1.
Introduction
Bioresorbable electronics or transient electronics[1] is constructed by bioresorbable materials that dissolve in biofluids and generate biological safe byproducts. These electronics devices maintain their functions in defined periods of time from seconds to months, and eventually disintegrate at molecular levels through hydrolysis and enzymatic degradation. Some pioneer works in developing bioresorbable electronics involve partially resorbable electronic devices[2, 3] and various types of bioresorbable materials that are suitable for constructing transient electronic devices[4–6], leading to fully bioresorbable devices that have been demonstrated as conceptual systems[1] as well as many basic electronic components[7–9].
Bioresorbable electronics can potentially lead to revolutionary changes in healthcare and consumer electronics. For example, implantable bioresorbable electronic systems can conduct internal post-surgical monitoring of organ, tissue, implant, and wound healing without the needs of surgery to remove them, leading to reduced risk of infection and complications caused by surgery. By replacing current electronic components within consumer electronics, the recycling processes of obsoleted devices can be much facilitated, resulting in reduced electronic waste and environmental pollution due to hazardous degradation byproducts[10–12]. As a relative new addition to modern electronic technology, bioresorbable electronics has gained significant progress in both fundamental mechanisms and applied techniques. It is considered to be a new type of flexible electronic technology, which can lead to innovative wearable systems that can disappear within a biological environment. This paper reviews materials, fabrication techniques, and applications of bioresorbable electronics and presents some existing challenges as well as potential solutions in the development of bioresorbable electronics. With more joint efforts, more revolutionary bioresorbable systems can be developed to generate broader social and economic impact that can benefit the wellness of both human beings and ecosystems.
2.
Materials used in bioresorbable electronics
Bioresorbable materials as well as their dissolution byproducts can be considered safe for the human body when used under the guidelines of body tolerance. They are used in formats of thin films and membranes to facilitate dissolution while offering flexibility to the entire devices. Thus, bioresorbable electronic devices are typically flexible, offering excellent compatibility to the mechanics of biological tissues. This section reviews the metals, polymers, inorganic compounds, semiconductors, and emerging carbon-based materials that have been used to construct bioresorbable electronics (Fig. 1).

class="figure_img" id="Figure1"/>
Download
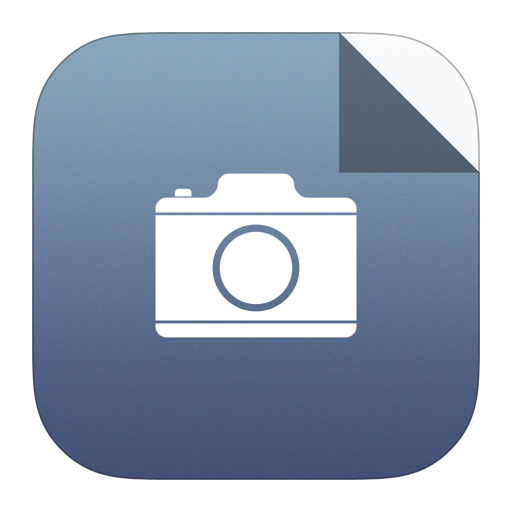
Larger image
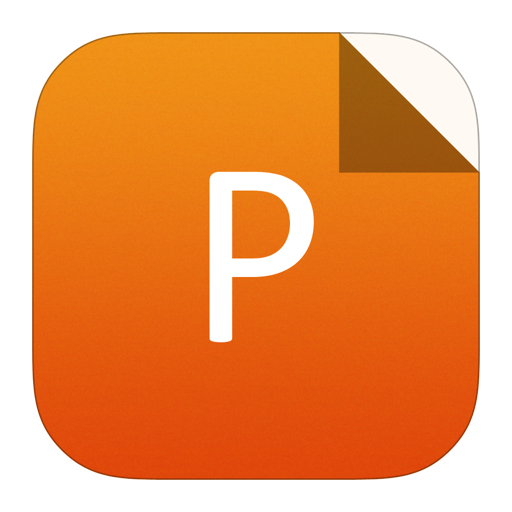
PowerPoint slide
Figure1.
(Color online) Materials and major electronic devices in bioresorbable electronics.
2.1
Metal
Bioresorbable metals typically include magnesium (Mg), zinc (Zn), and molybdenum (Mo) (Fig. 2), all of which can react with water molecules through hydrolysis and generate products such as Mg(OH)2, Zn(OH)2, and H2MoO4. Some of the dissolution products corrode slowly in aqueous conditions, but react with chloride ions in physiological conditions to produce metal chloride and hydrogen gas. The proper dose of bioresorbable metals is determined by their dissolution rates, body tolerance of hydrogen gas (<10μL(cm2·d)[13]), and their cytotoxicity.

