1.
Introduction
During the past few years, flexible optoelectronics which can conform to curvilinear surfaces and endure mechanical deformations have aroused a great deal of interest, because of their potential to be applied in smart and wearable devices, such as flexible displays[1–5], highly sensitive sensors[6], flexible solar cells[7–10], transistors[11], artificial skins[12], human-activity monitoring[13, 14], and personal healthcare[15–17]. With flexibility or even stretchability, these optoelectronics guarantee their normal working state when they are attached to the human body and are even folded with motion, which provides excellent convenience. Regardless of the emergence of flexible or stretchable optoelectronics, three challenges are still remaining. Firstly, new types of flexible, stretchable or foldable substrates are in great demand. Mechanical flexibility or stretchability is strongly dependent on the substrates, which need to possess low oxygen and moisture penetrability, high optical transparency, low surface roughness and excellent bending capability. The second main challenge for flexible optoelectronic devices is caused by the restriction of transparent conductive electrodes (TCEs). To replace the brittle indium-tin-oxide (ITO) electrodes, graphene[18–20], metal nanowires[21–25], metallic grids[1, 26–29], and dielectric-metal-dielectric (DMD)[30, 31] electrodes are explored and fully characterized. Besides flexible substrates and TCEs, the design of novel device architectures is another crucial point for optimizing the device performance. It has been demonstrated that the trapped light in the substrate and waveguide modes in the flexible displays can be efficiently extracted by introducing quasi-periodic nanostructures[32, 33], bio-inspired nanostructures[34, 35], light scattering layer[36, 37], and nanoparticles[38, 39]. Thus, the light out-coupling efficiency and external quantum efficiency (EQE) can be substantially increased. On the other hand, the light in-coupled into the solar cells can be increased with no influence on electrical properties via plasmonic backscattering layer[40] or microcavity configuration[41].
Herein, this review provides a detailed overview of recent developments of flexible optoelectronic devices. In the first section, we summarize the progress in flexible substrates, TCEs and light harvesting or outcoupling structures aiming at the abovementioned major challenges. Then, some applications of flexible optoelectronic devices in different fields are discussed. Finally, the conclusion and prospects are proposed to show the future of flexible optoelectronic devices.
2.
Flexible substrates
It is well known that ultrathin glass, metal foil and plastics[42] are the most widely used flexible substrate materials for conventional optoelectronics. Although the flexibility of the optoelectronic devices can be realized via these materials, there are still many disadvantages to use them as the substrates. For example, the metal foils with good mechanical flexibility are usually opaque, which is not suitable for bottom emission and dual-side emission in lighting or display applications. Also, the thin glass is easily broken during repeatable bending, and the application of polymeric materials is limited by the thermal expansion coefficient. To match the requirements of flexible devices and the characteristics of flexible substrates, novel materials which are light-weight, cost-effective and highly transparent, are emerging as alternative options. A plastic-paper hybrid substrate with high optical transmittance over 85% and haze over 90% in the broadband visible wavelength region performs well in both organic light-emitting diodes (OLEDs) and typical GaAs solar cells[43]. As shown in Fig. 1(a), a simple template transferring method was used to combine the plastic and paper to form an ultra-flat substrate with light coupling function.

class="figure_img" id="Figure1"/>
Download
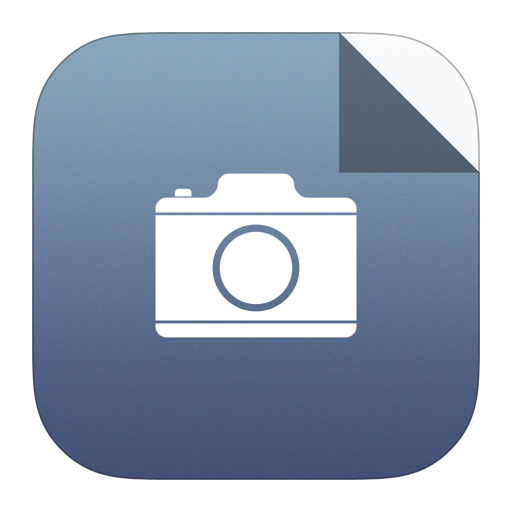
Larger image
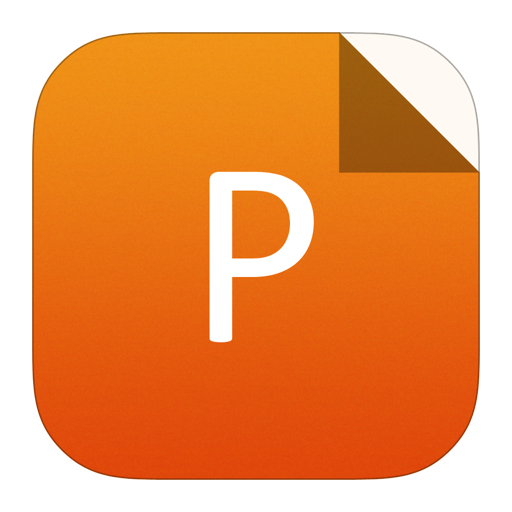
PowerPoint slide
Figure1.
(Color online) (a) Schematic showing the template polymer infiltration to combine the plastic and paper for a hybrid substrate, and the photo of flexible OLED based on the plastic-paper hybrid substrate under a bending state. Reproduced with permission from Ref. [43]. Copyright 2016, Royal Society of Chemistry. (b) The schematic image of the electrospinning epoxy backbone and spraying CNF fillers simultaneously, and dual-side emission of flexible OLED based on the hybrid film. Reproduced with permission from Ref. [49]. Copyright 2016, Macmillan Publishers Limited.
Cellulose nanofiber hybrid film, as a wood-based renewable material, is another hotspot of flexible substrates for use in flexible optoelectronic devices. The single cellulose fibril has a high Young’s modulus of 114 GPa[44] and high crystalline degree over 89%[45]. Incorporating the bacterial cellulose into the polymeric matrix, a nanocomposite film with low thermal expansion coefficient of 0.1 ppm/K[46] was fabricated. Manuspiya et al. reported the flexible substrates from bacterial cellulose (10–50 wt%) and poly-urethane based resin, which were utilized in a flexible OLED display with promising performance on light emission and bendability[47]. Yano et al. created nine types of matrix resin with cellulose nanofiber with good light transmittance and thermal expansion coefficient for the potential application as display substrates and flexible OLED with lower rates of ABPE10[48]. Park et al. used electrospinning and cellulose nanofiber fillers to form cellulose-epoxy hybrid materials as shown in Fig. 1(b)[49]. The cellulose-epoxy hybrid materials exhibited super mechanical thermal photo-patternable and optical characteristics for potential applications in transparent flexible OLEDs (right panel of Fig. 1(b)) and touch screen panels. Kippelen et al. fabricated efficient organic solar cells (OSCs) on recyclable cellulose nanocrystal substrates[50], exhibiting the cell performance up to the level of the referential devices on conventional polyethersulfone substrates. Cellulose nanofiber even exhibits suitable radio frequency properties for the integration of microwave-based optoelectronic devices[51], i.e., GaAs-based heterojunction bipolar transistors, Si-based complementary metal-oxide semiconductor digital devices and other essential components of integrated circuits. It is also worth noting that some stretchable devices have been fabricated on elastomeric substrates like photopolymer[52] and poly-dimethylsiloxane (PDMS)[53] for potential wearable applications.
3.
Transparent electrodes
For flexible or wearable optoelectronics, e.g., OLEDs, OSCs and touch panels, it is crucial that flexible TCEs must possess excellent optical, electrical and mechanical properties. In the past few years, the ITO conductor is the most commonly used TCE material for OLEDs and OSCs. Commercial ITO thin films usually possess an optical transmittance of over 80% in the visible range and sheet resistance (Rs) of < 20 Ω/□ [54]. However, the brittleness and scarcity of ITO limit the further developments of flexible optoelectronic devices. To discover the alternatives, various types of materials have been explored to replace ITO. In this review, we focus on five groups of TCE materials that have been proposed for organic optoelectronic devices, especially in OLEDs and OSCs, including conductive polymers, graphene-based electrodes, metallic nanowires electrodes, graphene-metallic nanowires hybrid electrodes and DMD electrodes[19–31, 55–66]. The representative key characteristics of these five groups are summarized in Table 1.
Type | Material | Transmittance (%) | Rs (Ω/□) | Flexibility | Applications | Ref. |
Conductive polymers | PDMS/PEDOT:PSS | > 90% | 81–122 | Flexible | OSC | [55] |
Acid treated PEDPT:PSS | > 90% in visible range | 95 | Flexible | OLED | [56] | |
UV-treated PET/PEDOT:PSS | 81% | 52.4 | Flexible | OLED & OSC | [57] | |
Ag@f-rGO/PEDOT:PSS | 88% | 88 | Flexible | – | [58] | |
Graphene- based electrodes | PET/graphene/Gra HIL | More than 85% in visible range | 30 | Flexible | OLED | [19] |
Graphene oxide/graphene | More than 88% in visible range | 268 | Flexible | OLED | [20] | |
PMMA/rGO/SWNT | 85% | 153 | Flexible | – | [59] | |
PET/graphene | ~90% in visible range | ~400 | Flexible | OSC | [60] | |
rGO-SWCNT hybrid (4 layers) | 65.8% @ 550 nm | 331 | Flexible | OSC | [61] | |
Metallic nanowires electrode | Embedded Ag network | > 87% | < 5 | Flexible | OLED | [1] |
PET/Ag NWs | 80% | 8 | Flexible | – | [21] | |
PDMS/Cu NWs | 70% @ 550 nm | 4.1 | Stretchable | – | [22] | |
PET/UV curable polymer/Ag NWs | 80.9% @ 550 nm | 8.2 | Flexible | OLED | [23] | |
PDMS/Au NWs | 92% @ 550 nm | 227 | Flexible | – | [24] | |
CuNi nanomesh | ~81% @ 550 nm | 7.5 | Flexible | OSC | [25] | |
PDMS/Ag NWs | ~88.5% @ 550 nm | 0.4 | Stretchable | Sensors | [28] | |
PET/Ag NWs | 85% | 33 | Flexible | Touch screen | [62] | |
PU/Ag NWs | > 80% | < 10 | Stretchable | LED integrated conductor | [63] | |
Graphene- metallic nanowires hybrid electrode | PEDOT:PSS/Ag NWs/graphene | 71.21% @ 550 nm | 181.67 | Flexible | OLED | [64] |
Graphene/Ag NWs hybrid | 94% in visible range | ~33 | Stretchable | Transistor & displays | [65] | |
Graphene/Ag NWs hybrid | 91% in visible range | 1 | Stretchable | Transistor | [66] | |
DMD electrode | ZnS/Ag/WO3 | > 80% | 6.0 | Flexible | OLED | [30] |
PET/ZnS/Ag/MoO3 | 74.22% @ 550 nm | 9.74 | Flexible | OLED | [31] |
Table1.
Summary of the reported transparent conductive electrodes.
Table options
-->
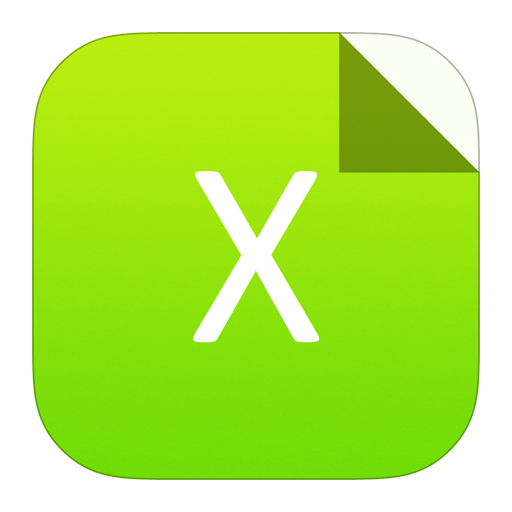
Download as CSV
Type | Material | Transmittance (%) | Rs (Ω/□) | Flexibility | Applications | Ref. |
Conductive polymers | PDMS/PEDOT:PSS | > 90% | 81–122 | Flexible | OSC | [55] |
Acid treated PEDPT:PSS | > 90% in visible range | 95 | Flexible | OLED | [56] | |
UV-treated PET/PEDOT:PSS | 81% | 52.4 | Flexible | OLED & OSC | [57] | |
Ag@f-rGO/PEDOT:PSS | 88% | 88 | Flexible | – | [58] | |
Graphene- based electrodes | PET/graphene/Gra HIL | More than 85% in visible range | 30 | Flexible | OLED | [19] |
Graphene oxide/graphene | More than 88% in visible range | 268 | Flexible | OLED | [20] | |
PMMA/rGO/SWNT | 85% | 153 | Flexible | – | [59] | |
PET/graphene | ~90% in visible range | ~400 | Flexible | OSC | [60] | |
rGO-SWCNT hybrid (4 layers) | 65.8% @ 550 nm | 331 | Flexible | OSC | [61] | |
Metallic nanowires electrode | Embedded Ag network | > 87% | < 5 | Flexible | OLED | [1] |
PET/Ag NWs | 80% | 8 | Flexible | – | [21] | |
PDMS/Cu NWs | 70% @ 550 nm | 4.1 | Stretchable | – | [22] | |
PET/UV curable polymer/Ag NWs | 80.9% @ 550 nm | 8.2 | Flexible | OLED | [23] | |
PDMS/Au NWs | 92% @ 550 nm | 227 | Flexible | – | [24] | |
CuNi nanomesh | ~81% @ 550 nm | 7.5 | Flexible | OSC | [25] | |
PDMS/Ag NWs | ~88.5% @ 550 nm | 0.4 | Stretchable | Sensors | [28] | |
PET/Ag NWs | 85% | 33 | Flexible | Touch screen | [62] | |
PU/Ag NWs | > 80% | < 10 | Stretchable | LED integrated conductor | [63] | |
Graphene- metallic nanowires hybrid electrode | PEDOT:PSS/Ag NWs/graphene | 71.21% @ 550 nm | 181.67 | Flexible | OLED | [64] |
Graphene/Ag NWs hybrid | 94% in visible range | ~33 | Stretchable | Transistor & displays | [65] | |
Graphene/Ag NWs hybrid | 91% in visible range | 1 | Stretchable | Transistor | [66] | |
DMD electrode | ZnS/Ag/WO3 | > 80% | 6.0 | Flexible | OLED | [30] |
PET/ZnS/Ag/MoO3 | 74.22% @ 550 nm | 9.74 | Flexible | OLED | [31] |
3.1
Graphene-based electrodes
Graphene is a one-atomic-layer-thick two-dimensional carbon material with hexagonal lattice structure, which possesses outstanding electrical, optical, mechanical and thermal properties. For example, the intrinsic mobility of graphene can reach 200 000 cm2V?1s?1 with the proper substrate at room temperature[67]. The transmittance of single-layer graphene is over 97% in the visible range and each additional monolayer decreases the total transmittance by 3%[68]. The Young Modulus of graphene is as high as 1 TPa[69], and its bending radius can be down to the millimeter scale without degradation[70]. These excellent properties promote graphene as a good candidate for TCEs. Various methods to synthesize graphene have been developed, such as mechanical exfoliation, chemically reduced graphene oxide (rGO), thermal decomposition of SiC and chemical vapor deposition (CVD). The multi-layer graphene (MLG) was utilized directly as TCE to substitute ITO in the early works[71, 72]. To further improve the device performance, various methods have been developed to modify the graphene for enhancing the hole injection properties. For instance, Li et al. dipped the single-layer graphene (SLG) in triethyloxonium hexachloroantimonate (OA)/dichloroethene solution for the p-type doping, which could increase the work function of doped graphene up to 5.1 eV[73]. Then, poly(3,4-ethylenedioxythiophene):poly (styrene sulfonate) (PEDOT:PSS) was spin-coated on SLG to ensure the surface wettability, and transition metal oxide MoO3 was introduced to further reduce the hole-injection barrier. As shown in Fig. 2(a), the white OLED based on the MoO3/PEDOT/SLG stack electrode exhibited an EQE of 17% at 3000 cd/m2 without the light extraction structure.

