optical communication[26], it is hard for strain engineering to further extend the response to MIR regime for sensing and free-space communication applications. A straight-forward extension to longer wavelengths in IR and MIR range is to exploit small bandgap GeSn materials on Si, as discussed in Section 3.1.2. Several groups are devoted to GeSn p–i–n photodiodes with small Sn content up to 4%[146–150], while only a few teams studied direct gap GeSn detectors with Sn content of ~10% to target >2 μm photon detection[151–153]. The reason lies in the fact that it is more difficult to obtain good quality GeSn thin films with high Sn concentration and low dislocation density.
Table 4 summarizes the recent progress in Ge1-xSnx photodetectors. So far the GeSn photodetectors can hardly compete with their tensile-strained Ge counterparts at wavelengths ≤ 1600 nm due to much higher dark current (1–10 A/cm2 versus 1–10 mA/cm2) and relatively little improvement in responsivity. It is more attractive that the incorporation of Sn has extended the cutoff length to >2 μm[151–153]. In terms of bandwidth, GeSn photodetectors operating at 1550 nm with a high speed of 40 GHz have also been demonstrated[150], yet no high-speed capability has been achieved at λ ≥ 2 μm yet. For room-temperature application, a key hurdle is the much higher dark current density of GeSn PDs compared to that of Ge PDs, which greatly affects the detectivity. While this is partially due to the bandgap shrinkage, the 2 orders higher dark current density for low Sn composition GeSn PDs[146–150] compared to pure Ge indicates that material defects is the major problem. The lowest dark current density of 31 mA/cm2 at a reverse bias of ?1 V was reported in the Ge0.9Sn0.1 multiple-quantum-wells (MQW) detectors[153], reaching a similar level to that of Ge photodetectors. The Ge0.9Sn0.1 MQW was fully strained to the underlying Ge buffer layer without forming thread dislocations at the GeSn/Ge interfaces, thereby resulting in the low dark current density. A notable challenge in reducing the defect density in GeSn is the thermal budget. The post-annealing method widely used in epitaxial Ge technology is not suitable for GeSn due to the tendency of Sn segregation upon annealing. This is a concern not only for threading dislocation reduction, but also point defect reduction. Overall, GeSn photodetectors are still in the early stage of development, demanding greater efforts in material growth and device fabrication for improved performance. On the other hand, other simple technologies are emerging to compete with GeSn in the detection of 2 μm wavelength light, as will be discussed in the next section.
Year | Structure | xSn (%) | Dark current (A/cm2) at ?1 V | Responsivity (A/W) | Speed |
2011 Werner[146] | p–i–n | 0.5 (MBE) | 10 | 0.102 @ 0 V, 1550 nm 0.018 @ 0 V, 1640 nm | – |
2011 Roucka[147] | p–i–n | 2.0 (UHCVD) | ~10 | 0.113 @ 0 V, 1550 nm 0.086 @ 0 V, 1640 nm | – |
2011 Su[148] | p–i–n | 3 (MBE) | 2.36 | 0.23 @ ?1 V, 1540 nm 0.12 @ ?1 V, 1640 nm | – |
2012 Oehme[149] | p–i–n | 4 (MBE) | 100 | 0.181 @ ?0.1 V, 1550 nm 0.171 @ ?0.1 V, 1600 nm | – |
2014 Oehme[150] | p–i–n | 4.2 (MBE) | 0.9 | 0.218 @ ?1 V, 1550 nm | 40 GHz @?5 V, 1550 nm |
2016 Pham[151] | p–i–n | 6.4 (CVD) 9.2 (CVD) | 5.74 18 | 0.3 @ 0 V, 1550 nm 0.19 @ 0 V, 1550 nm Cutoff 2.6 μm | |
2012 Gassenq[152] | MSM | Ge0.91Sn0.09/Ge quantum well, (CVD) | – | 0.1 @ ?5 V, 2.2 μm | 2 MHz |
2017 Dong[153] | p–i–n | Ge0.9Sn0.1/Ge MQW (CVD) | 0.031 | 0.216 @ ?1 V, 1530 nm 0.023 @? 1 V, 2 μm | 1.2 GHz |
2014 Peng[154] | waveguide coupled p–i–n | 1.28 (CVD) | 6.75 | 0.27 @ 0 V, 1550 nm 0.124 @ 0 V, 1600 nm | – |
2005 Liu[26] | p–i–n | 0 (UHCVD) 0.2% tensile strained Ge | 0.01 | 0.56 @ 0 V, 1550 nm 0.11 @ 0 V, 1605 nm | 8.5 GHz @ ?1 V |
Table4.