class="figure_img" id="Figure2"/>
Download
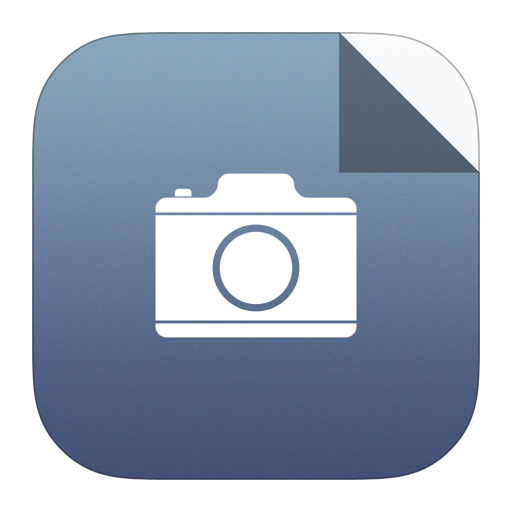
Larger image
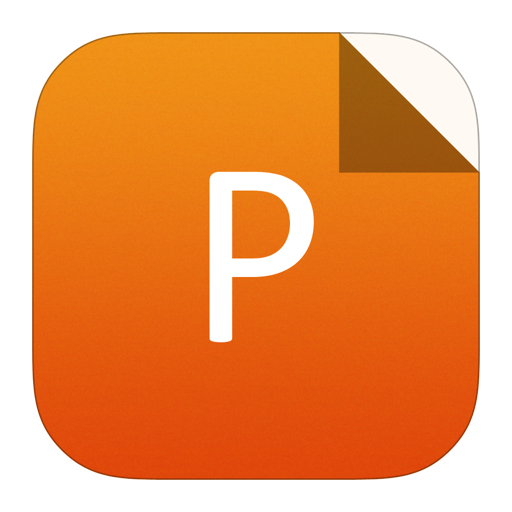
PowerPoint slide
Figure2.
(Color online) Typical bioresorbable metals used in bioresorbable electronics. Images used in the figures are reprinted with permission from Ref. [25] (Copyright 2008 Elsevier Inc.), Ref. [19] (Copyright 2013 John Wiley and Sons), Ref. [21] (Copyright 2016 Elsevier Inc.), and Ref. [23] (Copyright 2016 Nature Publication Group).
The most common transient metal used in bioresorbable electronics is Mg, which is wildly presented in cellular systems and is involved in all metabolic pathways. Mg has a rapid dissolution rate in the biological environment containing Cl? ions. Unprotected Mg first develops into slow dissolvable magnesium hydroxide (Mg(OH)2) in water, and then reacts with Cl? to form highly soluble Mg chloride (MgCl2) and hydrogen (H2) gas. The dissolution rate of Mg in stimulated body fluid is determined to be 19–44 mg/cm2/day[14], and is subjected to the influence of parameters, such as pH values, ion concentrations, and oxygen levels. In addition, immunological responses from the localized tissues due to direct and intimate contact[15] also affect Mg dissolution. Mg has been wildly used to construct conductive interconnects in bioresorbable circuits. However, the rapid corrosion rate of Mg in the electrolytic physiological environment limits its use in long-term implantable applications. Thus, a bioresorbable surface coating with a slow dissolution rate based on polycaprolactone[16], silicon carbide (SiC)[17], and silicon dioxide (SiO2)[18] can be introduced to protect Mg patterns from direct exposure to surrounding environments.
An alternative bioresorbable metal with slower corrosion rate is Zn. In-vivo experiments by implanting Zn wires in the abdominal aorta of rats show uniform corrosion behaviors for the first 3 months, and severe, localized corrosion after 4.5 months, leading to increased dissolution rates from ~12 μm/y in the first month to ~50 μm/y after implantation for six months[19] . Zn has been used as a single construction material for stents[20] and scaffolds[21], or used as an alloying material by combining with Mg or Ca. As Zn is more stable in organic solvents than Mg, thus Zn nanoparticles can be used as fillers[22] in conductive inks and pastes to construct circuit patterns and sensors. In addition, Zn foils can also be used as electrodes[4] for bioresorbable batteries.
Another promising bioresorbable metal is Mo, which is an essential trace element that is needed for serval oxidase enzymes. Mo compounds have low solubility in pure water, and possess increased solubility in oxygen-rich water. The dissolution rate of Mo in buffer (pH = 7) and NaCl solutions is between 10?4 and 10?3 μm/h at room temperature[4]. Such a slow dissolution rate is ensential for constructing bioresorbable devices that are required to work sustainedly in an aqueous enviroment. Mo has been used as an electrical interconnect between sensors and external readout[23], and has been used to make electronic components such as electromagnetic coils and resistive heaters for thermally actuated drug delivery[24].
2.2
Polymer
Bioresorbable polymers can be degraded either through hydrolysis of carbonyl functional groups (i.e. esters, amides, and carbonates) or enzymic secreting in biological environments, resulting in dramatic changes in their chemical structures. In addition, effectiveness of water uptake in hydrophilic polymers leads to more direct interaction between water molecules and physical structures as well as chemical bonds within the polymers. Thus, hydrophilic polymers dissolve faster as compared with hydrophobic polymers.