class="figure_img" id="Figure2"/>
Download
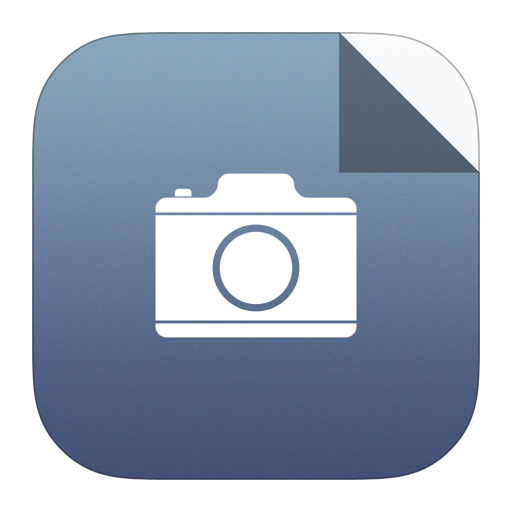
Larger image
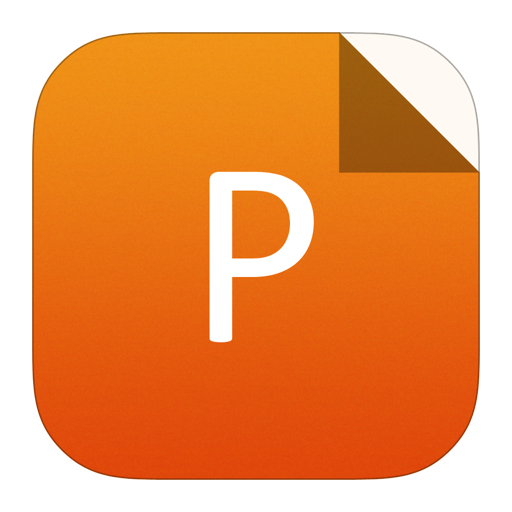
PowerPoint slide
Figure2.
(Color online) (a) Device structure (left), photos (middle) and EQE characteristics (right) of phosphorescent white OLED on monolayer graphene. Reproduced with permission from Ref. [73]. Copyright 2013, Macmillan Publishers Limited. (b) Schematic illustration of self-organized Gra HIL (left), device photo (middle) and luminous efficiency (right) of OLED on four-layered graphene anode doped with HNO3 (4L-G-HNO3). Reproduced with permission from Ref. [19]. Copyright 2012, Macmillan Publishers Limited. (c) The device structure (left), luminance photo (middle), and EQE performances (right) of OLED on TiO2/graphene/Gra HIL anode. Reproduced with permission from Ref. [3]. Copyright 2016, Macmillan Publishers Limited.
Another modification method was proposed by Han et al.[19], in which the HNO3 or AuCl3 was used as p-type dopant to reduce the Rs of graphene, and perfluorinated ionomers (PFI) were mixed into PEDOT:PSS to form a self-built-in-gradient hole-injection layer (Gra-HIL) for the energy level tuning. The phosphorescent green OLED device exhibited a maximum luminance efficiency of 102.7 lm/W (Fig. 2(b)). Lee et al. made a concerted graphene electrode with a sandwich structure, where the single-layer graphene was sandwiched by a high-index TiO2 layer for enhancing the cavity resonance and a low-index PEDOT:PSS/PFI Gra-HIL for reducing the surface plasmon polariton (SPP) loss (Fig. 2(c))[3]. The resulting OLED performed a high EQE of 40.8%. Regardless of the successful application of graphene films as TCEs in optoelectronic devices, the production and transfer of high-quality large-scale graphene with uniform properties are still challenging for the commercialization. It is necessary to extend the excellent graphene-based TCEs with reproducibility.
3.2
Metallic nanowires electrodes
Metallic nanowires (NW) are another kind of promising TCE materials that are capable of replacing ITO for flexible devices, since metallic NWs possess outstanding electrical conductivity, optical transparency and bending durability against mechanical force. Metallic NWs can be dispersed uniformly into solutions and then coated to form large-scale TCEs through solution processes, such as spin-coating, Mayer rod coating, spray coating, ink-jet printing or drop casting. The production process is low-cost, environmentally-friendly and repeatable[21–28, 62–66]. However, the oxidation of metallic nanowires occurs easily, and the junctions between nanowires may induce high roughness and uneven Rs.
Ag NWs are the most widely used materials as TCEs due to their high conductivity. At present, the products of Ag NWs are available in the market, which are dissolved in water, alcohol or organic solvents with relative high aspect ratio (typically diameter of 20 to 40 nm, length above 20 μm)[54]. For example, Song et al. directly spin-coated Ag NWs on PET substrate to function as the TCE, showing a Rs of 10 Ω/sq and high transmittance around 95% at 550 nm[74]. However, there are still two aspects that need to be solved for the TCEs based on randomly distributed Ag NWs: (1) the partial high contact resistance between Ag NW junctions and (2) the low adhesion between Ag NWs and flexible substrates. To reduce the junction resistance, many treatments have been developed, including thermal welding[75], large pulsed electron beam welding[76], mechanical force pressing[77], and ion-gel bonding[78]. Several representative methods are followed. One way is to form the hybrid structure by introducing the semiconducting oxide, e.g., indium-zinc-oxide (IZO) or ITO. For example, Yun et al. fabricated an Ag NWs/IZO /PEDOT:PSS hybrid electrode through the method as shown in Fig. 3(a), where the IZO not only prevents the beneath layers of Ag NWs from oxidation but also fixes them tightly to the substrate[79]. Similarly, Kim et al. sputtered the ITO on the Ag NWs to form the TCE with a Rs around 26.07 Ω/sq and transmittance of 83.62% at 550 nm[80]. The second way is to integrate an additional metallic layer. For example, Cui et al. coated the Ag NW junctions with Au (Fig. 3(b)), resulting in the substantial decrease in Rs from several thousands to the several tens level[21]. Triambulo et al. spin-coated Ag nanoparticles (NPs) as a welding medium to bond the junctions, and the resulted TCE exhibited the superior mechanical flexibility[81]. Similarly, Zhang et al. dipped the Ag NWs TCE into the mixture of AgBr and NaVc and then into Na2S2O3 solution, through which the Ag NPs grew spontaneously on the TCE[82]. Tao et al. combined the Ag grid with Ag NWs to form a TCE[83], where the Ag grid connected the gap between Ag NWs and the overall Rs was reduced. The third way is to use the mechanical press to fuse nanowires and reduce the contact resistance. For example, Lin et al. fabricated an orthogonally woven Ag NWs TCE by applying shear force between the Meyer rod and silver nanowires solution to repeat the bar-blading twice with orthogonal movement directions and then treating with sol-gel ZnO[84]. The OLEDs and OSCs based on the orthogonally woven Ag NWs TCE show better performances than conventional devices.

