1.
Introduction
Recent advances in ultrathin electronic devices have broadened the scope of flexible/stretchable electronics from flexible circuits, foldable displays and flexible energy harvesting/storage devices to the advanced stretchable and wearable biomedical devices that can integrate with the complex curved geometries to closely monitor and record the various vital bio-signals[1–6]. The poor mechanical properties of conventional inorganic electronic materials greatly hinder their integration in flexible/stretchable applications, which motivated researchers to make efforts in developing alternative materials, with excellent mechanical endurance against bending/stretching, that can be coupled with shape-conforming systems maintaining the functional properties and electronic performance parameters unaffected with body movements.
In the efforts to investigate materials for developing efficient flexible/wearable devices, graphene has emerged as a most promising material in the last decade. Its excellent mechanical properties with huge tensile strength ~130 GPa, Young’s modulus of ~0.5–1 TPa, spring constant ~1–5 N/m, and stretching ability (elasticity)[7–10] make it highly suitable material for flexible and stretchable/wearable devices. In addition, the other outstanding material properties of graphene, such as high chemical and thermal stability, large specific 2-D surface area for conformal adhesion of the other materials/substrates, wide optical absorption spectrum (300–1400 nm), excellent transparency of ~97% in the visible wavelength range, piezo and thermo-resistive response, and electrical sensitivity towards biochemicals, make it a most promising multifunctional material for flexible devices. However, the electronic properties of graphene have been realized as unique features till now over other available electronic materials such as its ultra-high electronic mobility (15000–200000 cm2/(V·s)) due to the ballistic transport of carriers, ambipolar behavior, and high conductivity comparable to conventionally available indium tin oxide. Fig. 1(a) shows the variation of resistivity in monolayer graphene with the application of bias voltages which indicates its ambipolar behavior with the charge neutrality point at 0 V[11]. The variation in resistivity (left) and mobility (right) with respect to applied voltage and carrier concentration, respectively, is shown in Fig. 1(b) for suspended graphene[12]. The blue and red curve shows the characteristics before and after current annealing the device. The current annealing of the graphene monolayer eliminated the residual impurities, thus, was found to improve the transport properties. Further, the monolayer graphene-based field effect transistors were demonstrated. Fig. 1(c) shows the variation of conductance values with sweeping of top gate bias at different back gate voltages for monolayer graphene. The wafer scale graphene was produced using c-face silicon carbide (SiC) wafer and an array of graphene transistors were fabricated as shown in Fig. 1(d)[13]. The zoomed part of the individual transistor is shown below Fig. 1(d). The corresponding high-frequency measurement for the 240 nm gate length transistor is shown in Fig. 1(e). The current gain was estimated to be 1 at 100 GHz cut-off frequency. Such exciting electrical features of graphene at an early stage opened a wide scope towards graphene-based electronics. In addition to these beneficial electronic properties, its low sheet resistance and high transmittance, combinedly makes it a potential candidate to replace conventional transparent conducting electrodes (TCE) materials like indium tin oxide and Al-doped ZnO (AZO) in flexible optoelectronic applications[14, 15]. Therefore, graphene has been enormously explored as TCE in various flexible applications such as touch-panels, light-emitting diodes (LEDs), photovoltaic cells, sensors, and energy harvesting devices, etc.

class="figure_img" id="Figure1"/>
Download
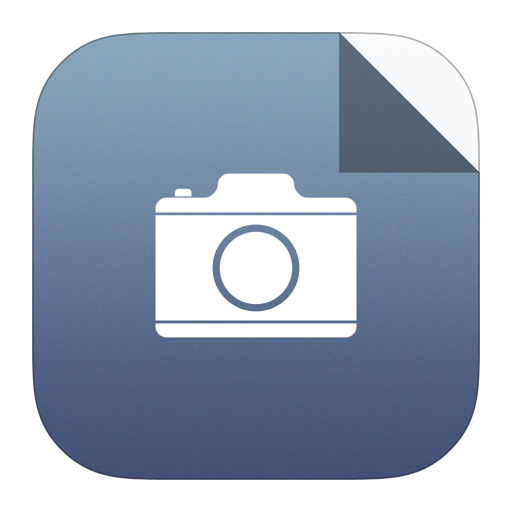
Larger image
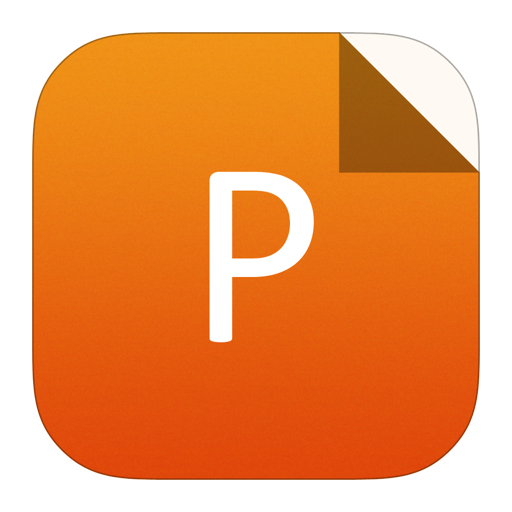
PowerPoint slide
Figure1.
(Color online) (a) The tuning of charge carrier from electrons to holes under opposite bias indicating the ambipolar electric field effect in monolayer graphene. Insets show the conical low-energy spectrum, representing the changes in Fermi energy position with applied gate voltage[11]. (b) Measured resistivity and mobility of suspended graphene device as a function of gate voltage before (blue) and after (red) current annealing. Gray dotted line shows the data of the device fabricated on the substrate[12]. (c) The conductance of a single layer graphene-based top-gate FET as a function of the bias voltage under different back-gate voltages. The inset shows the schematic structure of the fabricated device. (d) An array of transistors fabricated on graphene wafer produced by thermal desorption of Si from the c-face of a SiC wafer. The inset shows the enlarged view of an individual transistor. (e) High-frequency performance of a 240 nm gate length graphene transistor[13].
Since the discovery of graphene by mechanical exfoliation in 2004[16], several efforts have been made towards its growth and synthesis, such as epitaxial growth on SiC and metal surfaces[17–19], reduction from graphite oxide[20], liquid-phase exfoliation[21] and chemical vapor deposition (CVD)[22]. Among these, CVD has been considered to be the most promising and feasible method for large-scale production of graphene films[23, 24] and provided an opportunity for the realization in the practical application of flexible/wearable devices. The presence of multiple defects (pinholes, microcracks and overlapped grain boundaries) that originated during CVD synthesis leads to the degradation of its outstanding physical properties[25], which is a critical concern. Hence, the optimization of growth processing for the production of high quality, large-area, defect-free graphene is still a challenge that needs to be addressed for the implementation of graphene-based efficient flexible and wearable devices.
In this article, we review the recent progress made in graphene-based flexible and wearable devices for various applications. First, we summarize the main synthesis approaches for large area production along with its transfer for desired device architecture towards flexible and stretchable platforms. Thereafter, we highlight several applications of graphene in flexible and wearable devices including high-speed field effect transistors (FETs), touch panels/displays, LEDs, photovoltaic cells, biomedical sensors, and energy-harvesting devices. Finally, the article is concluded with discussing the present technological challenges/issues involved and future possibilities in developing graphene-based practical, wearable devices for commercialization prospects.
2.
Synthesis and transfer process: compatible with large area device fabrication
As pristine graphene produced by mechanical exfoliation is limited by its tiny size and difficulty to reproduce, enormous effort has been attempted to establish the high quality, cost-effective scalable synthesis of graphene[11, 26]. The effective development production of large area, high-quality graphene films is needed for future flexible device applications[27, 28]. A layer-controlled simple solution-based chemical exfoliation has proven to be an effective method for producing a large quantity of graphene but the electrical and optical property of the graphene exhibits an inferior quality due to the chemical disorder[29, 30]. In contrast, chemical vapor deposition (CVD) is an expensive method which requires a small amount of carbon gases without any material resources also capable of producing a large area high-quality graphene layer that makes it suitable for large area flexible device application. Here, in this section, we will mainly present the recent progress of the synthesis and transfer method of graphene using CVD methods for flexible and wearable applications.
2.1
Scalable synthesis method: chemical vapor deposition
Large area high-quality synthesis of graphene can be achieved by chemical vapor deposition on metal (Ni or Cu) substrate using a gaseous carbon source[31–33]. There are two general mechanisms of graphene growth on metal by CVD namely, (1) precipitation and (2) surface-mediated reaction. In both cases, the growth process simply occurs in three steps. First, the hydrocarbon gas precursor is inserted at a certain growth temperature into the chamber where the thin metal film is placed. In the next step, a carbon molecule decomposes on the metal surface and diffuses into the substrate. Finally, a graphene layer grows during the cooling process. The growth kinetics of graphene on the Ni and Cu surface is illustrated schematically in Fig. 2(a)[23, 24]. Due to the lower solubility of carbon in Cu than that of Ni substrate, the dissolving and decompose rate of carbon is lower on the Cu substrate that facilitates surface migration leading to monolayer graphene growth on the Cu surface. In contrast, due to the high solubility of carbon, a large amount of carbon atoms can be dissolved, and bulk diffusion of carbon atoms occurs in Ni. As a result, a cooling process of segregation occurs via precipitation that mostly results in the growth of poly-graphene. Lee et al.[34] demonstrated a large 3 inch wafer-scale growth of high-quality graphene film on Ni and Cu film coated on Si/SiO2 substrate in ambient pressure. Experimentally, 300 nm Ni and 700 nm Cu film was used as a metal catalyst. Three to eight layers of graphene grew on a Ni catalyst depending on the reaction time and cooling rates, whereas, the mono-and bilayer graphene grows predominantly on a Cu catalyst. Fig. 2(b) shows an image of the as-grown graphene film on the 3-inch SiO2/Si substrate. A transfer method was developed to etch the metal layer instantly by using soft poly (dimethylsiloxane) (PDMS) stamps and thermal-release tapes (Nitto Denko Co.). Fig. 2(c) shows the Raman spectra of graphene layers grown on Cu (blue and red lines) and Ni (black line) films and transferred on PDMS substrate. Since the large amount of C is absorbed in Ni, the growth of high-quality graphene and proper control of the growth parameters such as reaction gas mixtures, the thickness of Ni film, and cooling rate is important. Kim et al.[35] reported a rapid cooled down process for the synthesis of Ni graphene at the rate of 10 °C/min by flowing Ar gas and an optimized thin thickness of 300 nm Ni layer was used to limit a large amount of carbon absorption. In this technique, a fast cooling rate is critical for controlling the multiple layers formation. Transmission electron microscope TEM image in Fig. 2(d) shows a different number of graphene layers grew in the different part of Ni substrate depending on the solubility of carbon atoms.