Summary of the recent demonstration of GeSn detectors compared to free-space Ge p–i–n photodiodes in Ref. [26].
Table options
-->
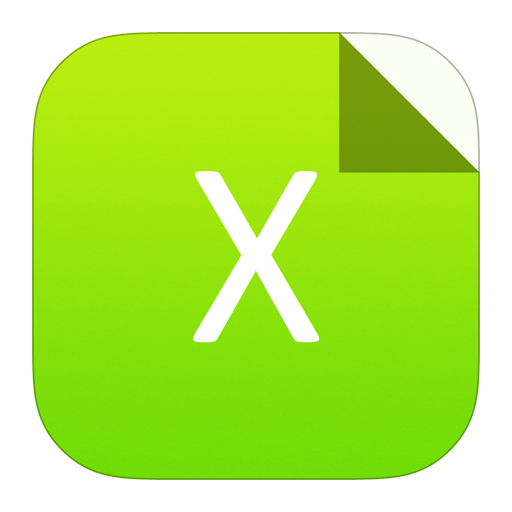
Download as CSV
Year | Structure | xSn (%) | Dark current (A/cm2) at ?1 V | Responsivity (A/W) | Speed |
2011 Werner[146] | p–i–n | 0.5 (MBE) | 10 | 0.102 @ 0 V, 1550 nm 0.018 @ 0 V, 1640 nm | – |
2011 Roucka[147] | p–i–n | 2.0 (UHCVD) | ~10 | 0.113 @ 0 V, 1550 nm 0.086 @ 0 V, 1640 nm | – |
2011 Su[148] | p–i–n | 3 (MBE) | 2.36 | 0.23 @ ?1 V, 1540 nm 0.12 @ ?1 V, 1640 nm | – |
2012 Oehme[149] | p–i–n | 4 (MBE) | 100 | 0.181 @ ?0.1 V, 1550 nm 0.171 @ ?0.1 V, 1600 nm | – |
2014 Oehme[150] | p–i–n | 4.2 (MBE) | 0.9 | 0.218 @ ?1 V, 1550 nm | 40 GHz @?5 V, 1550 nm |
2016 Pham[151] | p–i–n | 6.4 (CVD) 9.2 (CVD) | 5.74 18 | 0.3 @ 0 V, 1550 nm 0.19 @ 0 V, 1550 nm Cutoff 2.6 μm | |
2012 Gassenq[152] | MSM | Ge0.91Sn0.09/Ge quantum well, (CVD) | – | 0.1 @ ?5 V, 2.2 μm | 2 MHz |
2017 Dong[153] | p–i–n | Ge0.9Sn0.1/Ge MQW (CVD) | 0.031 | 0.216 @ ?1 V, 1530 nm 0.023 @? 1 V, 2 μm | 1.2 GHz |
2014 Peng[154] | waveguide coupled p–i–n | 1.28 (CVD) | 6.75 | 0.27 @ 0 V, 1550 nm 0.124 @ 0 V, 1600 nm | – |
2005 Liu[26] | p–i–n | 0 (UHCVD) 0.2% tensile strained Ge | 0.01 | 0.56 @ 0 V, 1550 nm 0.11 @ 0 V, 1605 nm | 8.5 GHz @ ?1 V |
3.3.2
Plasmonic Schottky infrared detectors
Conventional Si-based photodetectors utilize the direct bandgap absorption of other semiconductor material system (III–V, Ge, GeSn) integrated on Si. Some are well established in the telecommunication wavelength range (e.g. Ge PDs), while others are under development with potential applications in the MIR regime (e.g. GeSn PDs). In recent years tremendous advances are made in the field of all-silicon sub-bandgap photodetectors due to their compactness and simplicity requiring no additional tools beyond standard CMOS fabrication. These sub-bandgap photodetectors can also find the applications in monitoring the optical power in Si photonic circuits. The working principles of these devices include defect-mediated absorption, TPA, and internal photoemission (IPE)[155]. By making the best of the emerging materials and novel structures, the compact all-silicon PDs are paving a new way for photodetection on Si platform beyond heteroepitaxy and wafer bonding. Of these new approaches, defect-mediated Si photodetectors have limited absorption and requires high driving voltage for avalanche gain[25]. Photodetectors based on TPA mechanism is not suitable for Si photonic circuits due to the requirement of high optical power density[155].