class="figure_img" id="Figure3"/>
Download
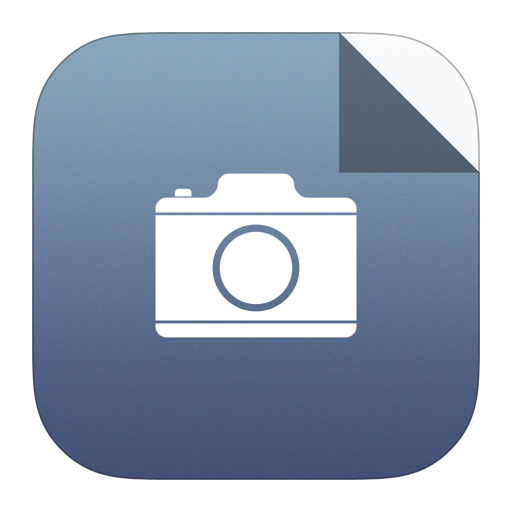
Larger image
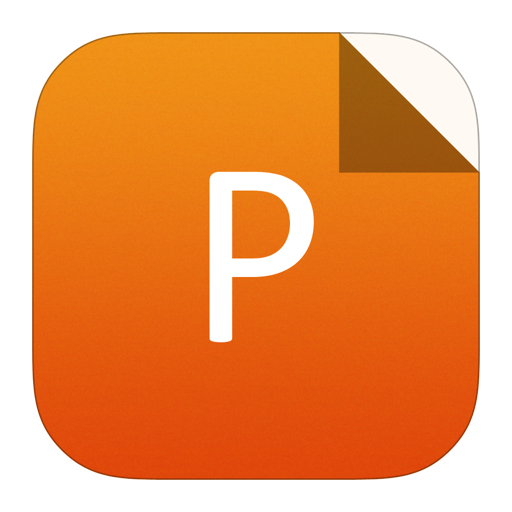
PowerPoint slide
Figure3.
(Color online) (a) An illustration of the fabrication process of the hybrid electrode and device, and the SEM images of pristine Ag NWs, IZO-coated Ag NWs, and PEDOT:PSS coating on Ag NWs-IZO. Reproduced with permission from Ref. [79]. Copyright 2016, Macmillan Publishers Limited. (b) Explanation of Au-Ag alloy network formation during the solution procedure. Reproduced with permission from Ref. [21]. Copyright 2010, American Chemical Society.
To handle the adhesion problem of Ag NWs on flexible substrates, many methods have been explored as follows. Sun et al. buried the Ag NWs into PEDOT:PSS and transferred them onto photopolymer NOA63[85], leading to an ultrasmooth TCE for the enhanced device performance. Duan et al. also used NOA63 to stick Ag NWs, which could be peeled off from the rigid Si substrate after UV exposure[86]. Xiong et al. produced the networks of Ag NWs through the vacuum-filtration process as shown in Fig. 4(a), in which the Ag NWs were welded via silver-ammonia and glucose mixed solution and then conductive ion-gel was introduced as the protective layer[78]. Ok et al. formed a hybrid TCE based on Ag NWs via an inverted film process (Fig. 4(b)) by employing Ag NWs into colorless polyimide (cPI), showing a transparency above 80% in the visible range and Rs of 8 Ω/sq[4]. Liu et al. controlled the adhesion of the Ag NWs through changing the hydrophobic and hydrophilic properties on the PDMS surface to form the auto-patterned Ag NWs/PEDOT: PSS on epoxy, which can be peeled off from the PDMS (Fig. 4(c))[87]. Zhou et al. embedded Ag ink into UV resin with a hexagonal pattern to form the TCE with a high transmittance above 87% in the visible range and a low Rs below 5 Ω/sq[1]. Jin et al. also embedded a Ag grid into the photopolymer (Fig. 4(d))[88], leading to the decrease in the root-mean-squared (RMS) roughness below 1.0 nm after embedding treatment and the tight stick of Ag NWs to the substrate. In contrast, Kang et al. built a photo-patternable TCE with embedded Ag NWs into photocurable polymer under selective UV exposure (Fig. 4(e)), in which the Ag NWs were tightly stuck to the substrate[23].

class="figure_img" id="Figure4"/>
Download
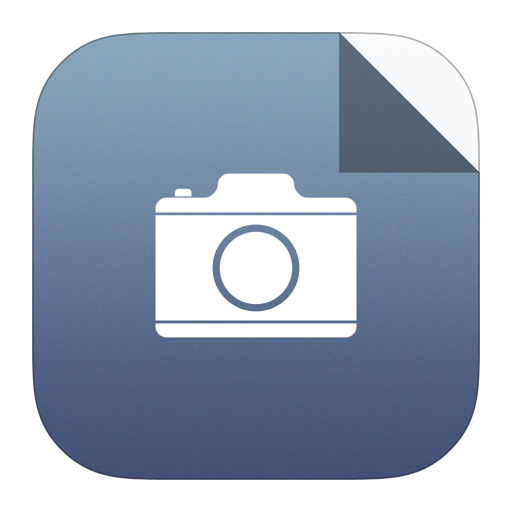
Larger image
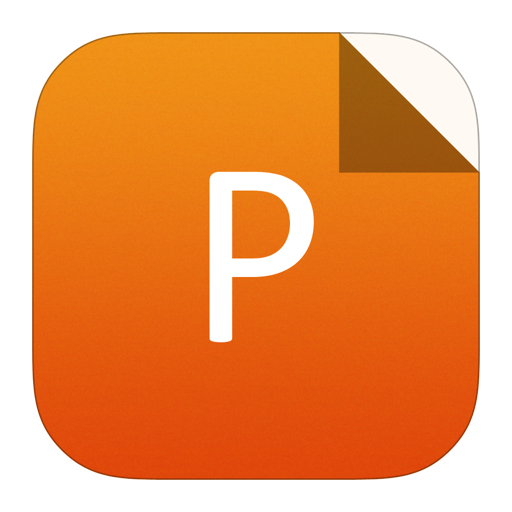
PowerPoint slide
Figure4.
(Color online) (a) The fabrication process of stable Ag NWs@ion-gel composite film. Reproduced with permission from Ref. [78]. Copyright 2016, Wiley-VCH. (b) The procedure and characterization results of Ag NWs embedded TCE. Reproduced with permission from Ref. [4]. Copyright 2015, Macmillan Publishers Limited. (c) The illustration of auto-patterned TCE. Reproduced with permission from Ref. 87. Copyright 2016, American Chemical Society. (d) The fabrication process of grid-embedded TCEs (ME-TCEs). Reproduced with permission from Ref. [88]. Copyright 2016, Macmillan Publishers Limited. (e) The fabrication steps of photo-patternable TCE. Reproduced with permission from Ref. [23]. Copyright 2016, American Chemical Society.
In addition to the use of Ag NWs, other metallic NWs such as Cu and Au have been extensively investigated for the fabrication of TCEs in optoelectronic devices. Hwang et al. synthesized ultralong Cu NWs with an average length of 92.5 μm and sprayed them on PDMS to form a TCE with a low Rs of 4.1 Ω/sq[22]. Wang et al. got the well-dispersed Cu NWs with a high aspect ratio via the hydrothermal process[89], and the TCE made of these Cu NWs performed well in conductivity, transmittance and flexibility after a wet treatment. Zhong et al. combined selective intense pulsed light (IPL) and roll-to-roll (R2R) wiping process to produce a precisely patterned Cu NWs electrode with a low Rs of 17.4 Ω/sq (Fig. 5(a))[90]. To induce the pure Cu NWs to contact with each other, Won et al. chose lactic acid to remove the surface oxide from Cu NWs on the substrate[91]. Kim et al. attained a cupronickel nanomesh electrode with Cu NWs via an UV adhesive-assisted transfer print process[25], which showed a high oxidation resistivity, low Rs of 7.5 Ω/sq and high optical transparency of 81% at 550 nm.
Gold provides excellent stability against chemical degradation and environmental oxidation compared to other metals. For example, Maurer et al. produced Au NWs-based TCE by sintering Au NWs into the imprinted hexagonal structure under stamping (Fig. 5(b))[24]. The resulting TCE possesses a low Rs of 29 Ω/sq and changeable transmittance by wire concentration and stamp geometry. Similarly, Gong et al. spread Au NWs at the air-water interface, resulting in the self-assemble of Au NWs into mesh-like bundles during the solvent evaporation[92]. Such a TCE is flexible, transferable and patternable, which shows potential applications in flexible or wearable optoelectronic devices.