class="figure_img" id="Figure2"/>
Download
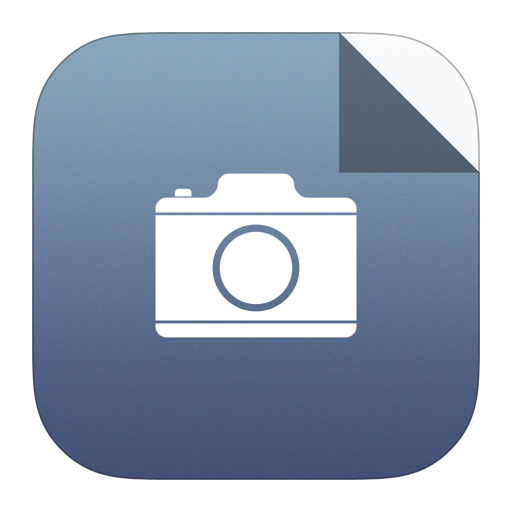
Larger image
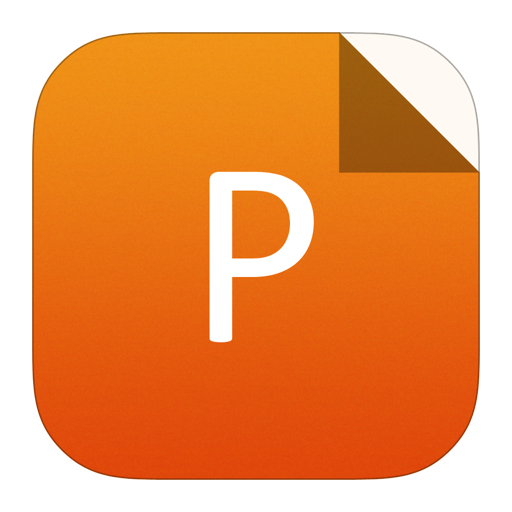
PowerPoint slide
Figure2.
(Color online) (a) Schematic diagrams of the growth kinetics of CVD-produced graphene via precipitation and surface-mediated reaction[23, 24]. (b) A photographic image of as-grown 3 inch graphene film on 300 nm thick Ni on a SiO2/Si substrate. (c) Raman spectra of the large-area graphene layers grown on Cu (blue and red lines) and Ni (black line) films, which subsequently transferred on PDMS substrate (green line)[34]. (d) TEM images of as-grown graphene films on thin(300-nm) nickel layers of different thicknesses[35]. (e) Optical microscope image of transferred monolayer CVD graphene on SiO2/Si, which was grown on Cu foil also showing wrinkles and two-and three-layer regions[22].
In the case of Cu substrate, the entire growth process (adsorption, decomposition, and diffusion of molecules) occurs only on the surface because of the self-limiting effect, which preferentially leads to the growth of monolayer graphene. Therefore, the CVD method using a Cu catalyst is introduced to the synthesis of large area monolayer graphene. Large-area graphene centimeter scale graphene films were grown on Cu substrates predominantly using methane by Li et al.[22] In particular, a 25 μm thick Cu foil first annealed at 1000 °C in a furnace with a 10 sccm H2 flow. Then the surface of Cu foil was cleaned by annealing at 1000 °C for 30 min to reduce the formation of CuO and increase the grain size. Finally, the reaction gas mixture of CH4 : H2 (15 : 10 sccm) was introduced for 30 min, followed by being rapidly cooled down to room temperature using H2. This technique proved to be almost 100% effective for achieving growth of monolayer graphene as indicated in the optical image in Fig. 2(e) with a few small regions showing the bi and tri-layer regions.
2.2
Large area transfer process
It is necessary to transfer the as-grown graphene film to assemble it into various graphene devices since the as-grown graphene film is precluded to being used directly. Therefore, an efficient transfer method needs to be developed without degrading the quality or further defect generation. There are several transfer methods that have already been established to transfer large-area graphene film[34–38]. Among them, transfer printing using poly(methyl methacrylate) (PMMA) as a carrier from the synthesized metal surface on an arbitrary substrate is believed to be the most popular technique[34, 39]. In this method, a PMMA supporting layer is spin-coated on the top of as-grown CVD graphene film on Cu to protect the top graphene surface in the following wet chemical etching process by an ammonium persulfate solution followed by rinsing with de-ionized water. Then the resultant PMMA/ graphene is floated on water, which can be transferred on an arbitrary substrate. Finally, PMMA is removed by acetone. Another advantage of this method is by repeating this transfer process multiple times, stacked multilayer graphene films can also be achieved. However, the wet transfer method using PMMA suffers from PMMA residues being left on the graphene surface, which may further introduce structural discontinuities[40]. Recently, an industry applicable efficient “roll-to-roll” (R2R) transfer method has been developed for graphene grown on a flexible Cu foil[38]. In particular, a thermal release tape is attached by adhering to the graphene/Cu by the roll process. Then Cu foil is etched chemically using Cu etchant followed by the roll transfer of graphene film onto the target substrate and releasing the thermal tape exposing its release temperature (Fig. 3(a)). This novel roll-to-roll technique is efficient of producing as large as 30 inches in the diagonal direction as shown in Fig. 3(d).

class="figure_img" id="Figure3"/>
Download
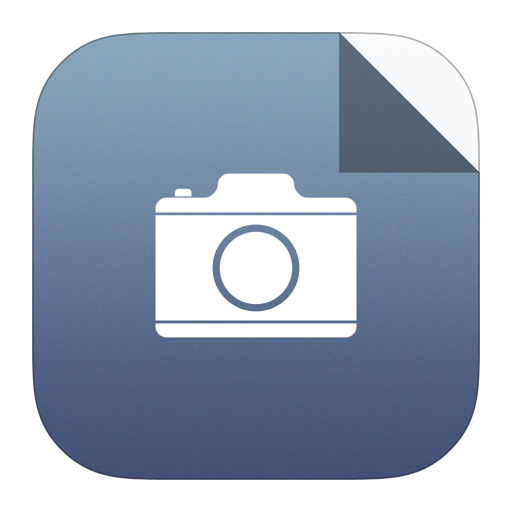
Larger image
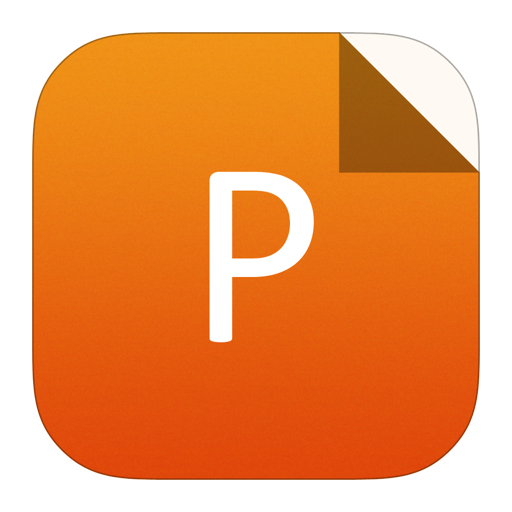
PowerPoint slide
Figure3.
(Color online) (a) Illustration of the roll-based production of graphene films grown on a copper foil[38]. (b) Schematic illustration of a face-to-face method for transferring graphene mediated by capillary bridges. (c) Image of a partially submerged 8-inch PMMA/graphene/water layer/SiO2/Si wafer in water during face-to-face transfer[41]. (d) Demonstration of transparent ultra-large 35-inch graphene film transferred on PET[38]. (e) Image of the graphene/epoxy/PET roll with a width of 230 mm PET base[42].
The conventional transfer method leaves a PMMA residue, which is unavoidable, and transfer-induced cracks and wrinkles still remains a critical challenge. Gao et al.[41] developed a face-to-face transfer method for wafer-scale graphene films and subsequent transfer on an arbitrary substrate using nascent gas bubbles and capillary bridges between the graphene film and the underlying substrate. The schematic illustration of the mechanism of an etching process using the formation of capillary bridges is shown in Fig. 3(b). Fig. 3(c) depicts a photograph of a partially submerged large 8-inch PMMA/graphene/water layer/SiO2/Si wafer in water. This approach provides the advantage of the much-reduced density of transfer defects also independent of size and shape of the substrate. In another approach, a roll-to-roll CVD was developed with a scalable CVD synthesis of graphene by Kobayashi et al.[42] using selective Joule heating of a suspended copper foil followed by the subsequent transfer process. CVD synthesis carried out at a growth temperature of ~1000 °C using a low-pressure thermal CVD system. A 100-m-long graphene film with a low sheet resistance as low as 150 Ω/sq could be transferred directly by the roll-to-roll transfer process. Fig. 3(e) shows a large area 210 mm photograph of the graphene/epoxy/PET roll.