The IPE effect in the Schottky diodes proved to be a more promising alternative. A simple structure comprises a metal film on doped semiconductor (e.g. Si) forming a Schottky contact at the metal/semiconductor interface with a barrier height of Φb (Fig. 16(a)). Upon excitation by photons with hv > Φb, electrons in the metal gain enough energy to overcome the Schottky barrier and reach the semiconductor side, thereby accelerated under the electric field of the depleted region in the semiconductor and collected as photocurrent. Typically Φb is less than the bandgap of Si (1.1 eV), which makes Schottky diode configuration a good candidate for all-Si sub-band photodetection[155]. The internal quantum efficiency of IPE is given by the product of two factors: one is the fraction of the excited carriers having appropriate momentum and energy to overcome the potential barrier Φb, and the other is the transmission probability of these optically excited hot carriers over the Schottky barrier after being scattered by cold carriers and the metal boundary surfaces. To enhance the IPE efficiency, we could concentrate the incident optical field near the metal/semiconductor interface either by localized plasma with nanoscale metallic structures (nano antenna) or by SPP propagating along the metal/Si interface[156]. The latter offers longer interaction length between the propagating SPP and the photodetector, thus allowing larger fraction of the hot electrons to be generated nearby the Schottky barrier. It can also be easily integrated with waveguides.

class="figure_img" id="Figure16"/>
Download
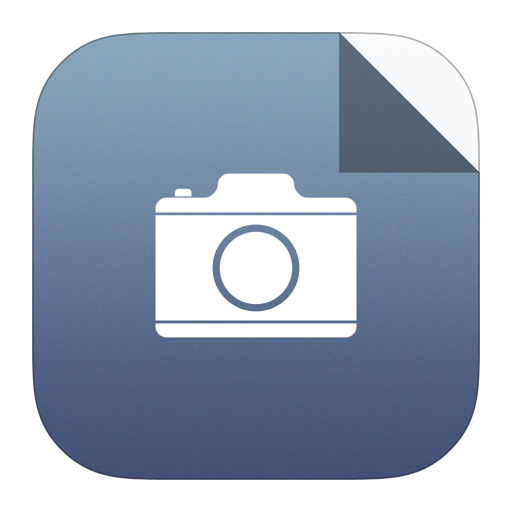
Larger image
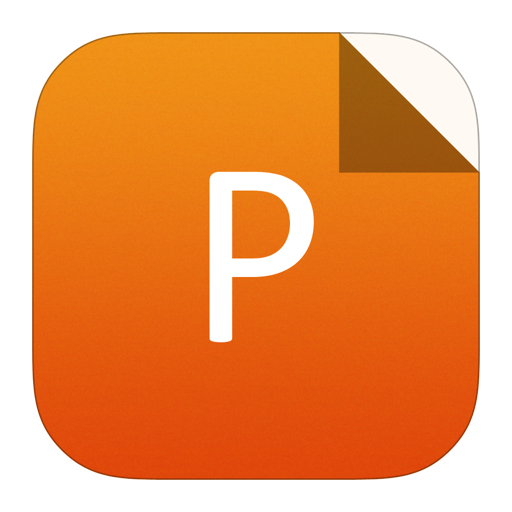
PowerPoint slide
Figure16.