class="figure_img" id="Figure5"/>
Download
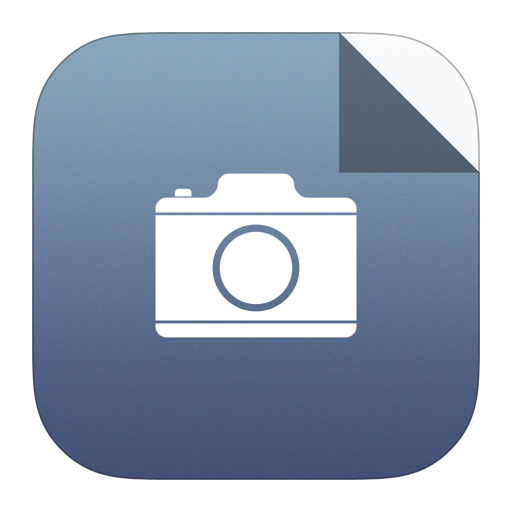
Larger image
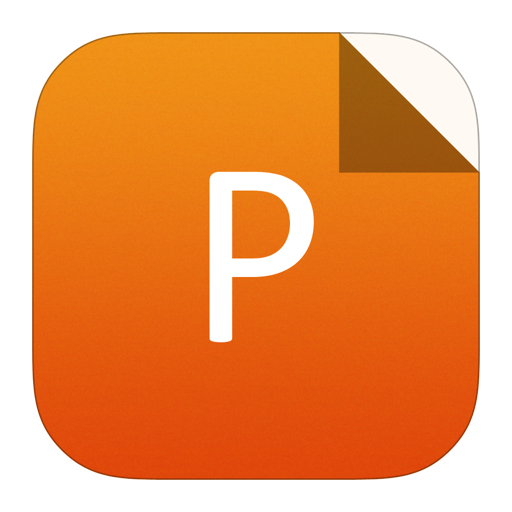
PowerPoint slide
Figure5.
(Color online) (a) Schematic illustration of the patterning method for flexible TCEs based on Cu NWs using a mask for selective intense pulsed light (IPL) irradiation, and the Cu NWs in the nonirradiated area can be clearly eliminated. Reproduced with permission from Ref. [90]. Copyright 2016, American Chemical Society. (b) Schematic diagram of the templated self-assembly of ultrathin Au NWs by the nanoimprinting process, and the diagrams below are detailed explanations of the bundling and sintering process. Reproduced with permission from Ref. [24]. Copyright 2016, American Chemical Society.
3.3
Graphene-metallic NWs hybrid electrode
To overcome the drawbacks of some existing transparent conductive materials, one promising method is to combine them into hybrid electrodes to improve the overall performance due to synergistic effects[93, 94]. For example, Dong et al. designed a graphene/Ag NWs/polymer hybrid electrode by integrating Ag NWs onto single-layer graphene with a hot press and covering a mixed polymer on top of the Ag NWs-graphene layer to prevent oxidation and corrosion[93], which exhibited a Rs of 8.06 Ω/sq and the transmittance of 88.3%. Similarly, Lee et al. constructed high-density Ag NWs on the CVD-grown graphene via van der Waals force to form a hybrid TCE for single-pixel displays and field-effect transistors (Fig. 6), which have shown outstanding optoelectronic performances, such as low Rs of 33 Ω/sq, high transmittance around 94% in the visible range, robustness against short circuits and oxidation, super flexibility and stretchability of 100% in tensile strain[65].

class="figure_img" id="Figure6"/>
Download
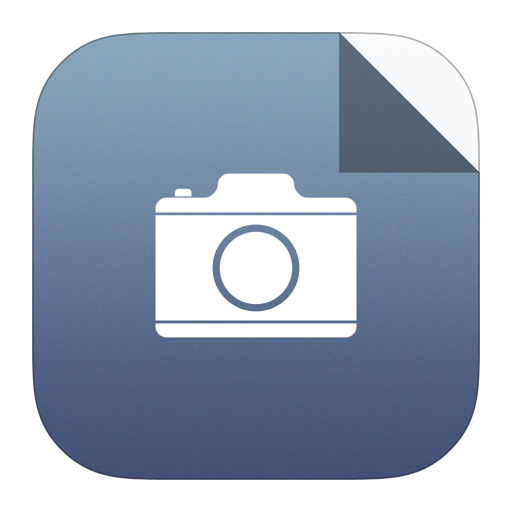
Larger image
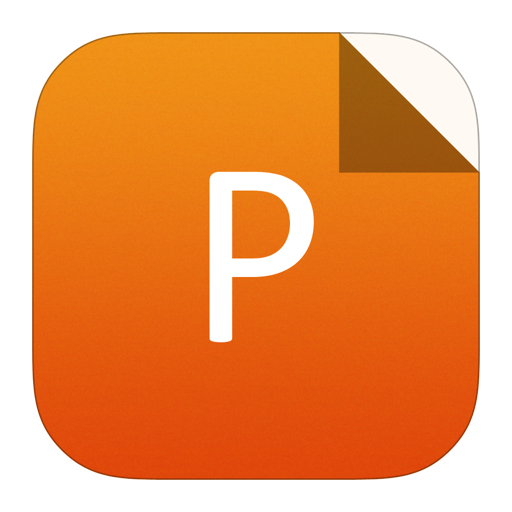
PowerPoint slide
Figure6.
(Color online) (a) Photograph of graphene-Ag NW hybrid TCE on PET substrate. Inset shows the SEM image of this hybrid. (b) Photos of the hybrid electrodes wrapped on cylindrical supports. (c) Schematic illustration of a single-pixel contact lens display using an inorganic light-emitting diode and the hybrid electrode. (d) Oxide semiconductor transistors with graphene?AgNW hybrid source/drain electrodes. Reproduced with permission from Ref. [65]. Copyright 2013, American Chemical Society.
3.4
Dielectric–metal–dielectric (DMD) electrode
DMD electrodes usually consist of an outer dielectric layer with high refractive index for optical coupling, a sandwiched semitransparent metal film for electrical conduction electrode, and an inner dielectric layer for carrier injection, e.g., ZnS/Ag/WO3[30], ZnS/Ag/ZnS[95], WO3/Ag/WO3[96], or ZnS/Ag/MoO3[97]. Kim et al. proposed the multilayer DMD electrodes with the structure of ZnS/Ag/MoO3 as anode and ZnS/Cs2CO3/Ag/ZnS as cathode, respectively, which were successfully used in flexible transparent OLEDs[31]. For an effective DMD-based TCEs, one major challenge is to form an ultrathin metal film with continuous film morphology, high optical transparency, and good electrical conductivity. The layer thickness of the sandwiched metal films in the DMD electrode should be as thin as possible for maximum optical transmission. However, the island-like growth mode of metal films will result in the formation of metallic NPs, and then cause the electrical disconnection and surface plasmonic (SP) loss at the metal/dielectric interface. For example, the deposited Ag film (<10 nm) tends to form discrete three-dimensional islands in the Volmer-Weber growth style with an isolated granular morphology. Although the growth of a thicker metal film can avoid the abovementioned problem, strong optical microcavity effects are inevitable, leading to spectral distortion of angle-dependent emission characteristics and poor color stability. To alleviate the film homogeneity problem for the ultrathin metal films, a sophisticated strategy has been developed by using a nucleation-inducing seed layer and the metal co-deposition effect[34]. For example, Ou et al. reported the thermal co-evaporation of an 8 nm-thick Ca:Ag alloy film onto a 1 nm-thick aluminum (Al) seed layer (Fig. 7), leading to the optimized film homogeneity of this Ca:Ag alloy ultrathin layer[98].

class="figure_img" id="Figure7"/>
Download
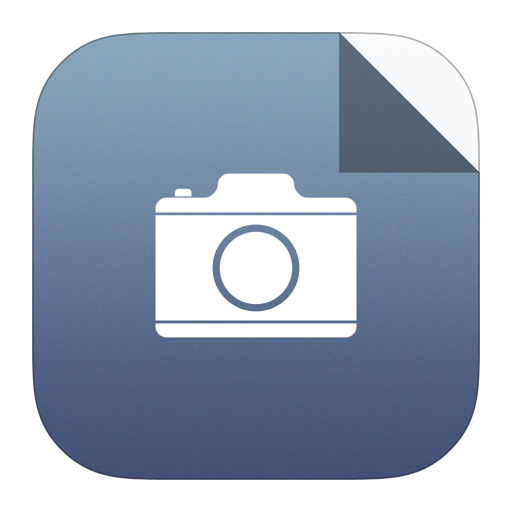
Larger image
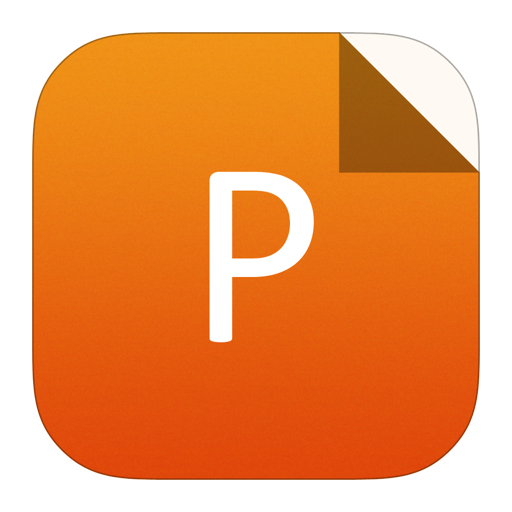
PowerPoint slide
Figure7.
(Color online) Schematic and SEM images of the nucleation-induced ultrathin metal film growth of (a) the Ca:Ag alloy with a metal seed layer and (b) pure Ag without the seed layer. Reproduced with permission from Ref. [98]. Copyright 2016, Optical Society of America.
4.
Light management
Due to the application of phosphorescent and thermal activated delayed fluorescent (TADF) materials for emitting components, the internal quantum efficiency (IQE) has reached nearly 100%[99–102]. However, the large differences between the refractive indices of organic films/TCEs (n1 ≈ 1.7–2.1), flexible substrates (n2 ≈ 1.5–1.7) and air (n3 = 1.0) cause severe waveguide loss at the organic/TCEs-substrates (waveguide mode), and total internal reflection (TIR) at the substrates/air interfaces (substrate mode)[103–106]. In addition, SPP loss occurs at the surface of the back metal electrode, which confines a fair amount of emitted photons. As a result, the ultimate EQE is typically around 20–30% for traditional planar OLEDs. To achieve high performance of flexible OLEDs, a light extraction technique has to be applied to out-couple the internal light to boost EQE for the relationship given by EQE = IQE ×ηext, where the ηext is the light extraction efficiency. During the last decade, various methods have been explored to enhance ηext, such as roughing the substrate surface, shaped substrate technique, micro-lens arrays, microcavity, two-dimensional photonic crystals, and nano-patterned and nano-porous films[107]. While few of them have been applied on flexible OLEDs, more efficient and convenient light-coupling techniques are highly desired on flexible devices due to their great potential in perspective display and illumination fields.
On the other hand, the OSC technique has been intensively developed as the new-generation renewable and clean energy source due to the compatibility to low-cost and large-area roll-to-roll fabrication process, which also holds great potential in wearable and portable energy storage devices[8, 108, 109]. To solve the incommensurate length scales of light absorption length (~100 nm) and exciton diffusion length (~10 nm), bulk hetero-junction (BHJ) structure has been conducted in OSCs to compensate the large length-scale mismatch. However, the power conversion efficiency (PCE) of OSCs remains low on account of the obstacle imposing thickness limitations on the photoactive layer while carriers travel through the distorted BHJ structure[110, 111]. Therefore, light management schemes such as surface plasmon, anti-reflection coatings, photonic crystal patterns, and micro-cavity are indispensable to enhance the light absorption in OSCs. In the next content, several state-of-the-art light management schemes in OLEDs and OSCs will be discussed.
4.1
Light extraction for OLEDs
4.1.1
Quasi-periodic nanostructures
For the light extraction in OLEDs, the use of periodic structures will cause severe spectral shift and color dependence on the viewing angle. One popular light extraction technique for OLEDs is the utilization of quasi-periodic nanostructures, which can result in the wavelength- and angle-independent out-coupling enhancement[106]. Compared to periodic grating structures, the quasi-periodic nanostructures can broaden the periodicity and randomize the emission directionality, resulting in the superiority of color stability over the visible wavelength range for wide viewing angles (Fig. 8(a))[106]. This method has been successfully applied to OLEDs. For example, Ou et al. achieved highly power-efficient white OLEDs by combining the deterministic aperiodic nanostructures (DANs) via a soft nanoimprinting lithography (SNIL) method with a PDMS mold for broadband quasi-omnidirectional light extraction and a multilayer energy cascade structure for efficient photon generation (Fig. 8(b))[112]. The EQE and power efficiency at 1000 cd/m2 of white OLEDs based on this device architecture are 54.6% and 123.4 lm/W, respectively, which are over two times that of the conventional planar device. Such a light extraction scheme has also been applied to tandem-structure OLEDs with remarkable efficiency improvement, color spatial uniformity as well as operational stability[33]. Using the same method, Xiang et al. reported that the combination of built-in Ag network TCE and quasi-periodic nanostructures could greatly improve the power efficiency values of red, green, blue and white flexible OLEDs to 47, 49, 56, 52 lm/W by releasing the confined light in the substrate mode (Fig. 8(c))[32]. Based on the same SNIL method with a perfluoropolyether (PFPE) mold, the quasi-periodic nanostructures were integrated into the DMD anode of flexible OLED, which could effectively eliminate the microcavity effect and enable the broadband and angle-independent out-coupling enhancement of waveguide light with minimized SPP loss (Fig. 8(d))[34]. Correspondingly, a highly efficient flexible OLED was achieved with an EQE of 47.2% and a power efficiency of 112.4 lm/W, respectively[34].