A major type of bioresorbable polymer is aliphatic polyester, which is available in formats such as polylactic acid (PLA)[26–28], polyglycolic acid (PGA)[29], polylactic-co-glycolic acid (PLGA)[30, 31](Fig. 3(a)), and poly-ε-caprolactone (PCL)[32, 33]. These polymers have been approved by the US Food and Drug Administration (FDA) for clinical use[34, 35] in tissue engineering and bone repair implants.
Among the aliphatic polyesters, PLA is one of the most widely used biodegradable polymers in biomedical applications, and is classified into crystalline poly-l-lactic acid (L-PLA or PLLA) that is inert to hydrolysis and amorphous poly-dl-lactic acid (DL-PLA or PDLA), which is sensitive to hydrolysis. Another common aliphatic polyester is PGA, which has a high degradation rate as compared with PLA. PLA can be copolymerized with PGA to yield PLGA, whose dissolution rate can be tuned by crystallinity and the ratio of PLA and PGA in the copolymers. A more biologically stable polymer is PCL, which has high crystallinity (>45%)[36] and hydrophobic behaviors, offering a slower dissolution rate in a biological environment as compared with PGA and PLGA. Due to its good flexibility and machinability, PCL can also be copolymerized with other polymer biomaterials including PLA and PLGA to yield unique properties such as shape memory and elasticity. Aliphatic polyester has been used as substrates for organic thin film transistors[37], sensors[9, 23], and functional circuits[24], or worked as a surface coating to extend the implantation period of bioresorbable electro nics[38].
Different from aliphatic polyesters, which exhibit bulk erosion behavior, surface erosion polymers that dissolve layer by layer offer much better encapsulation performance. One notable example is polyanhydride, which allows sustained protection of bioresorbable electronic devices from the external environment. Biocompatibility studies of polyanhydride conducted both in vitro and in vivo indicate that its degradation products are non-mutagenic and non-cytotoxic. Polyanhydride has been used as the packaging materials of a complete bioresorbable primary battery[39], which can last 24 hours in liquid electrolyte. In addition, bioresorbable implantable devices showed a longer working period when packaged with polyanhydrides[40].
In addition to the synthesized polymers, natural polymers based on naturally existing proteins are also important bioresorbable materials. These polymers can be derived from proteins such as silk fibroin, collagen, gelatin, elastin, and albumin, and can be used as single constitution components or combined with other bioresorbable polymers such as PLA[41], PLGA[42], and PCL[43] to tune their mechanical and degradation properties. Some of these polymers such as silk and gelatin have been used as substrates for bioresorbable electronics (Fig. 3(b)), and some (e.g. chicken albumen[44], silk[45], gelatin[46]) can be used as the dielectric for transistors. In addition, natural proteins may be used to construct dielectric-modulated transistors for sensing specific biomolecules using receptor/ligand systems such as biotin/streptavidin[47], immunoglobulin E (IgE)/anti-IgE[48], and albumin/anti-albumin[49].
Stretchability of the bioresorbable electronic devices, which couple with curvilinear and stretchable biological tissues can be achieved using bioresorbable elastomers as substrates. One of the common elastomeric polymers is poly(glycerol sebacate) (PGS), which can be obtained through covalently crosslinked random coils with hydroxyl groups attached to its backbone. PGS materials offer 267 ± 59.4% stretchability with average Young’s modulus in the range of 0.282 ± 0.0250 MPa and ultimate tensile strength larger than 0.5 MPa[50]. PGS has been used as the dielectric for fully resorbable pressure sensors based on parallel plate capacitors, and has shown more than 7-week stable performance in a PBS buffer solution[51]. Another typical elastomeric polymer poly(1, 8 octanediol citrate) (POC) can be synthesized through the polycondensation reaction between citric acid and linear aliphatic diols (1, 8-octanediol). The mechanical properties of POC can be tuned by types of diol monomers and processing parameters such as post-polymerization time[52] and temperature, allowing tunable Young’s modulus from 2.84 to 6.44 MPa and stretchability from 253% to 375%[52]. POC has been used to construct epidermal sensors together with Mg electrodes, SiO2 surface coating and silicon nanomembranes (Si NMs). The resulting devices demonstrate more than 30% stretchability with capability to conduct biopotential and pH sensing on skin[53] (Fig. 3(c)).