(Color online) (a) Schematic band diagram and working principle of metal/semiconductor Schottky IR photodetectors (original figure). (b) Simulated cross-secitonal optical intensity profile of a SPP waveguide mode supported by a metal?graphene?Si Schottky photodetector structure. (c) SEM micrograph of the waveguide coupled metal-graphene-silicon Schottky photodetector. (b) and (c) are reprinted from Ref. [162] with permission from ACS publication.
Several Si plasmonic IR Schottky photodetectors have been demonstrated, using both localized plasma[157, 158] and SPP[159, 160]. Though the responsivity of plasmonic Schottky diodes is improved by two orders of magnitude compared to plain metal/Si Schottky diode, it does not exceed few tens mA/W with maximum IQE of 1% due to the requirement of momentum matching in the IPE effect[161]. A remarkable recent discovery is that 2D material such as graphene can greatly boost the responsivity of waveguide-coupled plasmonic Schottky photodetectors to 85 mA/W at 1550 nm under ?1 V reverse bias, corresponding to 7% internal quantum efficiency. At a larger reverse bias of ?3 V, the responsivity reaches 0.37 A/W due to avalanche gain in Si[162]. The optical mode profile of a metal–graphene–Si waveguide structure is shown in Fig. 16(b), where the optical field is confined close to the metal/Si interface. Fig. 16(c) shows the top-view SEM image of a waveguide-coupled metal–graphene–Si Scottky diode. Later, the reason for the strongly enhanced IPE due to the graphene layer is tentatively explained. It has been suggested that the field enhancement at the metal/graphene/Si interface (Fig. 16(b)) increases the optical absorption in graphene layer itself. In addition, the relatively long hot carrier relaxation time in graphene may allow multiple attempts for the excited carrier to overcome the Schottky barrier and transport into the semiconductor[163]. Interface disorder, such as nanoscale roughness at the interface, is also crucial to overcome the momentum mismatch in the internal photoemission process[163]. However, there have been no reports on the 3 dB bandwidth so far. Overall, the development of plasmonic Schottky photodetector is still at an early stage, which is in great need of fundamental studies on hot carrier transport in thin metals and through the Schottky barrier, as well as avalanche multiplication in semiconductors induced by hot carriers.
3.3.3
Si-based non-avalanche SPDs
In the Si photonics community, most of the attention has been devoted to optical communications and interconnects in recent years. Relatively few have noticed the breakthrough in Si photonics in ultrahigh sensitivity imaging, photon counting, and single photon detection. In this section, we will discuss a recent breakthrough in Si quanta image sensors (QIS) that achieves non-avalanche SPDs at room temperature with a record low dark count of 0.1 e?/s and read noise < 0.2 e ?. These devices greatly benefit from the CMOS scaling and may inspire new ways of envisioning/optimizing electronic-photonic integration on Si. Instead of using avalanche gain for SPD, QIS devices utilize the simple principle that an appreciable voltage change (ΔV) can be measured if a photoelectron (e?) is transferred to a small capacitor (C) according to ΔV = e?/C. The small capacitance is naturally enabled by CMOS scaling, a perfect example of electronic-photonic synergy.
Digital image sensors and cameras based on Si CMOS technology has become part of our everyday life since early 2000 s. Practically every cell phone is equipped with a camera based on backside-illuminated Si CMOS image sensor these days. However, even 5 years ago probably few in the optical communication and quantum optics community would imagine implementing SPDs using these devices. On the other hand, as early as 2005 Si QIS has been envisioned by Dr. Eric Fossum, a major inventor of Si CMOS image sensors. He asked what we could do with the sub-diffraction limit (SDL) pixels enabled by the progress in CMOS technology nodes following Moore’s Law. He envisioned two major impacts of these SDL pixels that he later termed “jots”: (1) They enable ultrahigh sensitivity photon detection down to single photon level. This is because the photovoltage conversion gain for a single photoelectron, ΔV = e?/C, can reach the order of mV when the pixel capacitance C scales down to hundreds of aF level with the advance of CMOS technology nodes[164]. The mV-level signal generated by a single (absorbed) photon can be read out using low-noise CMOS circuity, thereby achieving non-avalanche SPD. The long standing issue of avalanche noise is naturally avoided. (2) They enable a large oversampling of the image, where SDL pixels can be later regrouped for various image processing to retrieve more information. In recent years, these visions were experimentally implemented using the industrial CMOS image sensor foundry at Taiwan Semiconductor Manufacturing Company (TSMC).