class="figure_img" id="Figure8"/>
Download
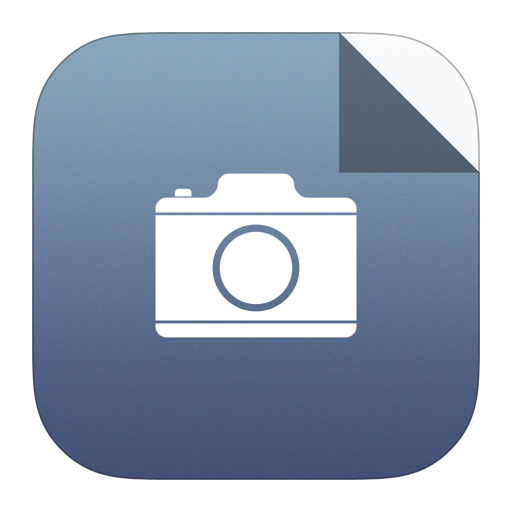
Larger image
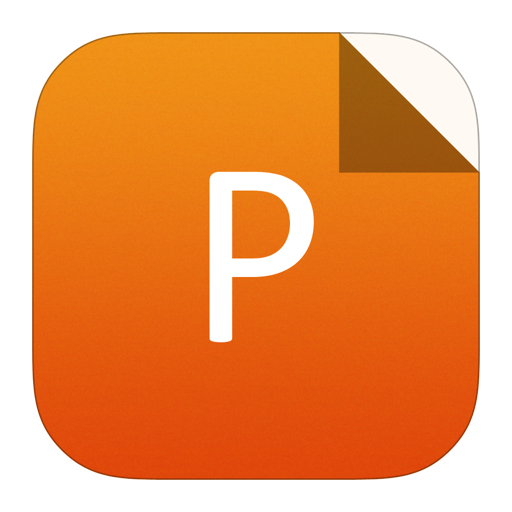
PowerPoint slide
Figure8.
(Color online) (a) Angular dependence of emission spectra for blue-, green-, and red-emission flexible OLEDs with periodic grating and quasi-periodic nanostructure. Reproduced with permission from Ref. [106]. Copyright 2015, Wiley-VCH. (b) Schematic of the white OLED patterned with DANs on both sides of the ITO glass substrate, and the device performance. Reproduced with permission from Ref. [112]. Copyright 2014, Wiley-VCH. (c) Schematic illustration of flexible OELDs using PET-AN/OC as a transparent conductive electrode. Reproduced with permission from Ref. 32. Copyright 2015, American Chemical Society. (d) Flexible OLED with nanostructured metal-dielectric composite electrode on a plastic substrate. Reproduced with permission from Ref. [34]. Copyright 2016, American Chemical Society.
4.1.2
Random scattering nanostructures
Recently, the introduction of a scattering layer into OLEDs nearby the ITO electrode has been developed for enhancing the out-coupling of internal light in the waveguide mode[113–115]. For example, randomly patterned structures, such as buckling structures and polymer spheres, have been reported to out-couple the internal light for angularly and spectrally independent enhancement in EQE[116–118]. Kim et al. developed a simple and scalable method with spontaneously formed metal oxide NPs randomly distributed on the substrate as a light scattering layer for internal light extraction of the waveguide mode in white OLEDs (Fig. 9(a)), resulting in the EQE enhancement with a factor of 1.7 and excellent color stability over broad viewing angles[119]. Regardless of the performance enhancement, the high-temperature processing in this method limits substrate availability. Huh et al. fabricated transparent OLEDs with improved efficiency and spectral viewing angle independency by using an internal random scattering layer cooperated with a high refractive index planarization layer between substrate and ITO layer (Fig. 9(b))[36]. Due to the efficient extraction of waveguide mode, a remarkable improvement of 40% and 46% in EQE and luminance efficiency has been achieved. Under the same consideration, Lee et al. melted the Ag NWs to obtain Ag nanodots as the light scattering structures[37], and the detailed illustration is displayed in Fig. 9(c). As a result, the EQE of the OLED with such an internal light out-coupling structure was improved by 49.1%. Furthermore, metal NPs have also been reported to excite the surface plasmonic effect, which would promote the radiative recombination of excitions to enhance the IQE by localized surface plasmon resonance induced by the metal NPs[105, 120–122].

class="figure_img" id="Figure9"/>
Download
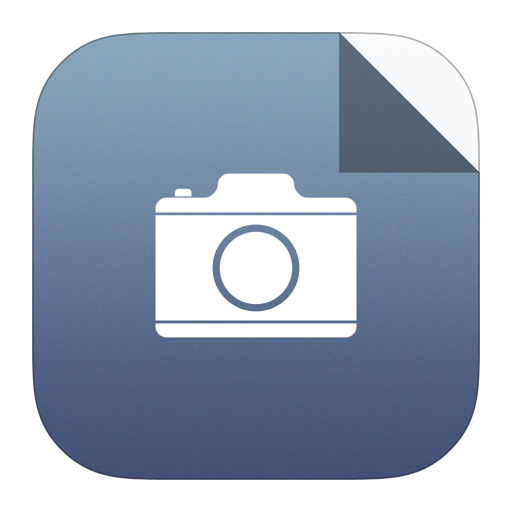
Larger image
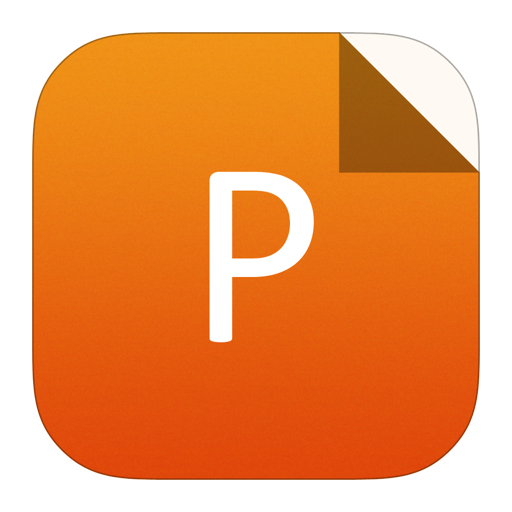
PowerPoint slide
Figure9.
(Color online) (a) Schematic process and SEM image for spontaneously formed SnO2-based scattering nanostructures. Reproduced with permission from Ref. [119]. Copyright 2015, Wiley-VCH. (b) Schematic structure of transparent OLEDs with an internal scattering layer, and SEM images of random scattering nanostructures. Reproduced with permission from Ref. [36]. Copyright 2014, Royal Society of Chemistry. (c) Schematic process flow of the SiOx-based internal scattering layer and the SEM images. Reproduced with permission from Ref. [37]. Copyright 2016, American Chemical Society.
4.1.3
High-refraction-index layer
As previously mentioned, most of the light generated in OLEDs is trapped within the device mainly due to the waveguide mode and substrate mode induced by the mismatch of the refractive indices of various materials. Consequently, the high-refractive-index substrates (n > 1.8) have been applied to substantially enhance the out-coupling of waveguide-mode light from the organic layers into the substrate [123–125]. Fig. 10(a) illustrates the light propagation originating from the organic emitter in OLEDs on different substrates. If the low-refractive-index substrate (nLsub ≈ 1.5) is replaced with a high-refractive-index one (nHsub = 1.7–1.8), the total internal reflection at the organic (norg = 1.7–1.9)/substrate interface can be minimized, so that part of the originally reflected light can be coupled into the substrate. For the fabrication of high-performance flexible OLEDs, Kim et al. proposed the use of an industrial-grade version of polyethylene naphthalate (PEN) (Fig. 10(b)), a relatively inexpensive commodity polymeric substrate, as a high-refractive-index plastic substrate to increase light extraction[124]. By combining the built-in scattering properties, the use of PEN substrates caused a significant enhancement in light extraction with power efficiency of 98.7 lm/W and EQE of 29.6%, respectively[124]. Unfortunately, most of the light-weight flexible plastic substrates used in flexible OLEDs have a relatively low refraction index (n ≈ 1.5)[126]. To overcome this obstacle, Wang et al. reported a multifunctional anode stack with a DMD structure consisting of an optical coupling layer of high-refractive-index Ta2O5, an electrically conductive layer of semitransparent Au thin film, and a hole-injection layer of MoO3 based on low-index plastic substrate without extra light extraction structures (Fig. 10(c))[127]. The fabricated green OLED on flexible plastic achieved a high EQE of ~40% at a brightness of 10 000 cd/m2, which could be further improved to 60% by using a lens on top of the device for reducing the amount of light trapped in the plastic substrate[127]. However, a microcavity effect occurs typically when such a DMD structure is used to enhance the extraction of the internal light between two metal electrodes. Its limitation is the narrow electroluminescence spectrum and strong angular dependence, which is unfavorable for general lighting and displays[128].