3.
Applications
After the first physical existence of graphene in 2004[16], the growth and transfer process for graphene at a large scale was developed[38]. These growth and transfer processes have been continuously optimized as time passes[43]. Such growth and transfer processes of graphene at the large scale provided an opportunity to integrate it into several electronic applications. Here, we are addressing a few applications wherein graphene can be utilized potentially in a flexible format.
3.1
Flexible field effect transistor
Flexible FET is recognized as one of the critical components for realizing for analog and digital circuits, hence enormous efforts have been attempted for making such building blocks using various different materials such as polymer-based, oxide-based materials, and CNTs that can replace rigid ubiquitous silicon technology. However, this advancement has been limited due to their intrinsic mechanical or electrical drawbacks and technical issues related to process compatibility with the existing fabrication technology has remained the critical challenge to overcome. In contrast, novel electrical properties of graphene such as extremely high intrinsic carrier mobility, due to its massless Dirac fermions and Berry's phase are suitable for the fabrication of high-speed flexible transistors operating in the terahertz regime[44–46]. In addition to its excellent electronic properties, the excellent mechanical property of graphene to endure higher strain has made them useful in flexible, stretchable electronics. These extraordinary properties of graphene make it well suited for the fabrication of graphene-based FETs (GFET) in the evolution of next-generation flexible and stretchable electronics. Furthermore, recent progress in large area growth of high-quality graphene using CVD has opened up possibilities for the fabrication of large-array flexible, stretchable transistors. Due to its semi-metallic property, graphene can be utilized either as an active layer or as electrodes in FETs, and also it can be used as a transparent electrode in solar cells and organic lighting emitting diodes (OLEDs)[47–51]. In this section we will present the recent progress of graphene transistors based on CVD graphene only, for flexible, stretchable applications.
Novoselov et al.[16] demonstrated the first graphene FET in 2004, accompanied with the first isolation of monolayer graphene. The primary investigations of graphene's intrinsic property have been performed using an unconventional mechanical exfoliation method by physically peeling layers of graphene flake from bulk graphite using scotch tape and transferring the graphene layers on a SiO2 substrate. Although the bottom-gated transistor configuration is sufficient for investigating the intrinsic property of graphene, it is not suitable for the practical large area device applications such as wearable and flexible electronics. A number of researches on graphene-based FETs have addressed various issues and challenges related to device structure and selection of materials which can be compatible with flexible devices[52–54]. Recent progress in large area growth of high-quality graphene using CVD has opened up the possibilities for the fabrication of large-array flexible, stretchable transistors.
One of the problems with building a graphene transistor is the weak interface between graphene films and gate dielectrics. The traditional inorganic dielectric is not only difficult to form a uniform oxide film on a hydrophobic graphene surface but also inorganic materials are brittle in nature showing poor endurance to mechanical strain. However, Liu et al.[51] reported the graphene-based flexible FET using Al2O3 by employing the self-healing process. The self-healing process repairs the defects and cracks generated by bending at the gate dielectric interface, which results in the good compatibility in a flexible format. Because of the high growth temperature, high-k dielectric materials are also inappropriate for use on plastic substrates. Therefore, it is desirable to replace the rigid dielectric materials with new material, which is suitable in a flexible format maintaining a high capacitance and good interface with graphene. Solid polymer electrolytes ion gel poly (styrene-methyl methacrylate-styrene) triblock copolymer and 1-ethyl-3-methylimidazolium bis (trifluoromethylsulfonyl) imide exhibits high capacitance and an extremely high value of ~10?l F/cm2 at 10 kHz[55]. Thus, ion gel is a promising alternative because of its low-temperature compatibility with easy printing ability and high capacitance with good mechanical durability.
Kim et al.[56] demonstrated a high-performance, low-voltage graphene FET array (Fig. 4(a), inset) on plastic substrates where solution-processable, high-capacitance ion gel gate dielectrics serve as a gate dielectric. Use of ion gel dielectrics offers several advantages such as high capacitance yielded a high on-current and low voltage operation (Fig. 4(a)) and good mechanical stability proving a route to mechanically flexible, high-performance, and low-voltage graphene devices. A stretchable and transparent all-graphene transistor array with ion-gel gate dielectric using low-temperature aerosol printing methods demonstrated by Lee et al.[57] on a stretchable polydimethylsiloxane (PDMS) and rubber balloon (Fig. 4(b), top). To overcome the degradation of the average hole and electron mobilities of monolayer graphene devices due to scattering from the porous PDMS structure, a multilayered graphene channel was used where the bottom graphene screens out the external scattering effect and the top layer suppresses the external contamination. For a tri-layered graphene, the channel device improved the electrical property with a mobility of 1131 ± 96 cm2 /(V·s) was achieved with an electrical stability within an error range of 20% up to a strain level of 5% (Fig. 4(b), bottom). Kim et al.[58] represented transparent flexible graphene transistors and inverters in a simplified coplanar-gate device structure (Fig. 4(c)). The novel device structure enables the fabrication to be simplified into a two-step patterning step for inverter fabrication. The coplanar gated graphene-based FET exhibited average hole and electron mobilities of ~892 ± 196 and 628 ± 146 cm2/(V·s) respectively with good mechanical flexibility and robustness. Another novel approach is to use graphene oxide (GO) as a potential candidate for the dielectric that can make a good interface with graphene analogous to the SiO2 insulator for semiconducting Si. A high-performance, flexible all graphene-based transistor was demonstrated by Lee et al.[59] on plastic substrate employing GO as a gate dielectric and graphene as the channel, source-drain and gate electrodes (Fig. 4(d), inset). The monolithic graphene devices exhibited symmetric hole and electron mobility of ~150 and 116 cm2/(V·s), respectively, for different bending radii with a stable device operation under a tensile strain up to 3.5%. A photo-patternable ion gel gate dielectric was introduced in self-aligned flexible graphene transistors and inverters on plastic substrates (Fig. 4(e))[60]. In this approach, the photo-patternable ion gel simplifies the fabrication process by eliminating the extra graphene-patterning step since it was used as a negative photoresist for the patterning of underlying graphene as well as gate dielectrics. This technique is also advantageous for avoiding the side effect arising from the photoresist residue as the ion-gel served as a dielectric material and at the same time as an etching mask. The graphene transistors on plastic offered a low voltage operation (~2 V) with average hole and electron mobilities of 8952 ± 124 and 452 ± 98 cm2/(V·s), respectively, and fatigue mechanical stability.

class="figure_img" id="Figure4"/>
Download
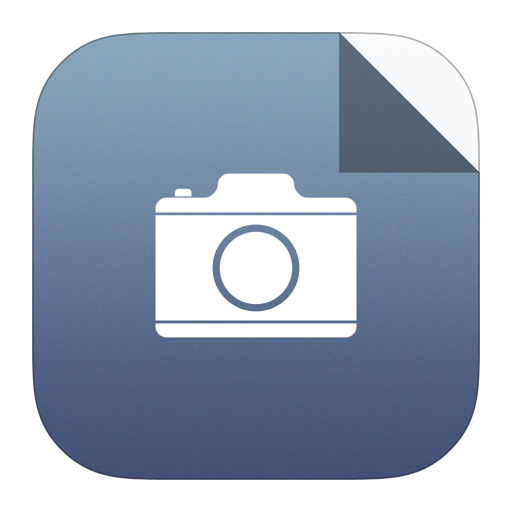
Larger image
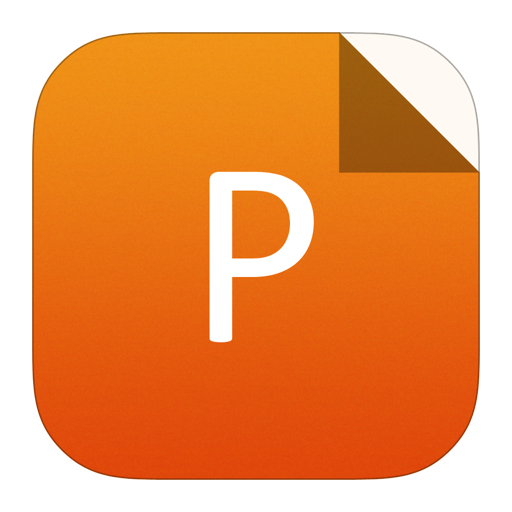
PowerPoint slide
Figure4.
(Color online) (a) Transfer (Ids–Vgs) characteristics of graphene FETs on a plastic substrate and the inset showing the images of an array of devices on a plastic substrate[56]. (b) Photographic image of stretchable ion-gel gated graphene FETs on a balloon (top): with images of graphene transistors on PDMS substrate (inset) and the normalized hole/electron mobility of graphene FET as a function of the stretching level (bottom)[57]. (c) Typical transfer characteristics (ID versus VG) of a coplanar-gate graphene transistor and photograph of the coplanar-gate graphene transistor array (inset)[58]. (d) Transfer characteristic of graphene/GO transistors, indicating the hole and electron mobilities are 300 and 250 cm2/(V·s) at VD = ?0.1 V, respectively. The inset shows the schematic bottom-gated all-graphene-based transistor[59].(e) Photograph of large-area flexible graphene transistor array (scale bar: 5 mm). The inset shows the optical image of a completed device unit[60]. (f) The de-embedded current gain with an intrinsic fT of 25 GHz for flexible GFET. VG is biased at the peak transconductance and VD = 0.5 V. Schematic of water-resistant flexible multi-finger embedded gate FET (upper inset) and flexible GFETs attached to the bending set up shown in the lower inset[55].