class="figure_img" id="Figure3"/>
Download
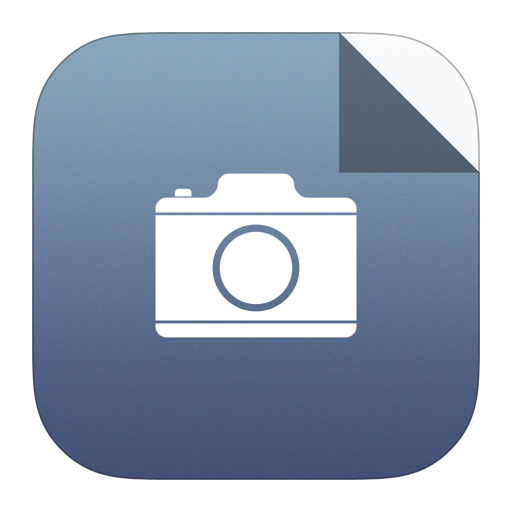
Larger image
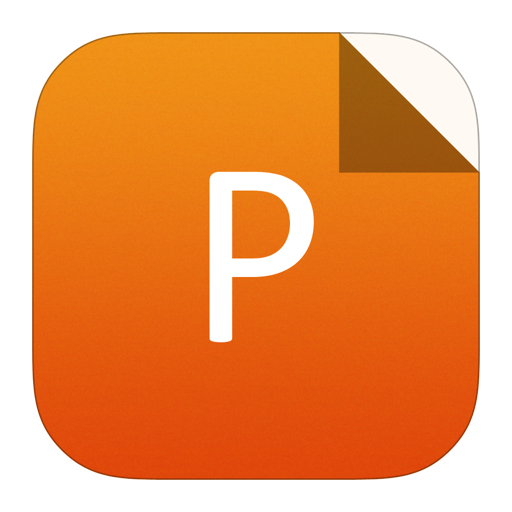
PowerPoint slide
Figure3.
(Color online) Typical bioresorbable polymers used in bioresorbable electronics. (a) An implantable pressure sensor based on a PLGA substrate (reprinted with permission from Ref. [40]. Copyright 2016 Nature Publication Group). (b) An in-vivo infection abatement device based on a silk substrate (reprinted with permission from Ref. [54]. Copyright 2014 National Academy of Sciences). (c) A stretchable bioresorbable device based on POC (Reprinted with permission from Ref. [53] Copyright 2015 American Chemical Society.)
2.3
Inorganic compounds
Inorganic compounds possess small dissolution rates and high chemical stability, and are typically used as surface coating layers to protect bioresorbable implants or as constituent materials for ceramics and composites. The electronic use of inorganic compounds includes gate dielectric for transistors, spacer layers for capacitors, and insulation layers.
In bioresorbable electronics, silicon dioxide (SiO2) is used as dielectric (dielectric constant = 3.9) or insulation layers (resistivity = 1014–1016 Ω·cm), and used as a passivation material to protect the bioresorbable electronic devices from a surrounding aqueous environment. The reaction product of SiO2 hydrolysis is silicic acid Si(OH)4, which is a naturally occurring component in biofluids with a typical concentration in serum ranging from 14×10?6 to 39 × 10?6 M[55]. The reaction to form silicic acid is catalyzed by OH?, hence the hydrolysis rate of SiO2 increases with increasing pH values[56]. In addition, the dissolution kinetics of SiO2 is also influenced by the concentration of other ions such as K+, Na+, Ca2+, and Mg2+[57]. Furthermore, SiO2 obtained through different processes also exhibits varied dissolution rates[57].
Another inorganic compound used in bioresorbable electronics is silicon nitride (Si3N4), which is a preferred ceramic material known for its chemical stability, high wear resistance, and low friction coefficient. The electronic properties of Si3N4 include a high dielectric constant (dielectric constant = 7), large electronic gap, and high resistance against radiation, leading to the extensive use of Si3N4 in microelectronic devices as a gate dielectric in thin-film transistors[58] and as a charge storage medium in nonvolatile memories[59]. Dissolution of Si3N4 in the physiological environments contains two-step reactions that can eventually turn Si3N4 into silicic acid (Si3N4 + 12H2O → 3Si(OH)4 + 4NH3). Due to the generation of SiO2 in the reaction, the dependence of the hydrolysis rate on pH and ion concentrations is expected to be similar to that observed in SiO2[57].
In addition to the inorganic compounds mentioned above, magnesium oxide (MgO), which is the eighth most abundant element in the earth's curst, is also wildly available as dielectrics and packaging materials in bioresorbable electronics. The dissolution of MgO is associated with the formation of a hydrated intermediate Mg(OH)2 in neutral and acidic solutions[60], and is strongly influenced by the presence of H+ ions[61]. MgO thin films have attracted significant attention due to their properties such as high electrical resistivity, high optical transparency, good chemical resistance, excellent thermal stability, and low refractive index. MgO is an ideal dielectric material for electronics due to its relatively higher permittivity (8–10)[62], and has been used as a gate dielectric in making transistors[63] and spacer layers of capacit ors[64].
2.4
Semiconductor
The semiconductor materials are significant in achieving active components in bioresorbable electronics. The most frequently used semiconductor material is silicon (Si), which has been proved to be biologically safe. Another semiconductor material that hydrolyzes in water is germanium (Ge), whose biological effect has not yet been fully studied. In addition, bioresorbable organic semiconductors such as β-carotene, indigo, perylenediimide, and indanthrene yellow G may also be used to construct active components in bioresorbable electronics.