class="figure_img" id="Figure10"/>
Download
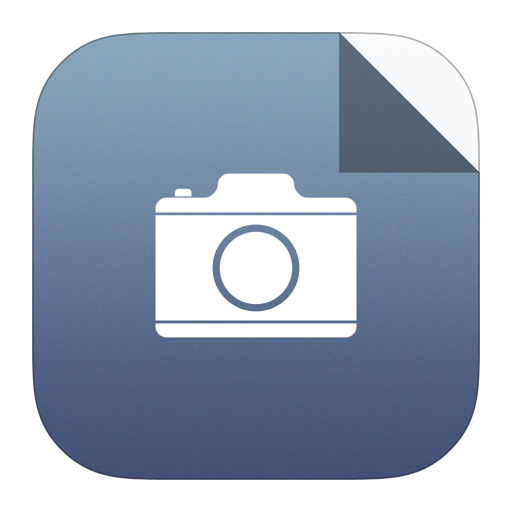
Larger image
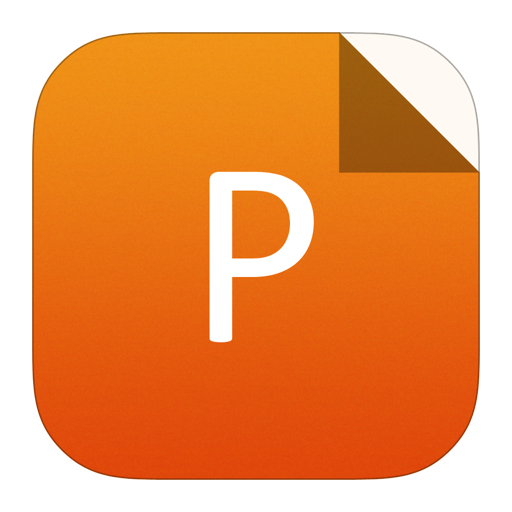
PowerPoint slide
Figure10.
(Color online) (a) Cross-sectional view of an OLED to illustrate the light propagation for released modes (solid line) and trapped modes (dashed line). Reproduced with permission from Ref. [123]. Copyright 2009, Macmillan Publishers Limited. (b) Schematic of OLEDs on industrial-grade PEN (I-PEN) substrate with high refractive index, showing the light propagation. Reproduced with permission from Ref. [124]. Copyright 2015, Wiley-VCH. (c) Schematic of flexible OLED device structure on plastic substrate with high-index stacked electrodes. Reproduced with permission from Ref. [127]. Copyright 2011, Macmillan Publishers Limited.
4.1.4
Bio-inspired nanostructures
In recent years, the biologically-inspired optical solutions mimicking natural structures have opened up new opportunities to design functional optical surfaces for lighting or display applications. The most widely used bio-inspired nanostructures are moth eye and firefly organs. Moth eye nanostructures belong to the quasi-periodic structure, as discussed in Section 4.1.1. Fireflies conduct their communication by flashing species-specific bioluminescence light in a dark environment, which calls for wide-angle lighting and effective luminescent emission[129–131]. Inspired by this phenomenon, Kim et al. investigated the hierarchical nanostructures on the lantern organ of fireflies[35], which were replicated using geometry-guided resist reflow, replica molding and PDMS oxidation (Fig. 11(a) and 11(b)). Similar to the cuticular hierarchical structures of a firefly lantern, the incorporation of this biologically inspired configuration in an OLED panel light enabled highly efficient light extraction and wide-angle illumination (Fig. 11(c)), and the EQE was improved by up to 61%[35]. These improvements are mainly attributed to the substantial suppression of TIR and Fresnel reflection induced by the asymmetric microstructures through index matching and subwavelength diffraction.

class="figure_img" id="Figure11"/>
Download
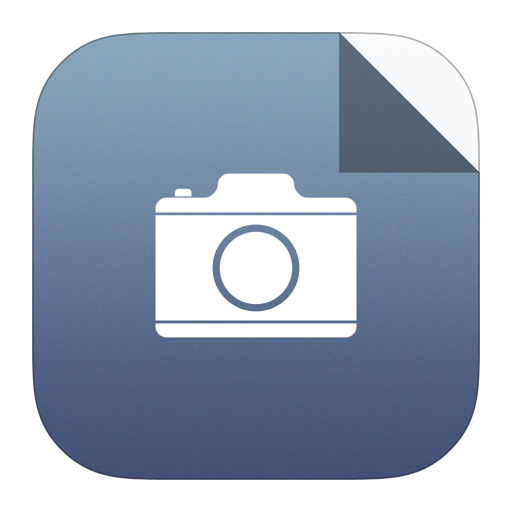
Larger image
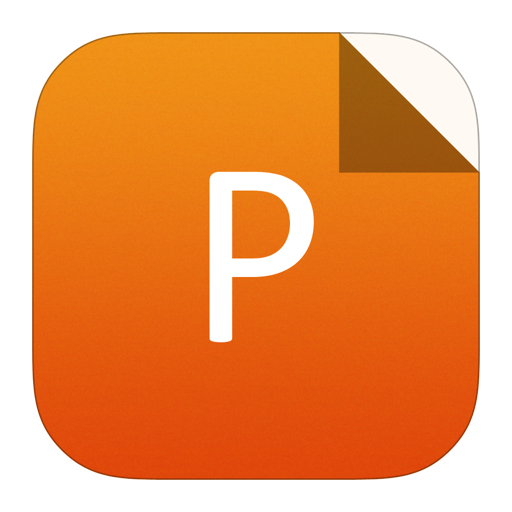
PowerPoint slide
Figure11.
(Color online) (a) Schematic configuration of a firefly lantern (left) and biologically inspired OLED device with hierarchical out-coupling structures on substrate (right). (b) A perspective SEM image of the hierarchical structure. (c) Luminescence appearance comparison with OLEDs in operation without and with structured surfaces. Reproduced with the permission from Ref. [35]. Copyright 2016, American Chemical Society.
4.2
Light trapping for OSCs
4.2.1
Surface plasmonic backscattering
One challenging factor for achieving highly efficient OSCs is to overcome the low absorption efficiency of the thin organic photoactive layers. Due to the low carrier mobility and short exciton diffusion length, the film thickness of typical organic photoactive layers is commonly limited to 100–200 nm for maximizing the extraction of photogenerated carriers and minimizing the charge recombination loss. However, such a thickness inevitably leads to low-efficient light absorption and carrier generation in OSCs[132–136]. In order to achieve highly efficient OSCs, light manipulation is indispensable to enhance light harvesting of ultrathin photoactive layers[137, 138]. The utilization of the surface plasmonic backscattering effect is beneficial to enhancing the light absorption of the photoactive layers by inserting metal NPs bet ween the back electrode and the photoactive layer[139–142]. Cheng et al. proposed a method for increasing light ab sorption by introducing a MoO3/Ag NPs/MoO3 sandwich structure as the hole collection layer for the rear anode (Fig. 12(a))[40]. The Ag NPs are fabricated with a self-assembly feature of thermally deposited Ag at sub-monolayer thickness on the top of the first MoO3 layer, which was then covered by a second MoO3 layer to form the MoO3/Ag NPs/MoO3 sandwich structure. The incorporation of Ag NPs structures will induce light backscattering and surface plasmonic coupling effects, which can effectively trap the incident light into an ultrathin photoactive layer by high-angle scattering and simultaneous triggering of localized surface plasmonic resonance (LSPR) by Ag NPs. Consequently, the short-circuit-current (Jsc) was highly improved, leading to the increase in PCE from 3.61% to 4.32%. In addition to inserting metal NPs, a continuous metal electrode with random nanostructures has also been used to enhance the light trapping in an organic photoactive layer. For instance, Niesen et al. introduced a patterned Ag rear electrode with nonperiodic structures by polystyrene (PS) beads nanosphere-templated colloidal lithography[143]. During this fabrication process (Fig. 12(b)), a short-range ordered nonclosed packed array of PS beads was drop-casted on the photoactive layer surface from an aqueous dispersion, which was then used as a shadow mask of nanoholes within the thermally evaporated MoO3 layer. After removing the PS beads, the Ag layer was deposited onto the MoO3 layer by filling these random nanoholes to form continuous nanostructures. As a result, the device efficiency was greatly enhanced by increasing the light absorption due to the LSPR effect and light scattering of this novel Ag electrode.

class="figure_img" id="Figure12"/>
Download
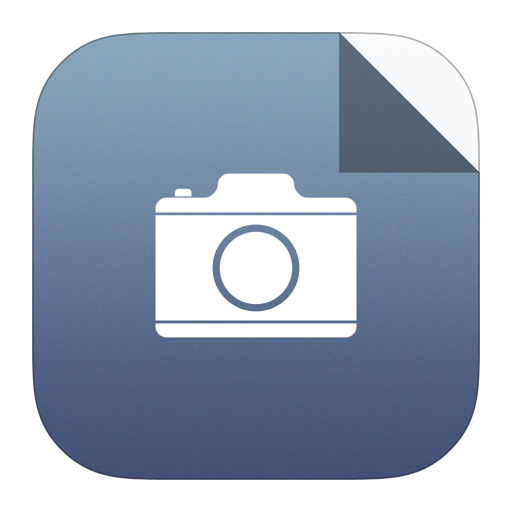
Larger image
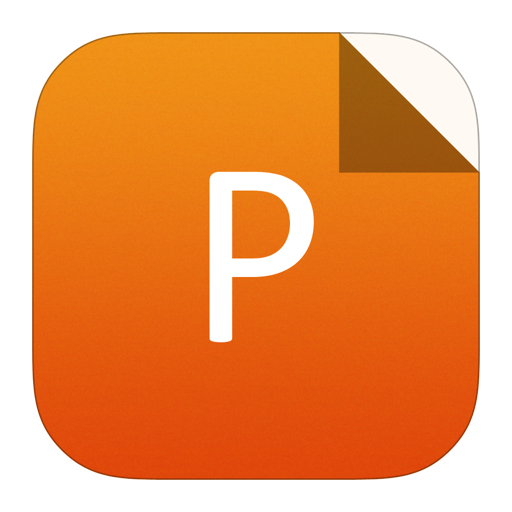
PowerPoint slide
Figure12.
(Color online) (a) Schematic device structure of an inverted OSC with Ag NPs embedded in the MoO3 hole collection layer, and the SEM image of Ag NPs thermally deposited on the MoO3 layer. Reproduced with permission from Ref. [40]. Copyright 2012, Royal Society of Chemistry. (b) Schematic fabrication process of nanoholes within the MoO3 layer in the nanostructured device (top) and the corresponding SEM images (bottom). Reproduced with permission from Ref. [143]. Copyright 2013, Wiley-VCH.
4.2.2
Antireflection coating
Due to the large difference between refractive indices of air and substrates, light becomes partially reflected when incident on the substrate surface, even for normal incidence[144]. In order to strengthen the light absorption of OSCs, antireflection (AR) coating is a consideration of great importance, which is applied to reduce light reflection and to promote more incident light to penetrate into OSCs by overcoming the intrinsic problem originating from the mismatched optical impedance at the air/substrate interface. Furthermore, self-cleaning AR coatings can guarantee the stable performance of OSCs by protecting devices from the accumulation of moisture and dust particles on the device surface in an ambient environment after a certain time[145]. To date, various designs of microstructures have been proposed to function as AR coatings to enhance the light trapping efficiency, among which microlens arrays (MLAs) have been widely applied[146, 147]. For example, Myers et al. used a self-assembled monolayer of PS microspheres as the template to form close-packed polymer MLAs with various diameters and spacings, and then molded a transparent polymer MLAs onto the light incident surface to boost light absorption by soft lithography (Fig. 13(a))[148]. Compared with the reference device, an efficiency enhancement of 15–60% was achieved due to the reduced surface reflection and increased light-absorption path in photoactive layer. Particularly, a wide-angle increment was achieved with this MLA-based AR scheme, which was ascribed to the efficient antireflection of high-angle incident light by the curved lens surface. Apart from MLA structures, Choet al. reported a paradigm combining the compound parabolic trapper with a V-groove textured surface based on the contrastive light trapping capacities among MLA, V-shaped configuration, and double parabolic trappers (Fig. 13(b))[149]. This light in-coupler configuration consisting of a one-dimensional compound parabolic concentrator (CPC) array that concentrated the incident light, and then the transmitted light was trapped by blocking mirrors between the entrances, resulting in the Jsc enhancement of 13.1%.