The lack of an intrinsic band gap limits graphene to be used in the digital application due to a low on-off ratio. But its excellent electronic properties such as high mobility and saturation velocity make it a suitable material for RF transistors with the terahertz regime. Recently there have been a few approaches to fabricate graphene-based RF devices for plastic electronics[50, 54, 55, 61–63]. As an example, Lee et al.[55] demonstrated flexible graphene RF transistors with a cut-off frequency of over 25 GHz integrating high-k dielectric. Although, achieving an intrinsic cut-off frequency of fT ~25 GHz at a modest lateral field of 1 V/μm on plastics is 50% lower than the state-of-the-art rigid substrate (Fig. 4(f)), however, the device exhibited good electrical stability within an error range of 20% at the high bending induced strain of over 8%, which is substantially larger than what has been achieved with thin-film Si and III–V flexible transistors.
Therefore, graphene’s extraordinary mechanical, optical and electrical properties have drawn enormous research interest and have been successfully attempted in various flexible, stretchable, and conformal electronic applications. The absence of the intrinsic bandgap in graphene limits its digital application; however, it can be suitable for a number of different flexible future device applications where a high on-off ratio is not necessary, for instance flexible analog electronics, such as attenuators, amplifiers, or signal mixers.
Electrodes are essential passive components for any kind of electronic devices as these are utilized either for injection or extraction of the charge carriers, therefore, the primary requirement in the selection of electrodes is to its conductivity and the formation of the minimum barrier at the interface. In this scenario, metals have been realized as the most promising candidates. However, when these are integrated into fabricating the flexible devices, then devices show degradable performance due to their poor mechanical endurance against the repeated external applied strain. Therefore, for practical realization of flexible devices, we need to look at some alternative electrode material showing excellent robustness against externally applied strain along with comparable electrical properties to metals. In addition to electrical and robust mechanical properties, transparency of the electrode materials becomes essential in some specific applications such as touch screens, solar cells, displays, smart windows, light emitting diodes etc[45, 46], wherein, currently ITO with good conductivity (10 Ω/sq) and transparency (90%)[64–66] covered the whole commercialized market. Further, ITO is not compatible with flexible devices because of its poor mechanical behavior and being unable to withstand external mechanical deformations. People explored many materials as an electrode, for example, metallic nanowires, nano particle-based inks, carbon nanotubes, and graphene[67–71]. Among all these, graphene showed excellent potentiality due to its transmittance (90%), conductivity (30 Ω/sq), and good flexible behavior[44, 72]. In addition to these, graphene is chemically and environmentally stable and can easily be synthesized in 2-dimensions at large scale, which is also a primary requirement from the industry point of view, thus, it is a most promising material for replacing ITO for flexible applications. There are several reports wherein graphene is potentially integrated as an electrode in demonstrating the flexible applications, which are briefly described below.
3.2
Touch based flexible screens
A touch screen is a part of the display which takes command if one physically touches its top surface and then responds depending on the position of the touch[73]. Touch-based operation of a display screen eliminates the additional hardware part, thus, reduces the extra electronic component and makes it more convenient as well as user-friendly. Touch operations can be of resistive, capacitive, projected capacitance, surface capacitance, and surface acoustic wave types. Among all, resistive and capacitive type operations are frequently used in commercialized applications, however, such operation requires the electrode materials having sheet resistance and transparency of the order of 300–1500 Ω/sq and 85%–90% at 550 nm, respectively[73]. Bae et al. successfully demonstrated the synthesis of graphene film and its transfer process at large scale using R2R technology[38], which showed the low value of sheet resistance ~125 Ω/sq and good transparency ~97.4% once printed on PET substrate. Graphene film was transferred by the dry as well as wet transfer method using the R2R process, and it has been observed that the dry assisted transfer yields more physical damage to graphene films, thus, consequently resulted in relatively higher sheet resistance as compared to PMMA assisted wet transfer. However, in case of stacked graphene films using multiple dry transfers, the value of sheet resistance becomes much lower than the wet transferred one. Further, reduction in sheet resistance up to 30 Ω/sq in 4-layers graphene films was realized with HNO3 as a p-type dopant, however, in this case, transmittance reduces up to ~90%, which is still fine for touch-based applications. Such excellent optical and electrical properties of graphene along with its flexible behavior seem to be somewhat superior to the commercially utilized ITO electrode. These graphene films with an exceptional combination of electrical, optical and flexible properties were utilized in fabricating the touch screen panel. For fabricating the touch screen, a screen printing process is utilized as shown in Fig. 5(a). First, electrodes and then dot spacers were printed, and after that graphene/PET-based top and bottom panels were arranged in such a way that if the top panel surface is pressed then electrodes of the top and bottom panels come in contact, and the resistance at the point of contact changes, consequently current flows. The whole assembly is connected to a controller installed in a computer that reads-out the signal resulted in from the contact. The electrotechnical behavior of a graphene-based touch screen was tested against the applied tensile strain and compared with the ITO based touch screen as shown in Fig. 5(b). It has been observed that the graphene-based touch screen could withstand up to 6% of applied external strain while the ITO based one could only withstand up to 2%–3%, which indicated the promising feature of graphene films in flexible devices. The touch screen was very well operated and its operation could easily be seen by connecting to a computer that was confirmed by taking a photograph during its operation, as shown in Fig. 5(c). The fabricated touch screen using graphene films could easily be shown by the photograph in Fig. 5(d) that confirms its folding behavior with excellent transparency. The R2R method for the etching and transfer process was also utilized by Ryu et al. in synthesizing the graphene films by employing the hydrogen free rapid thermal CVD (RT-CVD) method. This hydrogen free RT-VD method with R2R resulted in the fast and large area growth of graphene films of uniform size of 40 × 30 cm2. The sheet resistance of graphene films with the RT-CVD method was achieved as low as 250 Ω/cm, which was found to be stable over 12 weeks in an ambient environment with little fluctuations 250 ± 36 Ω/cm[74]. Additionally, the synthesized graphene films on PET showed relatively better optical transparency as compared to ITO on the same PET substrate, which made it more superior than conventional ITO. Further, these synthesized graphene films were integrated into fabricating the capacitive based multi-touch screen. It has been found that this graphene films-based multi-touch screen was able to operate a mobile phone, as shown on the left side of Fig. 5(e) whereas the right side of Fig. 5(e) shows the ITO based touch screen in a mobile phone for comparison purposes.

class="figure_img" id="Figure5"/>
Download
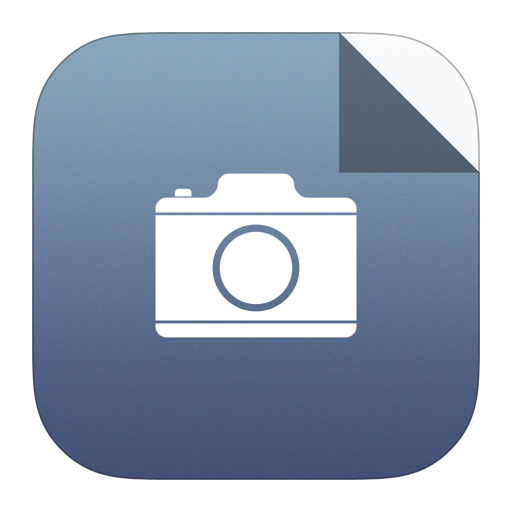
Larger image
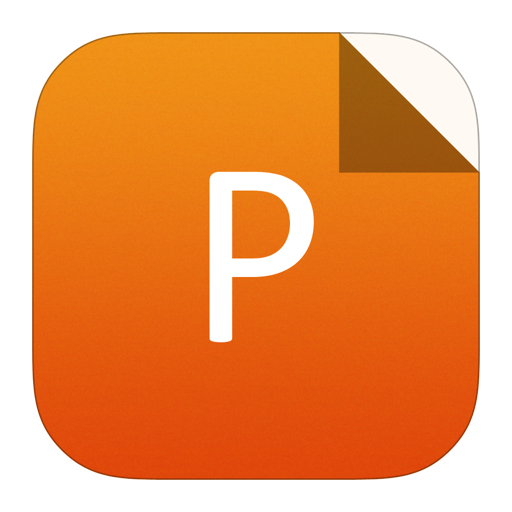
PowerPoint slide
Figure5.
(Color online) (a) The screen printing process utilized in fabricating touch screen. (b) Electrotechnical behavior of graphene and ITO based touch screens against the applied tensile strain. (c) Photograph of graphene-based during its operation. (d) Photograph of graphene-based touch screen in bending form[38]. (e) Photograph of graphene films based multi-touch screen integrated into a mobile phone (left side) whereas the right side shows the ITO based touch screen in a mobile phone for comparison purposes[74].