The first photon counting jot was demonstrated by Ma and Fossum in 2015. Fig. 17(a) shows the cross-sectional doping profile of the jot device structure[165, 166]. Photoelectrons are generated and collected in the n-type storage well (SW) under the transfer gate (TG) due to the designed doping and potential profile (Figs. 17(a) and 17(b)). Note that there is a potential barrier (PB) between SW and TG so that the stored photoelectrons are far enough from the oxide/Si interface at the TG region to avoid carrier recombination and dark counts induced by interfacial defects. Upon reading, the TG is turned on (Fig. 17(c)) so that the photoelectron is transferred to the potential well (PW) region right below the TG. Fig. 17(e) further shows the corresponding potential profile along the AA’ dashed line in Fig. 17(a), indicating that the potential barrier is removed when the TG is turned on, while the PW region has the lowest potential to accommodate the transferred photoelectrons. Subsequently, the TG is turned off so that the photoelectron is transferred horizontally to the heavily n-doped floating diffusion (FD) node. Thanks to the 65 nm CMOS technology node and the tapered pump gate (TPG) structure at the reset gate (RG) to minimize the overlap capacitance, an ultralow capacitance (C ~ 400 aF) is achieved for the FD region such that a photovoltage conversion gain of ΔV = e?/C~0.4 mV was achieved per photoelectron[164–166]. This conversion gain is well above the noise level of ~0.1 mV in 65 nm CMOS circuits and enables high-sensitivity SPDs.

class="figure_img" id="Figure17"/>
Download
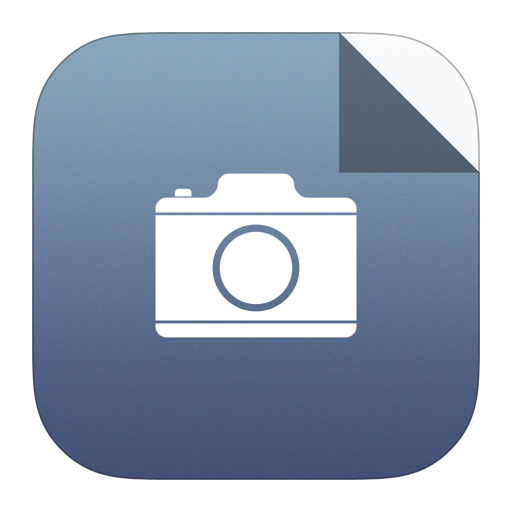
Larger image
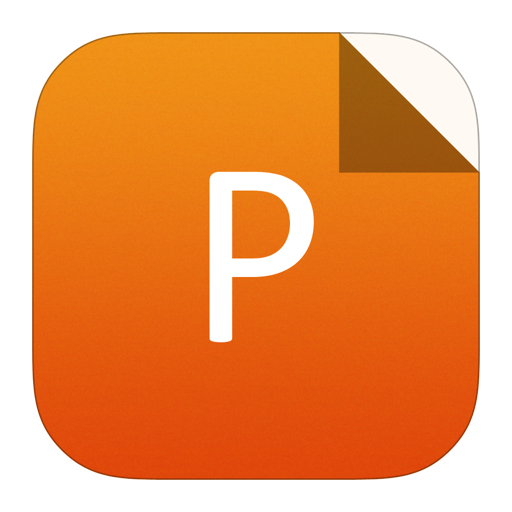
PowerPoint slide
Figure17.