Si is a trace element in the human body, and widely available in food and drinking water. Si is generally considered to be a chemically stable material due partly to the formation of native oxide on its surface. However, at nanoscale thickness (i.e., in the form of nanomembranes), even slow reaction and degradation rates can significantly alter the morphology and properties of Si, leading to complete hydrolysis of Si nanomembranes (Si NMs) in a scale of a few days or weeks. Recent systematic studies of the hydrolysis of mono-Si(100) in solutions with different pH values (between 6 and 10), ion concentrations, and temperature indicate that the dissolution rates of Si with low or modest doping levels are in the range of 0.5?624 nm/d. These values are well within a range that leads to complete disappearance of Si NMs (~300 nm or less) on time scales that are relevant for many potential implantable applications[65]. The toxicity studies of Si NMs have been conducted in live rats, suggesting excellent biocompatibility of Si NMs at device levels[66]. No dissolution residue has been observed by both the naked eye and stereomicroscopic analysis after a 5-week post implantation of Si NMs.
Bioresorbable organic semiconductors can be derived from natural pigments such as β-carotene[67] and chlorophyll[68] or from dyes such as indanthrene yellow G[69], brilliant orange RF[69], perylenediimide[70], and indigo[71], which are typically used as food, textile and cosmetic colorants[72]. These organic semiconductors contain π-conjugated carbon backbones that allow delocalization of electronics. Some organic materials such as β-carotene, chlorophyll, and indigo typically have low water solubility[73], and can be degraded within the body to yield dissolvable products[74]. The utilization of these semiconductors in making organic thin film transistors has been explored by many researchers[75], however these natural organic semiconductors show intrinsic low mobility (~4 × 10?4 cm2V?1s?1 β-carotene and ~2 × 10?4 cm2V?1s?1 for indigo)[69] that is compared much less than many semiconductor materials such as silicon, carbon nanotube, and pentacene. Thus significant efforts are still demanded to improve the electrical properties of bioresorbable organic semiconductors to yield high performance electronics for realistic applications.

class="figure_img" id="Figure4"/>
Download
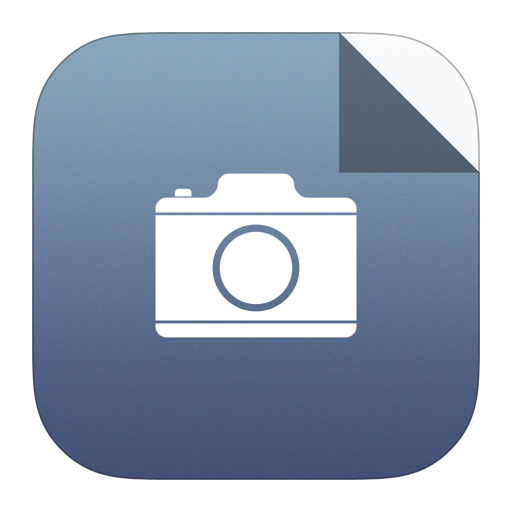
Larger image
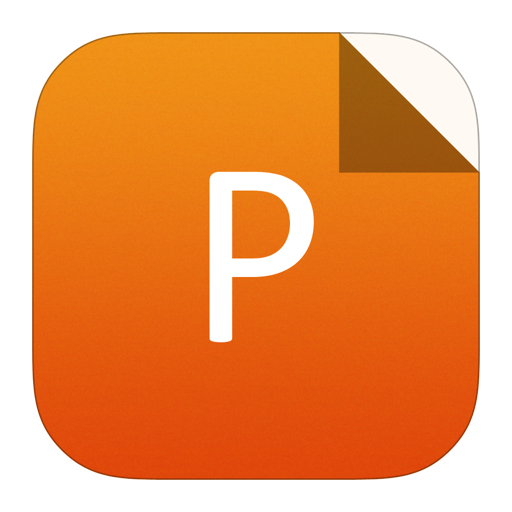
PowerPoint slide
Figure4.
(Color online) Bioresorbable semiconductor materials. (a) Silicon nanomembranes (reprinted with permission from Ref. [76] Copyright 2013 John Wiley and Sons). (b) Bioresorbable organic semiconductor materials (reprinted with permission from Ref. [69] Copyright 2010 John Wiley and Sons). (c) Composites based on carbon-based materials and silk (reprinted with permission from Ref. [77] Copyright 2016 American Chemical Society).
2.5
Carbon-based materials
Carbon-based materials such as carbon nanotubes (CNTs) and graphene can be potentially used to construct bioresorbable electronic devices. The biocompatability and dissolution of such materials have not yet been systematically studied. However, resent studies have indicated that surface functionlized single wall carbon nanotubes (SWCNTs) and multi-wall carbon nanotubes (MWCNTs) using carboxylate can be degraded by oxidative enzymes, while pristine SWCNTs and MWCNTs show strong resistance to the same enzymes[78]. Applications of carbon-based materials in bioresorbable electronics involve using graphene printed on a silk membrane to selectively detect bacteria on tooth enamel through self-assembly of antimicrobial peptides on graphene[3]. In addition, SWCNTs and graphene have been fed to silkworms[77] to yeild electrically conductive silk with highly developed graphitic structures, paving a new way to produce strengthened silk fibers in bioresorbable electronics.