3.3
Flexible organic light emitting diodes
A device that converts the electrical energy into light energy of a particular or mixed wavelength upon applying the voltage/current is conventionally termed as an LED. Such devices can be exploited as a source of light in several applications for example, it can be used as a tiny indicator in various portable electronic gadgets, as a back-light source in display screens etc. For display applications, organic based LEDs (OLEDs) are coming up with excellent features over conventionally available liquid crystal displays (LCDs) and LEDs. Such organic based displays are brighter, need less power to operate, and do not require a back light source; in addition, these are flexible, light weight, and thinner. OLEDs consist an electroluminescent polymeric layer wherein the emission of light occurs, thus called the active layer, which is sandwiched between two electrodes, anode, and cathode. The anode injects the holes while the cathode injects the electrons in the highest occupied molecular orbitals (HOMO) and the lowest occupied molecular orbitals (LUMO) of the electroluminescent organic layer, respectively. Another primary requirement of OLEDs is that one of the electrodes must be optically transparent so that the emitted light can come out. Since light emission in OLEDs occurs in an electroluminescent organic layer wherein the recombination of electron and hole takes place, thus, the efficient recombination produces the high-performance emission. The efficiency of recombination depends on how efficiently electrons and holes are injected from anode and cathode to HOMO and LUMO of the organic layer, respectively. Further, such injection depends on the matching of work functions of respective electrodes and organic layers bands. Currently, people are using the well-known conventionally available transparent conducting electrode ITO, which has a work function value of ~4.5 eV for organic-based LEDs display. However, the increasing cost of indium and the poor mechanical endurance of ITO against externally applied strain makes it inferior for flexible applications. Additionally, the other serious drawback by which ITO suffers is its indium (In) diffusion in the electroluminescent organic layer, thereby degrading the OLED performance over time. Again, as we described above, graphene is a conducting, transparent, flexible and more importantly it is chemically inert and its work function matches with ITO, thus it can be a potential alternative candidate of ITO that can overcome all these issues related to ITO and can be successfully integrated as a transparent electrode in fabricating flexible OLEDs based displays. There are many reports where graphene has been used as a transparent electrode in fabricating OLEDs. In this quest, the first attempt has been taken by Wu et al.[75]. They synthesized reduced graphene oxides (rGO) films via solution processing and employed it as an anode in fabricating the OLEDs. The performance of rGO based OLEDs was compared over the ITO based OLED device. The rGO based OLED device showed the luminescence of 300 cd/m2 at 11.7 V while for ITO this value of luminescence was observed at 9.9 V. Further, the turn-on voltages for rGO and ITO based devices were estimated as 4.5 and 3.8 V, respectively. Since the OLED devices were fabricated using the solution process based rGO films, which in practice shows higher sheet resistance due to the presence of a large number of defects and multiple grain boundaries, thus, the fabricated devices showed moderate performance. Although, these OLEDs devices showed moderate performance but this work opened a feasible way for graphene-based OLEDs, as an alternative to ITO based devices. Therefore, graphene with improved electrical properties was expected to result in much better performance as compared to ITO based OLEDs. After that Matyba et al.[47] fabricated an electrochemical cell that could emit light. In this light-emitting electrochemical cell, chemically derived graphene was used either as an anode or cathode and a polymeric layer made of blending of poly (para-phenylene vinylene) copolymer called “Super Yellow” with an electrolyte acting as an active layer. The beauty of this light-emitting electrochemical cell is that the charge injection does not depend on the electrode’s work functions. A high charge density occurs at the electrode’s interface against applied potential, which assists the efficient charge injection regardless of work function. In this ongoing context, Chang et al.[76] also demonstrated the flexible OLEDs wherein the anode material was synthesized as a composite based on rGO and PEDOT:PSS. The composite electrode showed electrical and optical performance comparable to ITO, but, the fabricated OLED device using this electrode showed much better mechanical robustness against external applied bending as compared to ITO. All these above-described OLED related reports are based on the solution process synthesis of rGO films and showed moderate performances mainly because of two reasons: (1) high sheet resistance and (2) different work function values of anode and hole transporting layer. However, after the successful demonstration of R2R process based large area synthesis of graphene films[38], a high-performance OLEDs could be realized by utilizing CVD graphene films. CVD graphene was successfully integrated by Han et al.[77] for the first time and they fabricated the flexible OLED. The flexible OLED showed high performance as compared to the ITO based OLED device. The improvement in the CVD based OLED device was due to its low sheet resistance as compared to the chemically derived one and further improvement could be expected by the combined effect of optimizing the graphene stacking layers and AuCl3 or HNO3 based p-type doping, that could lower the sheet resistance value as low as 30 Ω/sq. Moreover, the work function mismatch related issue was resolved by inserting a tunable work function based conductive polymeric hole injecting layer (GraHIL) that facilitates the efficient hole injection as shown in Fig. 6(a). The anode electrode was prepared using different stacking layers of graphene and doped with HNO3 or AuCl3. Based on these prepared electrodes, a number of devices were fabricated, as illustrated schematically in Fig. 6(b), and their performances were compared to ITO based devices. In both kinds of OLED devices, either graphene or ITO based, the hole injecting layer, GraHIL, was inserted. The performance of the green fluorescent device based on 4 layers of stacked doped graphene films was found to be superior as compared to ITO based devices. Fig. 6(c) shows the variation of luminous efficiency, which is estimated as 37.2 and 27.4 lm/W for graphene and ITO based devices, respectively. The performance of the phosphorescent device was also found to be higher over the ITO one by utilizing the 4 layers doped graphene electrode. When these OLEDs devices had gone through repeated bending cycles, then as expected graphene-based OLED showed great robustness against strain and almost the same value of current density even after 1000 bending cycles, while, in the case of ITO, the devices could not even survive after 800 cycles. It again confirmed the potentiality of graphene in flexible applications. Fig. 6(d) shows the photograph of 4 layers doped graphene films based green fluorescent OLED in bended form showing its flexible behaviour. After that, a few reports were coming up from time to time for demonstrating the graphene-based OLED with work function engineering[78]. In addition to the work function mismatch and sheet resistance, there is one more parameter, called outcoupling, that could also hinder the device efficiency. People proposed some geometries to improve the outcoupling issue[79]. Among all, microcavity resonance having planar geometry was found to be the most suitable one as it can easily eliminate the electrical shorts and some artefacts for example blurring, consequently, provide the efficient emission of red, blue and green colours. People initially tried ITO for enhancing such microcavity based outcoupling by tuning its thickness[80, 81], however, if one wants to apply a similar approach for graphene-based OLEDs then it is difficult as it is hard to synthesize thick graphene that could efficiently control the cavity length. To overcome this hurdle, Lee et al.[78] proposed a different type of architecture for an electrode in which graphene was sandwiched between a low index hole injecting layer (HIL) and high index TiO2. The schematic armament of this assembly is shown in Fig. 7(a). In this proposed architecture, high index materials behave like a reflector, which enhances the reflectance, and thus increases the resonance values. On the other hand, a low index HIL layer reduces the possibility of surface plasmon polariton, which helps to design the first order cavity. The high index reflector and low index HIL in synergetic manner could efficiently control cavity resonance and suppression of polaritons, which further maximized the external quantum efficiency upon optimizing the conditions. The different set of anode electrodes were prepared using TiO2/Graphene/GraHIL, graphene/GraHIL and ITO (185 nm)/GraHIL assembly, and green phosphorescent OLEDs were fabricated based on all these electrodes. The electrode with the TiO2/Graphene/GraHIL structure showed the 40.8%, 168.4 cd/A, and 160.3 lm/W values for EQE, current efficiency (CE), and power efficiency (PE), respectively. These numbers were estimated to be 31.7%, 119.0 cd/A, and 112.6 lm/W for the graphene/GraHIL based assembly. Further, for the ITO/GraHIL structure the numbers were 27.4%, 106.2 cd/A, and 104.3 lm/W, respectively. The photograph of the fabricated OLED device is shown in Fig. 7(b) while it was operated. The values of these parameters (EQE, CE and PE) were again improved by connecting the half-ball lens as a coupler to the substrate in fabricating the TiO2/graphene/GraHIL based OLED. The half-ball lens coupling increases the parameters up to 64.7%, 257.0 cd/A, and 250.4 lm/W, respectively. The potentiality of the proposed structure was explored in demonstrating the flexible OLED device. In this flexible OLED, the TiO2/graphene/ PEDOT:PSS based structure was employed, wherein, PEDOT:PSS was having the similar role as of GraHIL. Since the refractive index of PEDOT:PSS is a bit higher than the used GraHIL, therefore, the device performance was observed to be a bit inferior. The variations of EQE and PE with respect to luminance for this flexible OLED are shown in Figs. 7(c) and 7(d), respectively. Further, the flexible OLED was tested under repeated bending. The variation of luminance with respect to current density under bending cycles is shown in Fig. 7(e). The flexible OLED was found to behave robustly against external strain, for bending radii as small as 2.3 mm, and remain operative up to 1000 bending repeated cycles. Here, it is strange that even by using the TiO2 layer, which is inorganically brittle having a poor fracture level, the TiO2/graphene/PEDOT:PSS based flexible OLED showed excellent robustness even up to 1000 bending cycles. It was attributed to the 4% strain sustainability of TiO2 as the cracks were found to appear in zigzag directions. A photograph of flexible OLED is shown in Fig. 7(f) during its operation mode.

class="figure_img" id="Figure6"/>
Download
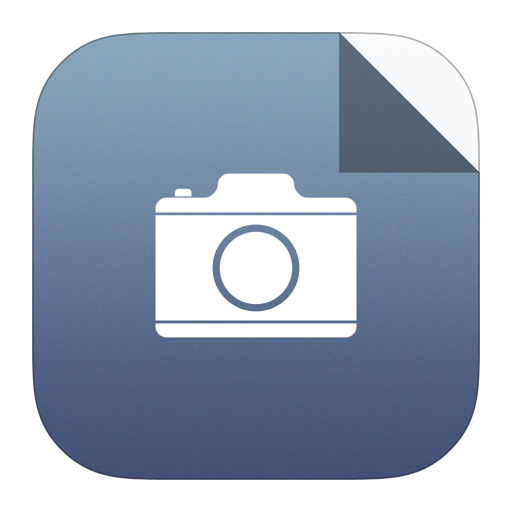
Larger image
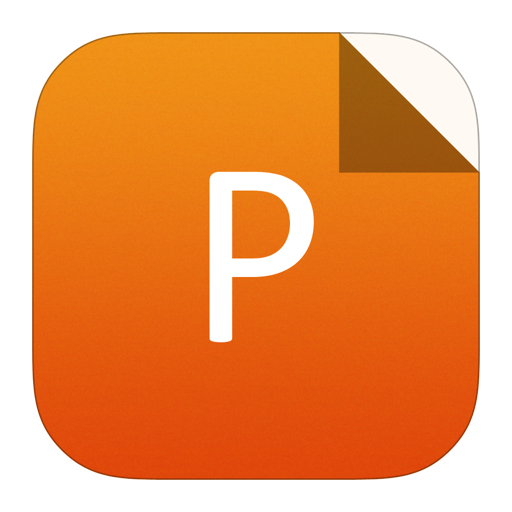
PowerPoint slide
Figure6.