(Color online) (a) Cross-sectional structure and doping profile of the Si QIS photon counting detector. Due to proprietary reasons, detailed doping concentration is not provided. Panels (b)-(d) show the potential profile of the device: (b) photon absorption and photoelectron generation in the SW region; (c) photoelectron transfer to the PW region when the TG is turned on; (d) further photoelectron transfer to the FD region for photovoltage readout. Reprinted with the Author’s permission from Ref. [166].
The photon counter performance of the Si QIS is shown in the photon counting histogram (PCH) of Fig. 18(a). In the ideal case, the PCH would show vertical lines (Delta functions) corresponding to the events of 0, 1, 2, …, N photoelectrons being generated in the jot. In reality one would see Gaussian peaks instead of Delta peaks at integer photoelectron numbers due to the read noise. The overlap of the peaks is associated with the bit error rate (BER) upon photon counting since one cannot distinguish N from N + 1 photoelectrons in these overlapped regions. Fig. 18(a) clearly show distinct peaks corresponding to the events of different number of photons absorbed by the jot, which demonstrates photon counting ability at room temperature. Compared to single photon avalanche detectors (SPADs), the dark counts is reduced by 3 orders of magnitude (0.1 e?/s[164–167] versus ~100 e?/s[168]) at room temperature since the avalanche noise is avoided in QIS devices. The read noise reaches 0.22 e? rms. in the optimal device (“golden” jot) with an average of 0.29 e?.

class="figure_img" id="Figure18"/>
Download
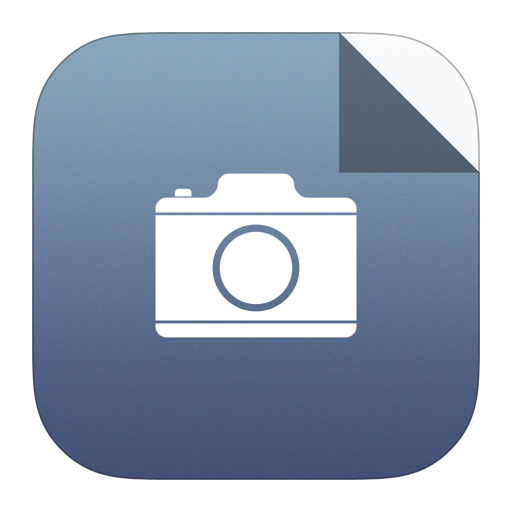
Larger image
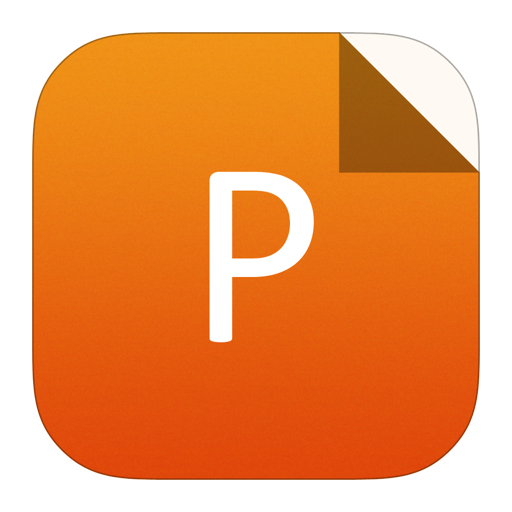
PowerPoint slide
Figure18.
(Color online) Photon counting histograms of (a) a “golden” jot of the first generation of Si QIS; (b) a jot in the second generation using punch-through reset to further decrease the capacitance and increase the photovoltage gain. Reprinted with the Author’s permission from Ref. [166].
Very recently, a second generation of Si QIS has been demonstrated in megapixel array[166, 167]. The Si QIS layer was fabricated using 45 nm CMOS technology at TSMC, integrated with 65 nm CMOS readout circuit. As shown in Fig. 18(b), the PCH shows very clearly defined Gaussian peaks that are much less overlapped compared to the first generation devices in Fig. 18(a). This is due to a lower read noise o 0.172 e? for the best jots in the second generation, corresponding to a BER of ~0.0015 for single photon detection. The average read noise of TPG jots is also decreased from 0.29 to 0.22 e?, i.e. reaching the level of the best jots in the first generation due to the advance in CMOS technology node as well as improved doping profile design. This represents 5× improvement in the average BER from 0.04 to 0.008 for single photon detection. Furthermore, the new punch-through reset jots eliminate the parasitic capacitance of the reset transistors, thereby further increasing the photovoltage gain and decreasing the read noise.