3.
Fabrication of bioresorbable electronics
Bioresorbable electronic devices are predominately achieved by complex, time-consuming processes based on CMOS technology. As bioresorbable materials are subject to the influence of hydroxyl, oxygen, and heat, which can trigger material degradation and surface oxidation. Thus, the fabrications processes have to be carefully selected to avoid the exposure of the materials to excessive water, oxygen, and heat. A typical fabrication process of bioresorbable electronics has been shown in Fig. 5(a). It starts with spin-coating a sacrificial layer (Poly(methyl methacrylate) (PMMA) and a diluted polyimide (Di-PI) layer on a silicon substrate. Si NMs that have been doped on selective regions can then be transfer printed from a silicon-on-insulator (SOI) wafer to the silicon substrate, followed by a dry etching process to yield active components. Transient metal layers and dielectric layers can be deposited and patterned to form interconnects, electrodes, and contact pads. All photoresists as well as PMMA used in the process can be lifted off in acetone, avoiding the introduction of any water-based solvent. Eventually, the bioresorbable devices released in acetone by removal of PMMA can be picked up by PDMS stamps, followed by removal of the Di-PI layer through plasma etching. Transfer printing of the resulting devices to moisturized bioresorbable polymer substrates completes the fabrication process. Instead of using the photoresist lifting-off process to define transient metal patterns, stencil shadow masks can also be introduced as a more direct approach to define the metal patterns during deposition. Due to the use of special chemicals and processes for minimizing the use of excessive water and heat, the additional cost and time consumed during the fabrication of bioresorbable electronics lead to challenges in mass fabricating bioresorbable electronics, urging the need to develop new fabrication approaches with lower cost and shorter time.
The recent effort in developing new fabrication techniques of bioresorbable electronics has led to devices based on direct laser cutting[24], aerosol or inkjet printing[79], screen printing[22] (Fig. 5(b)), evaporation vapor condensation[80] (Fig. 5(c)), and photonic sintering[79], demonstrating cost-effective and rapid-prototyping approaches as compared with the traditional CMOS fabrication process. The majority of these approaches used bioresorbable metals micro/nanoparticles to prepare inks or pastes, which can be printed to form conductive patterns on bioresorbable polymer substrates, featuring completely anhydrous fabrication processes that are suitable for bioresorbable materials. It is expected that with the addition of carbon-based materials that can be used to make either conductive or semiconductive elements, more functional bioresorbable inks and pastes can be developed, leading to fully bioresorbable electronic systems by printing approaches.

class="figure_img" id="Figure5"/>
Download
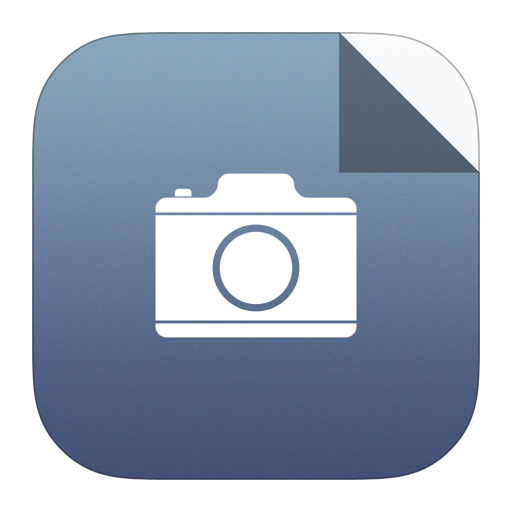
Larger image
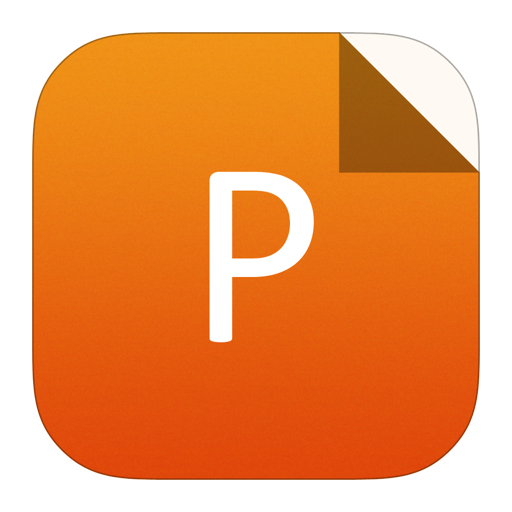
PowerPoint slide
Figure5.
(Color online) Fabrication techniques in bioresorbable electronics include (a) CMOS fabrication, (b) printing technology and photonic sintering (reprinted with permission from Ref. [79]. Copyright 2017 John Wiley and Sons), and (c) evaporation vapor condensation (reprinted with permission from Ref. [80]. Copyright 2017 John Wiley and Sons).