(Color online) (a) Schematic representation of a hole-injection process through a self-organized HIL having a tunable work-function (GraHIL) from a graphene anode to the NPB layer. (b) Schematic flow of the fabrication steps of OLED. (c) Variation of luminous efficiency of OLED devices using various graphene layers (doped with HNO3 or AuCl3) or ITO as an anode. (d) Photograph of green fluorescent OLED in the bent form using 4 layers doped graphene films showing its flexible behavior[77].

class="figure_img" id="Figure7"/>
Download
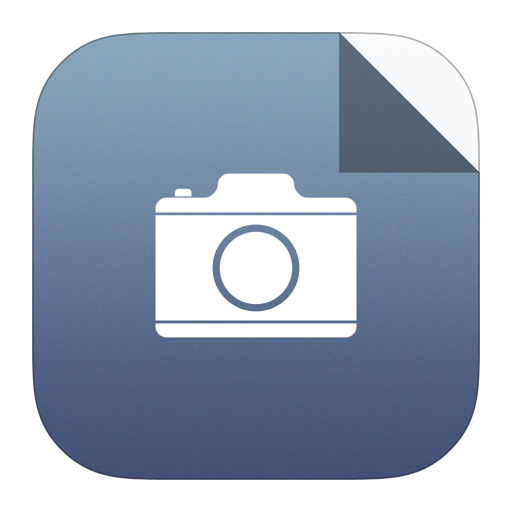
Larger image
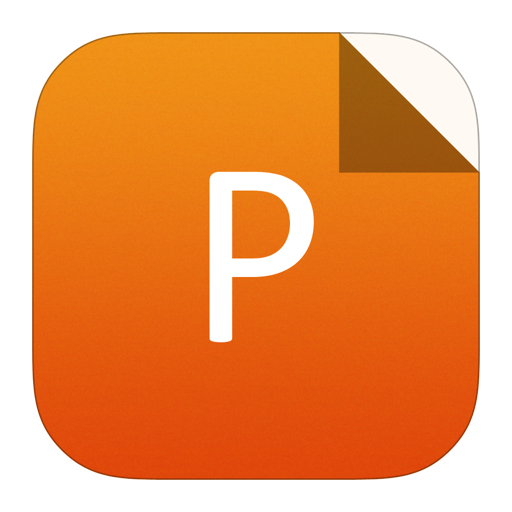
PowerPoint slide
Figure7.
(Color online) (a) Schematic device structure showing the graphene sandwiched between low index hole injecting layer (HIL) and high index TiO2 for making the high-efficiency graphene-based OLEDs. (b) Photograph of the fabricated OLED device. (c) & (d) Variation of EQE and PE with respect to luminance for flexible OLED, respectively. (e) Variation of luminance with respect to current density under bending cycles. (f) Photograph of flexible OLED during its operation mode [78].
3.4
Flexible solar cells
A device that produces the electron-hole pairs upon the absorption of sun light, and then these generated charge carriers are separated and extracted through the electrodes at opposite end, is called a solar cell. If these extracted charge carriers are substantially enough then a significant amount of voltage/current developed across the electrodes, which can further be utilized to derive the electronic appliances. Broadly, the whole issue is all about how significantly one can generate the charge carriers (i.e. maximum absorption in active layer) and then effective extraction before they get recombined, which decides the solar cell power conversion efficiency (PCE), in terms of which the device performance is judged. The PCE is calculated using the expression, PCE = (FF × ISC × VOC/Pin) × 100, where FF is the fill factor (described as the IPmaxVPmax/ISCVOC), ISC is the short-circuit current, VOC is the open-circuit voltage, and Pin is the incident optical power. The harvesting of solar energy, presented to address several challenging issues to the researchers worldwide, for example the need of highly transparent and conducting electrodes which must be chemically stable with respect to photoactive materials, the role of the electrode’s work function, insertion of an efficient electron/hole blocking layer etc. Here, we are summarizing only those reports which are related to transparent conducting electrodes that could show excellent performance in a flexible format. As we described above, graphene can easily find a suitable place in any electronic device wherever the electrodes having high conductivity, transparency, and excellent flexibility is required, for this obvious reason, again a graphene was a suitable choice to integrate it as a TCE in solar cell devices. After the first report of the physical appearance of graphene, a dye-sensitized solar cell was fabricated in 2008 by employing the exfoliated graphene as an electrode[82]. People have also tried the solution process based chemically derived graphene films[83–85], but as we know such graphene films always suffer from high sheet resistance, so, the obtained device performances were poor. The improvement in solar devices was observed after utilizing the CVD graphene, but still there is a huge gap which needs to be overcome to reach up to commercially available Si-based solar cells efficiency ~25%[86, 87]. Organic solar cells pose a hydrophobic surface over which it was found to be difficult to spread the hole blocking layer, thereby, these organic solar cells suffer from moderate performances[88]. People have tried to address this issue by modifying the graphene work function and surface properties with doping[89]. It was found that the performance of a solar device could be improved by doping the graphene anode with AuCl3, which basically changes its surface to hydrophilic[89]. These solar cell devices either fabricated with chemically derived or CVD based graphene films were tested in terms of flexibility[76, 77], where, PCE values were found to fall within the range of 1%–2%[90–92]. However, some sorts of improvements were observed from time to time for graphene-based solar cells, for example, Lee et al.[93] used HNO3 doped multi-layered graphene films for fabricating the flexible solar cells and tested them under bending. The flexible solar devices showed the PCE value of 2.5% even for 2.5 mm bending radii. Further, Liu et al.[94] added HAuCl4 into PEDOT: PSS and doped the CVD graphene films with this mixture. The doped CVD graphene films were used as an anode electrode for fabricating the flexible solar cell that could show the PCE value of 3.2%. In this case, the doping effect of PEDOT:PSS over graphene was enhanced by adding HAuCl4, and top layers of graphene films in the electrode were protecting the device from air contamination. The flexible solar cell showed good robustness against bending and it was found that the device showed only 8% decrement in PCE after 1000 bending cycles. Kim et al.[95] prepared the GraHEL by blending the PEDOT:PSS with less hydrophilic tetrafluoroethylene-perfluoro-3,6-dioxa-4-methyl-7-octenesulphonic acid copolymer (one of the perfluorinated ionomers (PFI)). This GraHEL improved the coating quality on top of the hydrophobic graphene surface, which improved the PCE value up to 4.33% for the flexible solar cell. Considering the above-described solar cell related works, it was noticed that the electrical properties of CVD grown graphene films are still inferior over ITO, especially in the solar cell context. Kim et al.[96] attempted the nonvolatile electrostatic doping in CVD graphene films for fabricating the flexible solar cells. The electrostatic doping in graphene films was realized by the polarization of ferroelectric polymer, poly(vinylidene fluoride-co-trifluoroethylene) (P-(VDF-TrFE)) on graphene film, which resulted in the PCE value of 2.07%. A schematic representation of an electrostatically doped organic solar cell is shown in Fig. 8(a). The sheet resistance of graphene films was found to decrease significantly after electrostatic doping over unpolarized, and it was noticed that the electrostatic doping remained more stable as compared to chemical based doping. The current-voltage characteristics and overall performance of the solar cell with electrostatic doping was found to be better than the one without doping. Fig. 8(b) shows the performance of the solar cell in terms of current density (square) and PCE (triangle) for different numbers of graphene layers. The filled and open symbols represent the characteristics of undoped and with electrostatically doped devices, respectively. The flexibility of the organic solar cell was tested under bending; for that the device was attached to a rolling machine as shown in Fig. 8(c). The current-voltage and normalized parameters of the solar cell device for flexible format are shown in Figs. 8(d) and 8(e), respectively. After that a significant improvement in PCE values has been realized by the work of Park et al.[48] by fabricating the flexible solar cell using graphene-based anode and cathode electrodes. They have achieved the PCE values up to 6.1 and 7.1% by utilizing graphene as anode and cathode, respectively. Such high values of efficiency were attributed to the presence of MoO3 and ZnO layers, which acted as electron blocking and transporting layers on graphene, respectively. Fig. 8(f) shows the photograph of a fabricated flexible solar cell. Figs. 8(g) and 8(h) show the solar cell performance parameters such as FF, Voc, Jsc, and PCE under flexible test.

class="figure_img" id="Figure8"/>
Download
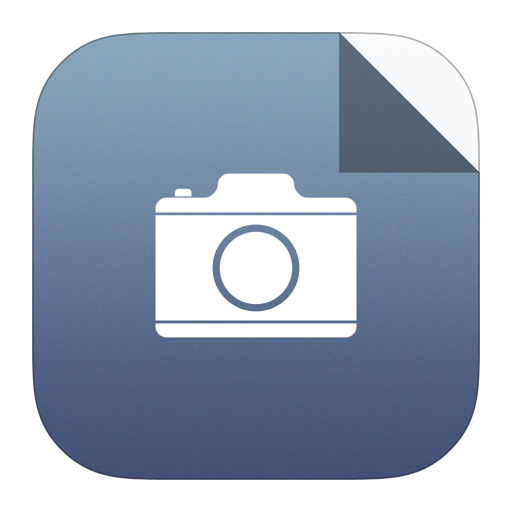
Larger image
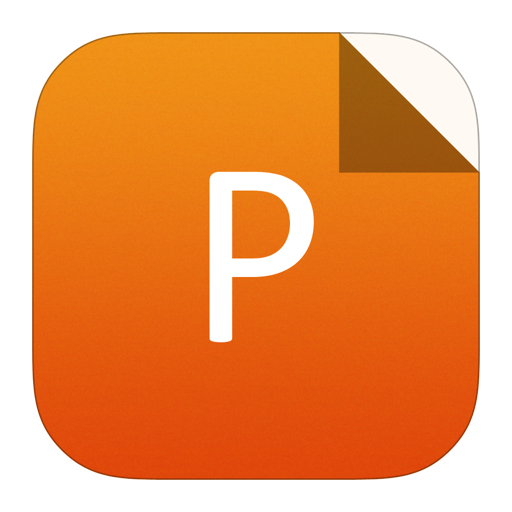
PowerPoint slide
Figure8.