class="figure_img" id="Figure13"/>
Download
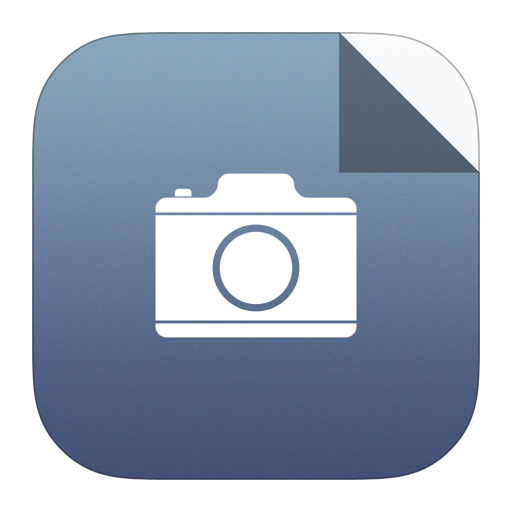
Larger image
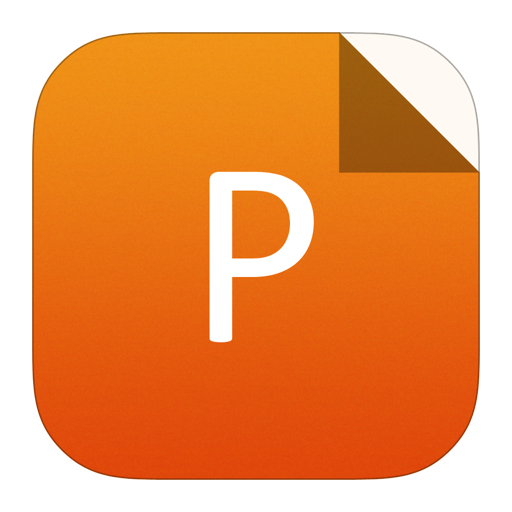
PowerPoint slide
Figure13.
(Color online) (a) Schematic illustration of light behavior with and without a microlens array (MLA) for OSCs, SEM image of a representative MLA, and Jsc performance of the device under angled incidence white light with and without a MLA. Reproduced with permission from Ref. [148]. Copyright 2012, Royal Society of Chemistry. (b) Schematic view of the compound parabolic trapper (CPT) consisting of a CPC array, blocking mirrors, spacer, and PV. Photo of the fabricated CPT. Normalized Jsc of OSCs along the cross-sectional incident angle variation. Reproduced with permission from Ref. [149]. Copyright 2015, Wiley-VCH.
4.2.3
Optical microcavity resonance
In addition to previously mentioned strategies, the integration of microcavity structures has been a popular scheme to enhance light trapping recently[150–152]. The term ‘microcavity’ refers to an optical resonator structure consisting of two planar surfaces with proper reflectivity, such as cathode and anode in OSCs. The penetrated light bounded inside a microcavity travels back and forth between these two surfaces until getting absorbed or leaking from the devices. As a result, light trapping is enhanced through multi-reflection, which can effectively extend the absorption path and reduce optical collection loss without using thick photoactive layers in OSCs. For the construction of the microcavity configuration, some high-refractive-index dielectric layers (e.g., MoO3, TeO2, and WO3) are usually utilized to manipulate the local electromagnetic field distribution in a microcavity cell[150, 153]. For instance, Chen et al. reported the OSC devices with an ITO-free microcavity structure as shown in Fig. 14(a), achieving a high PCE of 8.5% on both glass and flexible plastic substrates due to ~20% enhancement in Jsc relative to conventional ITO-based devices[41]. This significant performance enhancement has been attributed to efficient light harvest by the microcavity structure consisting of a transparent ultrathin Ag cathode and opaque Ag anode (Fig. 14(a)). On the other hand, tellurium oxide (TeO2) has been chosen as the top-capping and in-coupling layer due to its high refractive index (nTeO2 = 2.2) and convenient fabrication process via an evaporation process, which can theoretically reduce the total reflection of the ultrathin Ag electrode and strengthen the electric field within the organic photoactive layer[154]. Huang et al. demonstrated an ITO-free OSC device with 10.5% PCE composed of a structure of glass or PET/Ag/fullerene/BHJ/ MoO3/Ag/TeO2 based on microcavity configuration as shown in Fig. 14(b), containing a thick Ag cathode and a transparent ultrathin Ag anode with TeO2 as the capping layer[155]. In addition, an efficient large-area OSC device was realized by combining semitransparent grids and ultrathin Ag anode to improve the charge collection with reduced series resistance[155].

class="figure_img" id="Figure14"/>
Download
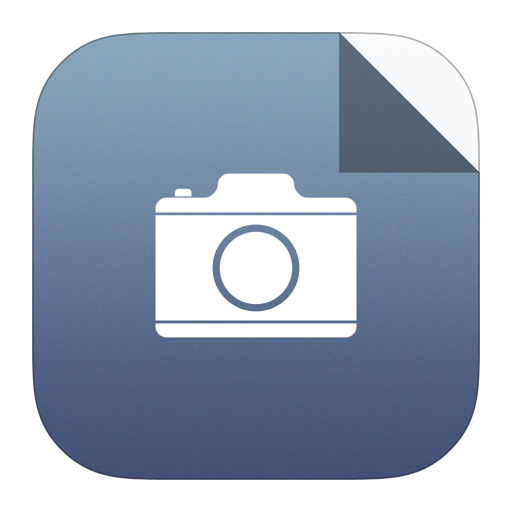
Larger image
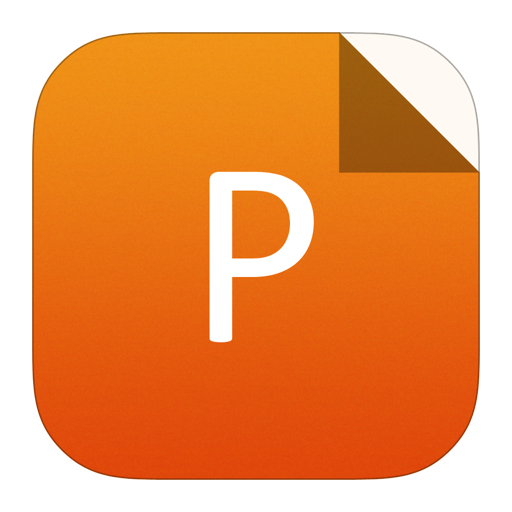
PowerPoint slide
Figure14.
(Color online) (a) Microcavity configuration using semitransparent Ag film and top-capping light in-coupling layer as the optical incident electrode. Reproduced with permission from Ref. [41]. Copyright 2014, Viley-VCH. (b) Micro-cavity embedded device configuration using a thick Ag film as cathode and an ultrathin Ag layer with a light in-coupling layer of TeO2 as anode on glass or PET. Reproduced with permission from Ref. [155]. Copyright 2015, Wiley-VCH.
5.
Applications
Due to the low-cost fabrication process, low-power consumption, and large-area capacity, flexible and wearable optoelectronic devices, such as OLEDs, OSCs, thin film transistors (TFTs) and sensors, have held huge potential and attracted large fundamental and technological interest[156, 157]. The progress of high-performance flexible and wearable optoelectronics largely relies on the developments of flexible substrates, flexible TCEs, and novel device architecture techniques. As discussed above, flexible OLEDs and OSCs have been extensively investigated based on novel flexible substrates with various TCEs and device configurations. In addition, the emerging field of electronics integration is calling for new requirements on electronic components. To directly integrate electronic components onto malleable objects such as biological organs, the ability to survive high mechanical deformation and a curvature of extremely small radius is inevitably required[158, 159]. In the following content, highly flexible or stretchable OLEDs, OSCs and TFTs are discussed as typical examples among electronics.
5.1
Stretchable OLEDs
Recently, stretchable OLEDs have attracted a great deal of interest because of their integration compatibility with various kinds of electronics[160, 161]. Compared to conventionally rigid or non-elastic OLEDs, stretchable OLEDs can be conformable to surfaces with complex topology like the human body, which ensures stretchable OLEDs have great potential in the field of wearable electronics. Up to now, the most popular method to achieve stretchable OLEDs is to fabricate devices with proper materials on highly flexible substrates that can be, to some extent, stretched and compressed without causing device failure[158]. For example, White et al. reported stretchable polymer-based OLEDs consisting of highly flexible conducting polymers, semiconducting polymers and thin metal layers with full function on an ultrathin (1.4 μm) PET substrate[5]. As shown in Fig. 15(a), an ultrathin (2 μm) stretchable OLED was fabricated, where the PET foil was primarily adhered to a PDMS-coated rigid glass support to ease processing. This stretchable OLED was demonstrated to withstand extreme deformation during crumpling and stretching, and had the stretch-compatibility to 100% tensile strain[5]. Different from 1D-stretchable OLEDs, Yin et al. demonstrated a stretchable OLED with high efficiency as well as excellent 2D stretchability[162]. The ultrathin OLED was firstly fabricated on a photopolymer (NOA 63)-coated Si substrate, which was then peeled off from the Si support. The fabricated OLED was adhered onto a pre-stretched elastic substrate, which was stretched uniaxially to 200% strain for 1D stretchable OLED and biaxially to 100% strain for 2D stretchable OLED at both diagonal directions (Fig. 15(b)). These stretchable OLEDs were formed with random buckles after releasing the elastic substrate. However, these stretchable OLEDs demonstrated ultraflexibility and mechanical stability during cyclic stretching measurements (Fig. 15(b)).