4.
Applications of bioresorbable materials in electronics
Bioresorbable materials have been used to make electronic devices that work either as single components or simple systems. Existing bioresorbable electronic devices include batteries, sensors, passive components (e.g. resistors, capacitors, inductors), and active components (e.g. diodes and transistors). These devices can be combined to yield functional circuits for power harvesting, signal generation, and amplification or to construct simple systems to realize drug delivery, therapy, and implantable monitoring. Some selective devices and systems are introduced below.
Bioresorbable batteries[39, 81–83] are the basic components in bioresorbable systems. Current formats of bioresorbable batteries mainly involve primary batteries. Lan et al. presented a bioresorbable primary battery based on stacked Mg–Mo cells filled with phosphate buffered saline as an electrolyte in a polyanhydride package. Four such cells with an overall dimension of 3 × 1.3 × 1.6 cm3 are combined to yield a stable voltage output at 1.5–1.6 V for up to 6 hours[39]. An alternative gel-like electrolyte, which is made of choline nitrate is used by Jia et. al. in an ultra-compacted magnesium-air battery[82]. The electrolyte is embedded in a chitosan package sandwiched by a polypyrrole–para(toluene sulfonic acid) cathode and a Mg alloy anode, resulting in a battery with an overall thickness of only 300 μm and a maximum volumetric power density of 3.9 W/L. A rechargeable battery that is dissolvable has been demonstrated by Douglas et al.[83] (Fig. 6(a)). This battery contains a gel-like mixture of PVA/LiClO4 as electrolytes in a PEO chamber sandwiched by porous Si electrodes coated with vanadium oxide (VOx). Although this battery has not been proved to be biologically safe, it represents an important concept of triggered dissolution in which externally applied alkaline solutions can trigger the dissolution of VOx in the battery, resulting in rapid disintegration of VOx and deactivation of the energy storage function of the battery.

class="figure_img" id="Figure6"/>
Download
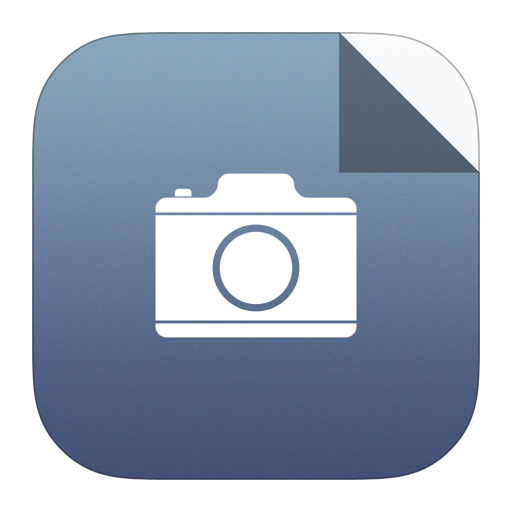
Larger image
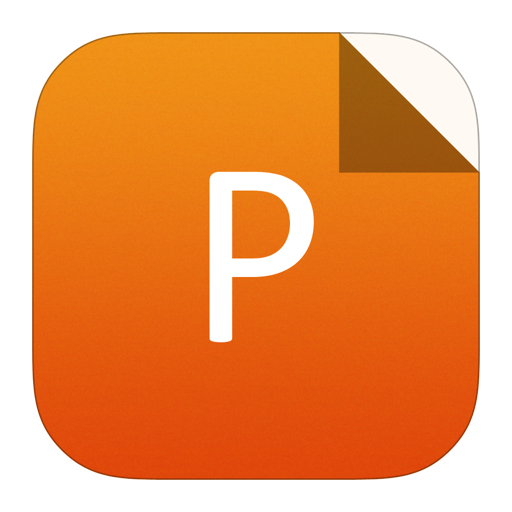
PowerPoint slide
Figure6.
(Color online) Examples of several bioresorbable electronics devices, including (a) batteries (reprinted with permission from Ref. [83]. Copyright 2016 Royal Society of Chemistry), (b) implantable sensors (reprinted with permission from Ref. [23]. Copyright 2016 Nature Publication Group), (c) transistor arrays (reprinted with permission from Ref. [76]. Copyright 2013 John Wiley and Sons), and (d) multifunctional systems (reprinted with permission from Ref. [38]. Copyright 2015 American Chemical Society).