(Color online) (a) Schematic representation of the electrostatically doped organic solar cell. (b) Shows the variation of current density (square) and PCE (triangle) for different numbers of graphene layers. The filled and open symbols represent the characteristics of undoped and with electrostatically doped devices, respectively. (c) Photograph of the flexible organic solar cell attached to the rolling machine. (d) & (e) Variation of current density with voltage and normalized parameters of solar cell device, respectively in a flexible format. (f) Photograph of the fabricated flexible solar cell. The inset shows the schematic representation of device assembly. (g) & (h) Show the solar cell performance parameters such as fill factor (FF), open circuit voltage ( Voc), short-circuit current density (Jsc), and PCE under flexible test[96].
3.5
Flexible sensors
Graphene showed an excellent potentiality in the flexible transparent conducting electrode and seems to be a potential alternative for replacing ITO. In addition to this, it also presents some sensing features such as piezo-response, electrochemical activity upon chemical treatment etc. Further, these sensing features with the combination of its transparency, conformal attaching ability due to the ultra-thin thickness and good stretchability allow its easy integration in fabricating the flexible and wearable sensors that could be easily attached conformably to human body parts or any kind of curvy surface. There are several reports on graphene-based sensing applications[97–99]. However, we are summarizing those reports which had set a bench mark till now. A transparent stretchable rosette-type strain sensor was reported[100], which was capable of detecting the direction as well as the strain value simultaneously. The device was conformally attached to wearable gloves and it showed the significant change in resistance when it deformed as shown in Fig. 9(a). Park et al.[101] reported the graphene-based tactile sensor that can conformally attach to a very rough surface. The tactile sensor showed an excellent response when subjected to a gentle touch. The corresponding pressure map is shown in Fig. 9(b). Yang et al.[102] also fabricated the tactile kind of sensor by utilizing the graphene-based microfabrics that can be easily woven. The fabricated tactile sensor was mounted on the wrist, which could measure the response from the wrist pulse as shown in Fig. 9(c). Recently, Kang et al.[103] demonstrated the graphene-based 3-dimensional capacitive type touch sensor. Fig. 9(d) shows the photograph (upper panel) of a graphene-based capacitive touch sensor mounted on the human hand, and its responses under multi-touch, spreading and scrolling are shown in the lower panel. Ameri et al.[104] demonstrated the graphene-based tattoo-like epidermal sensor. The graphene electronic tattoo (GFT) was fabricated by employing the “wet transfer, dry patterning” method. The total thickness, optical transparency, and stretching limit were estimated to be 463 ± 30 nm, 85% and 40%, respectively. Further, the GFT based skin hydration sensor and temperature sensors were demonstrated as shown in Figs. 9(e) and 9(f) respectively. Kim et al.[105] developed the multifunctional contact lens sensor by which one can monitor the glucose within tears and intraocular pressure. Fig. 10(a) shows the schematic representation of a transparent glucose sensor mounted on a contact lens. Fig. 10(b) shows the wireless sensing behavior of glucose concentration with and without wearing the contact lens on a rabbit eye, whereas, Fig. 10(c) shows the frequency response of the sensor during a pressure cycle. This kind of work which has the capability of real-time monitoring of biomarkers contained in body fluid is a bench mark in the graphene-based wearable field. Further advancement was brought by Lee et al.[106] by demonstrating the stretchable device by which one can monitor diabetes and feedback therapy. This was realized by improving the electrochemical activities on the graphene surface. The fabricated device consists of many electronic components such as a heater, temperature, glucose, humidity, pH sensors, and micro needles that can deliver drugs upon thermal activation. Fig. 10(d) shows the optical image of a diabetes patch under mechanical deformation. Fig. 10(e) shows the monitoring of glucose and pH in vitro measurement under hyperglycaemia in artificial sweat. A photograph of the graphene-hybrid electrochemical device array on the human skin is shown in Fig. 10(f). Monitoring of the glucose concentrations with time in the sweat and blood of a human is shown in Fig. 10(g). A comparison of the average glucose concentrations with the commercial glucose before and after correction using the measured pH was carried out, which is shown in Fig. 10(h). These graphene-based sophisticated sensing applications present the graphene potentiality in wearable sensors, especially in the field of medical sciences.

class="figure_img" id="Figure9"/>
Download
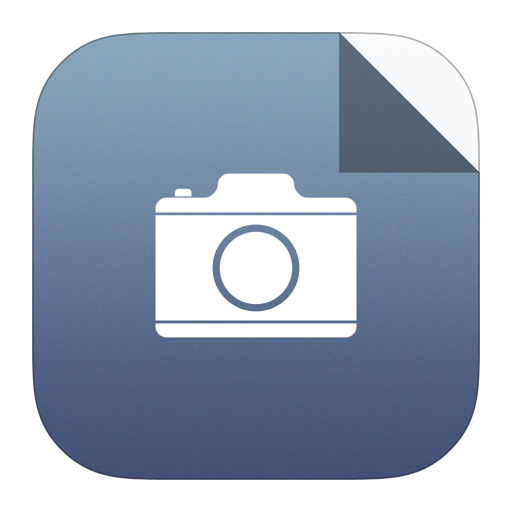
Larger image
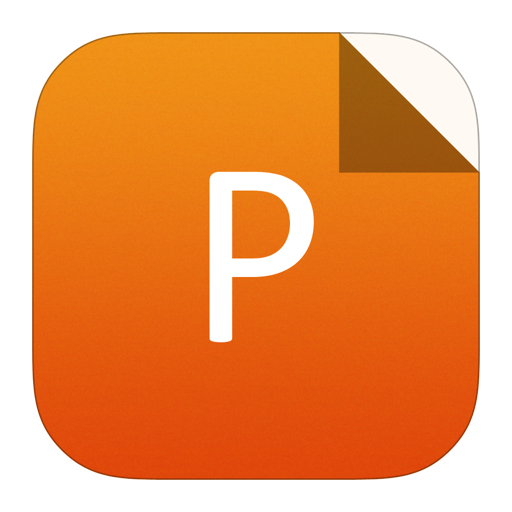
PowerPoint slide
Figure9.
(Color online) (a) Graphene-based strain sensor showing the significant change in resistance upon stretching. The inset shows the photograph of a sensor mounted conformally on wearable gloves[100]. (b) Shows the variation in pressure map when a tactile sensor is subjected to a gentle touch of 9 kPa[101]. (c) The fabricated tactile sensor shows the response from the wrist pulse[102]. (d) Photograph (upper panel) of graphene-based capacitive touch sensor mounted on the human hand and its responses are shown in the lower panel upon multi-touch, spreading and scrolling[103]. (e) & (f) Response of graphene electronic tattoo (GFT) based skin hydration sensor and temperature sensors, respectively [104].

class="figure_img" id="Figure10"/>
Download
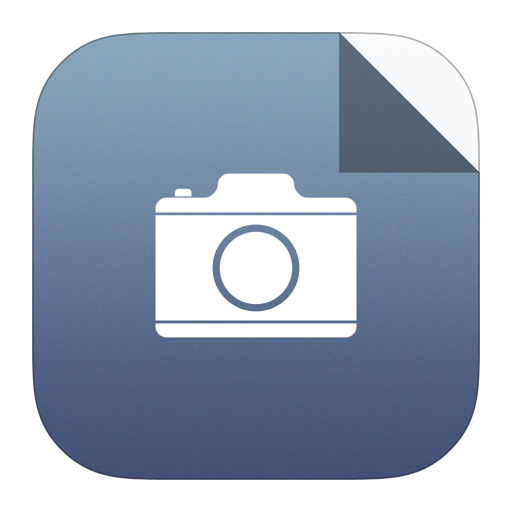
Larger image
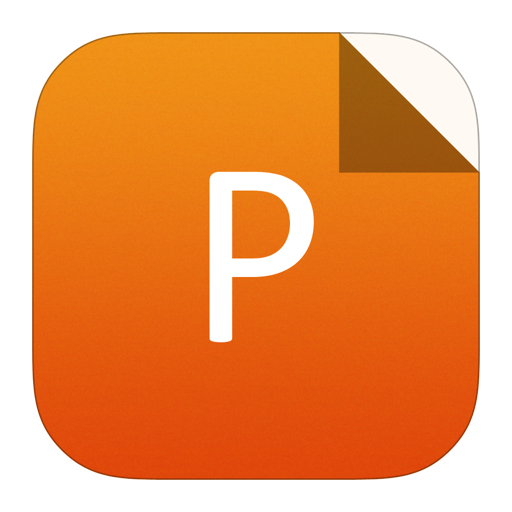
PowerPoint slide
Figure10.