class="figure_img" id="Figure15"/>
Download
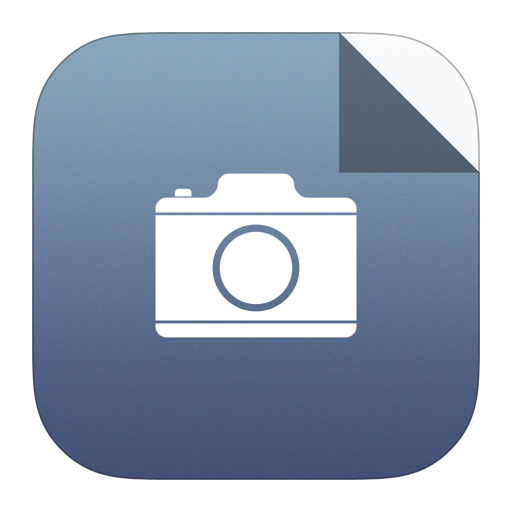
Larger image
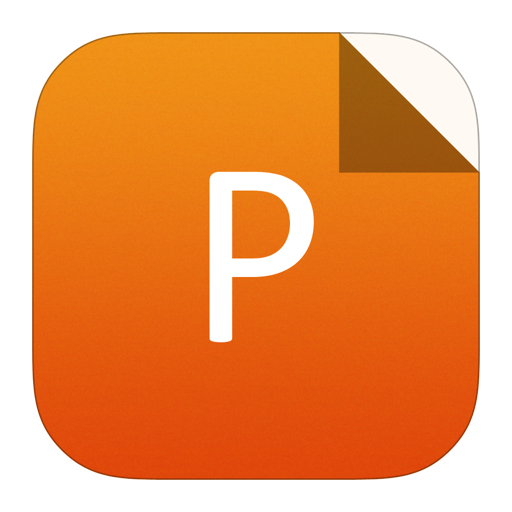
PowerPoint slide
Figure15.
(Color online) (a) Schematic of device structure and images of deformation tests of an ultrathin OLED operating during crumpling and stretching. Reproduced with the permission from Ref. [5]. Copyright 2013, Macmillan Publishers Limited. (b) Schematic of the fabrication process of 1D and 2D stretchable OLEDs, and images of 1D and 2D stretchable OLEDs operating at strains. Reproduced with permission from Ref. [162]. Copyright 2016, American Chemical Society.
5.2
Stretchable OSCs
Lightweight and mechanically resilient solar power sources, particularly OSCs, are attracting an increasing amount of attention for modern applications such as electronic textiles[163, 164]. To achieve highly flexible and stretchable OSCs, the first-line approach is the combination of ultrathin plastic substrate with high endurance of deformation and polymer functional layers. Correspondingly, Kaltenbrunner et al. demonstrated ultrathin, light, flexible and compliant OSCs fabricated on 1.4 μm-thick PET substrates where the whole device thickness was only 1.9 μm, which is even thinner than spider silk[165]. As shown in Fig. 16, all the materials were finely designed, including the highly flexible substrate, PEDOT:PSS, the photoactive layer of the poly (3-hexylthiophene) (P3HT):(6, 6)-phenyl-C61-butyric acid methyl ester (PCBM) bulk heterojunction, and the evaporated Ca/Ag metal electrodes, resulting in the extreme bending flexibility of this OSC. When this ultrathin OSC device was adhered onto a 100-μm pre-stretched elastomer (3M VHB 4905), it could be compressed by releasing the elastomer and stretched back to the pre-strain defined by the elastomer (bottom images in Fig. 16). In addition to polymers generally used as stretchable bottom electrodes, novel materials such as graphene and carbon nanotubes (CNTs) can be applied to serve as the electrodes as well. Different from the stretchable OSCs above, Kang et al. proposed a novel stretchable OSC structure containing microcell arrays linking each other using ITO electrodes[166]. During the fabrication process, the microcells were mechanically connected by thin polymer bridges with a serpentine shape on a sacrificial layer for support, which were then transferred by a PDMS stamp onto a pre-stretched PDMS substrate. Finally, by releasing the pre-strain PDMS substrate, a stretchable microcell array was achieved with non-coplanar layout in the serpentine shape, and the PCE of this OSC was reduced by only about 3% and 11% compared to its initial value when 6.5% and 18% tensile strain was applied. Recently, Nam et al. succeeded in fabricating a stretchable inorganic microcell array by using the same method of transfer-printing, demonstrating a practical route for the fabrication of stretchable solar cells for use in various soft electronic applications[167].

class="figure_img" id="Figure16"/>
Download
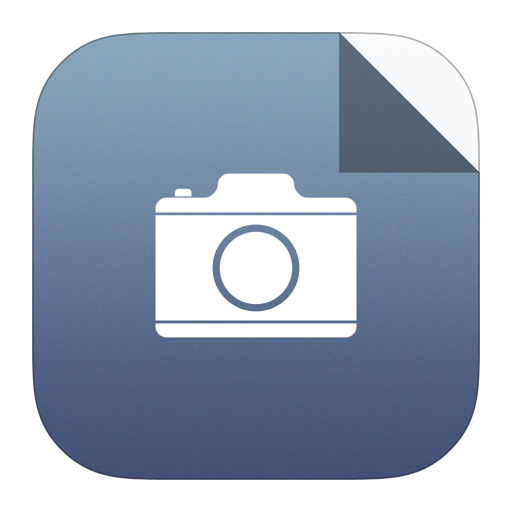
Larger image
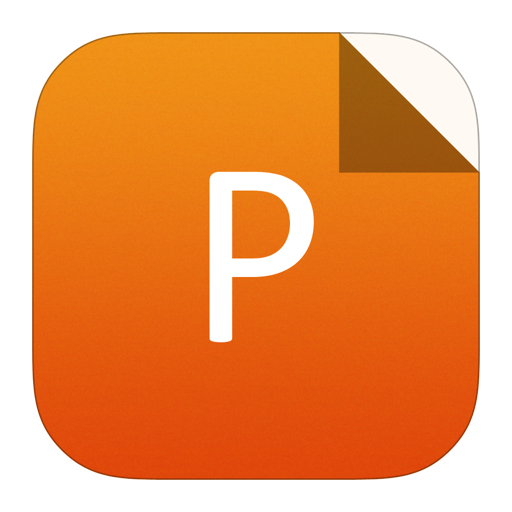
PowerPoint slide
Figure16.
(Color online) Schematic illustration of the stretchable OSC (top left), exhibiting extreme bending flexibility as demonstrated by wrapping it around a 35-μm-radius human hair (top right) and super stretchability under quasi-linear compression (bottom). Reproduced with permission from Ref. [165]. Copyright 2012, Macmillan Publishers Limited.
5.3
Flexible and stretchable TFTs
As a fundamental component of various electronics, TFTs have always been a research hotspot. A typical TFT is composed of three electrodes (source, drain and gate) and a dielectric layer that is interfaced with a semiconductor material, and its performance depends strongly on the interfacial properties[156]. Flexible and stretchable TFTs have gained a great deal of interest in recent years for their wide applications in a variety of wearable electronics including displays, sensors, and communication. For example, Dai et al. proposed the high-performance transparent and flexible TFTs by using graphene nanosheets (GNSs)-amorphous indium-gallium-zinc-oxide (a-IGZO) composites synthesized by a solution process as the active channel on an ultrathin (100 μm) and flexible glass substrate (Fig. 17(a))[168], which exhibited a high electron mobility of 23.8 cm2/V S and excellent resistance to mechanical bending with the variation of 8% in mobility after 100 times of repeated cycling bending while the degradation of the pristine one was over 70%. To illustrate the application of flexible TFTs, Xu et al. developed a flexible active matrix OLED (AMOLED) display driven by oxide TFTs back panel on a flexible polyethylene naphthalate substr ate[169]. As shown in Fig. 17(b), anodic AlOx and a-IGZO were used as gate dielectric and active channel, respectively, for these bottom-gate-structure TFTs, and a 5-inch flexible AMOLED display demo with good image quality in both flat and bent states was then fabricated by incorporating bottom emission OLED pixels and thin film encapsulation on the flexible TFT back panel. Recently, Liang et al. reported a flexible and stretchable TFT entirely fabricated by solution-based techniques[11]. The stretchable TFT employed a Ag NWs-PU acrylate (PUA) composite as the stretchable transparent electrodes, single-wall CNT network channel, and a PU-co- polyethylene glycol (PU-co-PEG) elastomeric dielectric (Fig. 17(c)). The resulting devices showed a mobility of ~30 cm2/(V·s), an on/off ratio of 103–104, and relatively low operating voltage. More notably, the devices could be stretched by up to 50% strain and subjected to 500 cycles of repeated stretching to 20% strain without significant loss in electric property.

class="figure_img" id="Figure17"/>
Download
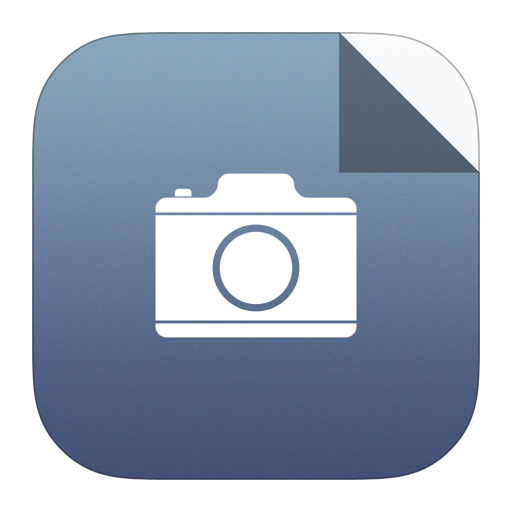
Larger image
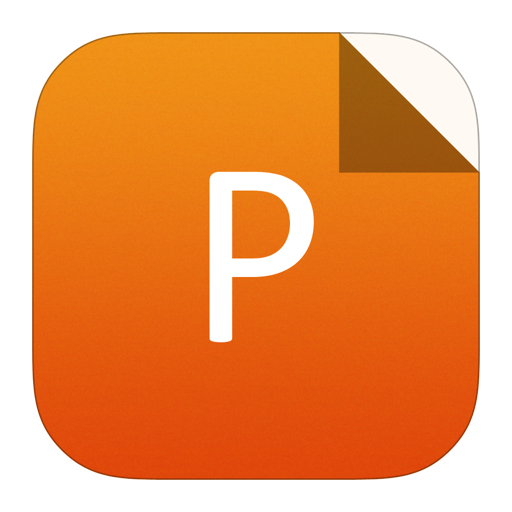
PowerPoint slide
Figure17.
(Color online) (a) Schematic of the transparent flexible TFT on an ultrathin glass substrate based on the solution-processed GNSs–a-IGZO channel. Reproduced with permission from Ref. [168]. Copyright 2013, Royal Society of Chemistry. (b) Cross-sectional view of the a-IGZO TFT on PEN substrate, and the photograph of flexible active-matrix OLED display driven by a-IGZO TFT arrays. Reproduced with permission from Ref. [169]. Copyright 2014, Royal Society of Chemistry. (c) Schematic of the flexible and stretchable TFT using printable Ag NWs, CNTs and an elastomeric dielectric, and optical image of a TFT array. Reproduced with permission from Ref. [11]. Copyright 2015, Macmillan Publishers Limited.
6.
Conclusions and perspectives
To date, flexible and wearable optoelectronic devices have been dramatically developed and have changed our life styles. Novel substrate materials, transparent conductive electrodes, light manipulations and device architectures all contribute to the innovations of high-performance flexible optoelectronic devices. Substrate materials are the supporting part and the transparent conductive electrodes with super flexibility or strechability are the foundation. Various TCEs made of graphene, metallic nanowires, and dielectric-metal hybrid structures are reviewed as above. Integrating the light extraction or trapping structures will be favorable for improving the device efficiency. With the increasing technology and market demands, a new era for flexible and wearable optoelectronic devices is coming. Electronic devices which can be rolled, bent, twisted, folded and even integrated to the human body will certainly be realised in the future.