The rapid progress of Si QIS for room temperature photon counting and SPDs clearly demonstrates the importance of electronic–photonic synergy. It means that our cell phone cameras can reach single photon counting sensitivity in the near future, a performance that has been associated with expensive and specialized scientific tools even a few years ago. We obtained two important inspirations from photon counting Si QIS for future electronic–photonic integrations: (1) Utilize the Si material to its maximal advantage. While Si itself is not a perfect optical absorber, 50 years of development of CMOS technology achieved unparalleled material quality. For example, the 0.1 e?/s dark count rate and the long photoelectron lifetime in the SW region is enabled by the nearly perfect Si material. If the same QIS device structure were moved to another material platform, it may not work nearly as well due to the defects in those materials. (2) Utilize the CMOS fabrication to its maximal advantage. The photovoltage conversion gain in QIS is enabled by very small capacitance of devices in <65 nm CMOS nodes, opening a new path other than APDs for single photon detection.
In the past, electronic-photonic integration has been mostly utilizing Si CMOS as a platform to accommodate photonic devices into the Si processing flow. In most cases there is still a big margin to achieve real electronic-photonic synergy by maximizing the advantage of Si and CMOS processing flow. While the low noise avalanche gain of Si has been utilized in Ge/Si APDs, the scaling of CMOS technology has not been used to its maximal advantage so far. We can ask the question: how about extending the photon counting capability of Si QIS to telecommunication wavelength regime by integrating an absorber at 1310/1550 nm with Si QIS structures? How much can we further reduce the energy/bit in photonic datalinks using such capability? Are there other aspects that we could benefit from CMOS scaling for a higher level of electronic-photonic synergy? We believe that addressing these questions may well lead to new device structures and integration schemes in future Si integrated photonic circuits, far exceeding the performance of photonic integration on any other platform.
4.
Conclusions
In conclusion, we have presented a comprehensive review of recent efforts and progress in emerging Si active photonic device technologies, including lasers, modulators and photodetectors, beyond the conventional ones that have already entered industrial foundries. For emerging laser sources, high-performance InAs quantum dot lasers on Si is promising for data center applications in the near future. Monolithic band-engineered Ge-on-Si lasers have made remarkable progress in the past few years, where improving material quality and device structure (e.g. tunneling injection to the direct conduction valley) is the key towards future applications. GeSn lasers show great potential for monolithic lasers in sensing and free-space communication applications, yet fundamental understanding needs to be deepened for this new system. Both Ge and GeSn lasers need to find a path towards QW and QD structures to be more competitive in laser performance. In terms of modulators, GeSi EAMs, graphene EAMs, and plasmonic-organic EOMs all show great promise for high speed and ultralow power modulation. GeSi EAMs are entering system level integration for data communications in the near future. Graphene EAMs show a unique broad spectral operation capability well beyond conventional EAMs, yet material and processing integration as well as device structure needs further development and optimization. It is likely that the optical confinement in the active graphene layer can be further improved using plasmonic waveguide structures. Plasmonic-organic EOMs offer the best MZI modulator performance on Si so far, and process integration and reliability will be the key aspects to address for practical applications. For photodetectors, GeSn detectors are on the way towards MIR application, pending further improvement in material quality to reduce the dark current. All-Si plasmonic Schottky detectors offer a facile solution beyond epitaxy and wafer bonding, yet fundamental studies are needed to further improve the internal photo emission efficiency. Finally, Si quanta image sensors already achieved non-avalanche, low noise single photon detection and photon counting at room-temperature, offering inspiration for new thoughts to optimize the synergy between Si photonics and CMOS electronics. These emerging technologies, though still under development, could make a significant impact on the future of large-scale electronic-photonic integration with performance inaccessible from conventional Si photonic technologies.