(Color online) (a) The schematic representation of a transparent glucose sensor mounted on a contact lens. (b) The wireless sensing behavior of glucose concentration with and without wearing the contact lens on a rabbit eye. (c) The frequency response of the sensor during a pressure cycle[106]. (d) Optical image of the diabetes patch under mechanical deformation. (e) Monitoring of glucose and pH in vitro measurement under hyperglycemia in artificial sweat. (f) Photograph of graphene-hybrid electrochemical device array on the human skin. (g) Monitoring of glucose concentrations with time in the sweat and blood of a human. (h) Comparison of the average glucose concentrations with the commercial glucose before and after correction using the measured pH was carried out[105].
3.6
Flexible energy harvesting devices
The possibility of the conversion of unused abundant mechanical energy generated from human body motion into electrical energy inspired a revolutionary approach of nano-energy harvesting. The concept of nanogenerators (NGs) developed to operate electronic devices by generating power from human activities or body motion such as muscle movement, heartbeat, breathing, and more to operate electronic devices[107]. The opportunity of such a self-powered energy harvester is huge as it can substitute conventional large size batteries with limited life time[108]. Moreover, these devices have found applications in sustainable, self-powering biomedical sensors, infrastructure monitoring, and security systems. Presently, enormous studies have been carried out on various energy harvesting devices that can covert mechanical energy into electrical energy based both on piezoelectricity and triboelectricity[109–111]. Still, several challenges such as short life time and poor mechanical property need to be overcome for practical applications. Furthermore, uses of a metal electrode in inorganic nanogenerators fail to meet the criteria for touch-screen and wearable skin sensors where optical transparency is required. Therefore, there is a need for the search for an ultrathin, transparent, and flexible electrode material for next-generation NGs. Due to its high flexibility and excellent optical transmittance graphene has emerged as a promising candidate for electrode material in future wearable device applications.
Recently, prototype piezoelectric nanogenerator (PENG) devices based on one-dimensional piezoelectric semiconductor materials with wurtzite structure (ZnO, CdS, and GaN), thin-film insulators PbZr0:52Ti0:48O3 (PZT) and poly (vinlyidene fluoride) (PVDF) with high piezoelectric constant, have been widely studied and demonstrated[112–119]. Bae et al.[120] demonstrated a graphene-based energy harvesting system by combining single micro fiber size ZnO nanowires (NWs) and graphene. The proposed system can harvest power from mechanical energy as well as solar energy and store it in the nanowire-based supercapacitor. Kwon et al.[121] demonstrated a PZT thin film based NGs, compatible with conventional batch fabrication process, using graphene as interdigitated electrodes using a low-temperature transfer process. The use of graphene also enjoys the benefit from its excellent mechanical, optical, and electrical properties along with the excellent flexibility and transparency achieved in PZT based nanogenerators. The poor mechanical property of the PZT based inorganic nanogenerator restricted the flexibility of the resulting device instead of the good performance of graphene. As an alternative, a polymer piezoelectric material (PVDF-TrFE) based NG by sandwiching between two electrodes built to form the actuator and NG. The device exhibited excellent mechanical stability under mechanical stress up to 100 cycles.
The triboelectric nanogenerator (TENG) is another class of nanogenerator that operates based on the triboelectric effect of material such as the electron affinity, work function, chemical structure, surface roughness, and humidity. When two dissimilar materials with different charge electron affinities come into physical contact, triboelectric charge can be generated. Due to the presence of different electrostatic charges by separating the two materials, a potential drop is created between the top and bottom surface, thus an electron flows from material to external load. Khan et al.[122] recently developed a graphene tribotronic touch sensor by combining a single-electrode-mode triboelectric nanogenerator (S-TENG) and a graphene FET. In their proposed system the current transport of graphene FET modulated by the charge produced by the S-TENG when any object comes in contact with its friction layer due to the triboelectric effect. Tribotronic sensors exhibited good sensitivity below the detection less 1 kPa level with a response time of ≈ 30 ms. Because of its ultrathin thickness, the device array could attach on skin, offering a future route for numerous artificial intelligence applications (Fig. 11(a)). Using simple surface texturing and plasma treatment a graphene-based skin-attachable conformal ultrathin TNG was demonstrated by Chu et al. (Fig. 11(b), top)[123]. The fluorinated functionalized PDMS surface enlarged the surface area and electron affinity, as a result the output peak power of the device increased significantly from 3.4 to130 μW. The relatively lower thickness (2.4 μm) of this TENG device allowed it to be attached on the human skin, which is promising for wearable electronics. Moreover, as a practical application, the skin mounted device could generate Morse code with the help of an assistive communicative device, which could transmit the word and be displayed on a smartphone. Kim et al.[124] demonstrated deformable graphene-based TENGs (GTENGs) that can empower various displays such as LCD, LEDs, and an EL display without any other external energy source (Fig. 11(c), top). The output power in the device reduced linearly as the number of graphene layers increased due to the puckering effect and electron-phonon coupling (Fig. 11(c), bottom).

class="figure_img" id="Figure11"/>
Download
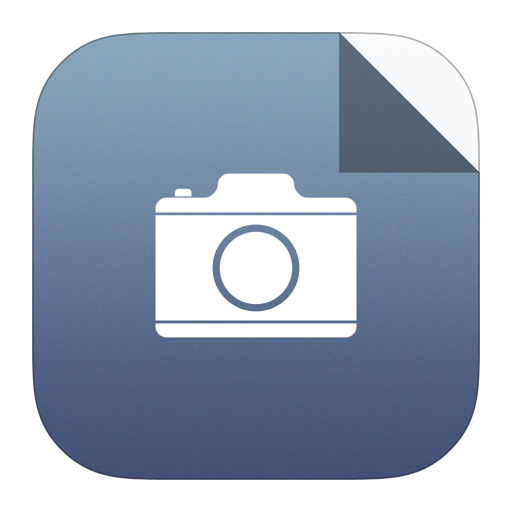
Larger image
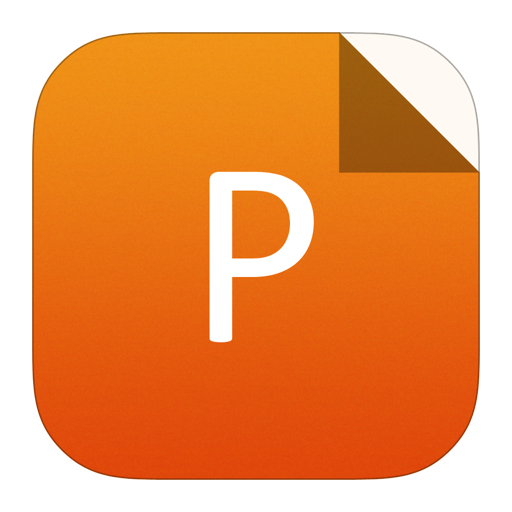
PowerPoint slide
Figure11.
(Color online) (a) Photographic image of graphene-based triboelectric touch sensor mounted on the wrist (top) and the spatial mapping image of current modulated by two-finger touch[122]. (b) Photograph of the conformal TENGs attached on the palm (top) and the bottom panel shows optical images of the self-powered contact sensors with contact to the different number of fingers and corresponding raw data[123]. (c) The stretchability of graphene is shown (upper) and the output voltage from GTNGs with various numbers of graphene layers under a vertical compressive force, with the inset which shows a captured image of three LED arrays that are simultaneously lit up by the power output generated from the GTNG[124].
The above-summarized literatures report that graphene indeed shows mechanical properties that fit the requirement for flexible NGs. ITO can be a conventional choice of material as a transparent conductive electrode in NGs. However, the poor compatibility in repeated bending/stretching makes it inferior to be a part of flexible NGs. On the other hand, the intrinsic elastic limit of graphene was demonstrated to bear up to a maximum value of 6%, mechanically much superior to ITO, along with its comparable transparency and conductivity, which have been realized as the best option for flexible NGs.
4.
Conclusions and perspectives
In the last decade, remarkable progress has been made in the field of graphene-based flexible and wearable electronics demonstrating a variety of applications such as high-speed FETs, touch panels/displays, LEDs, photo-detectors, photovoltaic cells, biomedical sensors, and energy-harvesting devices. The outstanding mechanical and unique electronic/optical properties of graphene make it the most explored material in this field. With continuous research and development, many new strategies are emerging towards the practical implementation of graphene-based flexible devices. It is believed that the smart applications of graphene systems with more functionality are expected to emerge in the near future, particularly in flexible and implantable bioelectronic devices to treat/monitor various diseases using electrical stimuli and also other bio-medical applications. Graphene as a basic building block of an implantable biomedical device fulfills most of the strict requirements such as low dimensionality, flexibility, stability in physiological conditions, and particularly being able to provide reliable electrical performance for years. Here, we reviewed the recent progress in graphene-based flexible and wearable devices and discussed where graphene-based research is progressing. This review is intended to give an overview of different interest areas, and discusses a few applications in this new and exciting field. However, it should be also noted that there are a number of other potential electronics applications of graphene. For those looking for a more in-depth explanation about the electronic performance of a specific class of graphene electronics or a particular electronic application, the reader can follow the work of other frontiers and renowned research groups. The considerable progress has already been made and the extensive research outcomes signify the strong potential of graphene in flexible/wearable devices along-with pointing out several challenging issues. The major issues that need to be addressed for commercialization of graphene-based wearable/implantable electronic devices include a systematic production approach for high-quality large area graphene with uniform and reliable electrical and mechanical properties, and the development of a superficial transfer technique providing graphene without defect generation. Innovative breakthroughs with ongoing fundamental research of graphene synthesis and transfer have provided a path towards its mass and low-cost production for industrial applications. Many start-up companies, as well as existing industrial enterprises, have thus started to produce graphene on a scale of thousands of square meters per year. Though the graphene industry is still in its early stages, very significant progress in large-scale production and certain industrial applications has already been realized.