Another important component in bioresorbable electronics are sensors, which have been realized in the formats of voltage sensing electrodes[23, 40], pressure sensors[51, 84, 85], motion sensors[40], and temperature sensors[40]. Yu et al. developed neural interfaces using Si as electrodes to allow biopotential sensing on the cerebral cortex of mice in a working period from hours to months[23] (Fig. 6(b)). When combined with arrays of MOSFETs realized by Si NMs, the interface can conduct multiplexing sensing with 64 electrodes to map electrocorticography on subgaleal space. Kang et al. also developed multifunctional Si sensors for monitoring temperature, acceleration, pressure, flow rate and pH values within the brain[40]. The sensors contain Si NMs as sensing elements, which are sealed within SiO2 on PLGA substrates. The above-mentioned sensors are both connected with external measurement circuits and power supplies. Improved approaches developed by Luo et al. use an electromagnetic field to drive pressure sensors[85]. The sensor contains two Zn/Fe bilayers sandwiching an air gap formed within a PCL spacer. The Zn/Fe bilayers contain an inductive coil and parallel capacitive plates, forming a LC tank circuit that allows sensing the deflection of the parallel plates through changes in the resonance frequency of the circuit. The fabricated sensor has been used to measure external pressure with a sensitivity of 39 kHz/kPa for up to 20 kPa.
Active components such as diodes and transistors have been made by inorganic and organic semiconductor materials, leading to the appearance of bioresorbable circuits with functions such as amplification, multiplexing, radio frequency generation, and power harvesting. Most inorganic active components are made of Si NMs, which are processed following the procedures as mentioned in the fabrication section, allowing the achievement of either discrete components or high-density arrays on bioresorbable substrates (Fig. 6(c)). Besides using inorganic semiconductor material, organic materials have demonstrated their potential as dielectric and semiconductor materials. For example, Capelli et al. demonstrated OFETs and organic light emitting transistors (OLETs) using both n-type perylene and p-type thiophene with silk fibroin as a dielectric. The charge mobility and on/off ratio of the bioresorbable OFETs are measured to be on the order 10?2 cm2/(V·s) and 104, respectively, while the n-type OLETs offer light emission of ~100 nW[86]. Other natural organic semiconductor materials such as vat yellow 1, vat orange 3, β-carotene, and indigo have been used to make inverters[71], ring oscillators and memory elements[87]. However, the relative large gate voltage from 4.5 to 7 V and small electron mobilities (~1 × 10?2 cm2V?1s?1) are still the major obstacle to achieving high performance organic bioresorbable electronics.
Simple systems have also been demonstrated by combining the above mentioned components. A radio-frequency power harvesting system has been developed to convert environmental electromagnetic fields into DC power. This system contains both passive and active components, including inductors, capacitors, resistors, diodes, transistors, conductive interconnects and dielectrics on silk substrates[1]. This conceptual system has inspired many succeeding systems with realistic biomedical applications. For example, Lee et al. reported a drug delivery device that uses four conductive coils with different resonance frequencies to selectively generate heat within four resistors[24]. The heat can be used to drive corresponding temperature sensitive lipid films to release drug molecules. In addition, Song et al. developed a multifunctional bioresorbable electronic stent capable of conducting flow sensing, temperature monitoring, data storage, inflammation suppression, localized drug delivery, and hyperthermia therapy[38] (Fig. 6(d)).
5.
Conclusions
This paper has presented the state-of-the-art devices and their construction materials which offer various options to construct fully bioresorbable electronic systems. Despite the great achievement of bioresorbable electronics, some critical challenges in fabrication, packaging, and the dissolution mechanism of bioresorbable electronics still exist and require further development.
Firstly, the complex and time-consuming fabrication approaches based on CMOS technology lead to low yield and high cost when fabricating complex bioresorbable electronic systems. Investigations of new fabrication methods involve a series of printing and sintering approaches such as screen printing, inkjet printing, condensed laser evaporation, and photonic sintering that can be conducted in atmosphere conditions and at room temperature. These techniques may combine with CMOS technology and materials based on nanotube, nanowires, nanoribbon and nanoparticles to yield revolutionary fabrication technology that is suitable for mass production of bioresorbable electronics systems.
In addition, high quality encapsulation materials and surface packaging that isolate bioresorbable electronics from the external environment have not yet been achieved. One solution may be to use a surface erosion polymer such as polyanhydrides with tunable crystallinity to yield controllable encapsulation performance. Another approach may use stacked inorganic compounds such as SiO2, Si3N4, and MgO deposited through plasma enhanced chemical vapor deposition or atomic layer deposition. Moreover, the electronic devices can also be sealed inside transient metal films/foils that can significantly slow down the dissolution processes.
Furthermore, current spontaneous dissolution mechanisms of surface coating and composition materials may be replaced by more controllable triggered dissolution approaches, which deactivate the device function through stimulation in the formats of heat, light, force, moisture, or electrical signals. It is also noticeable that many bioresorbable materials such as alloys, composites, and ceramics that have demonstrated their use in biomedical implants and their capabilities in improving mechanical and chemical properties of the implants are largely unexplored in bioresorbable electronics. Utilization of these materials in bioresorbable electronics may lead to devices with unique function and improved performance.
Bioresorbable electronic technology offers innovative solutions to eliminate the need for secondary surgeries and minimizes the generation of electronic waste. With more efforts to advance bioresorbable materials and fabrication approaches as well as other relevant techniques, more complex, fully implantable, and self-supported bioresorbable electronic systems may eventually appear and become wildly available, generating more impacts to the wellness of human beings and the environment.