1.
Introduction
In the last few years, there has been an explosion in the interest of devices suitable for ultra-high frequency applications such as in the Tera-Hz regime; this interest is witnessed in the benchmarks which have been made mainly in signal amplification where it reaches its peak value about 1 THz using both InP HEMT and InP HBT technologies[1–7]. The high-frequency characteristics and low noise performances of InP-based high electron mobility transistors (HEMTs) have made this technology the most important in the domain of millimeter or sub-millimeter wave applications[8]; the outstanding performances that have been obtained are the result of the combination of reducing the parasitic phenomena, using a high electron mobility channel material, the higher sheet charge densities and through the downscaling of devices to their minimum feature size[9]. The InP-based HEMTs have taken several studies in order to improve their performances, mainly for the frequency such as[10] whose authors have used an asymmetrically recessed gate, which has made an important minimization in the parasitic resistances consequently to the reported decreases of contact resistance. In Ref. [11] the authors have reported on the influence of barrier thickness and gate length on the various device parameters. In Ref. [12] the possibility of operating a pseudomorphic Indium Phosphide HEMT in the terahertz region has been studied by numerical simulation based on the Chalmers HEMT design by the author. In Ref. [13] a complex study of the influence of nanoscale InAs inserts into the channel material on the structural and electrical properties of the device has been performed. Many fields need the added performances in output characteristics of the device such as in bioengineering whose low value of output current will be adding to the highest frequency that characterized them. The electrical signal (such as the current) in the field of biomedical engineering is characterized by its low value and the noise to which it will be exposed, such as a signal that is characterized by a high frequency. Otherwise, in more professional terminology the relevant information reported in the biomedical signal is not visible from the use of an electric device with low power and which is sensitive to the low current value required. In this paper we perform the drain current modulation depended as a function of substrate structural energies in order to present the characteristic description of the substrate, which can produce a device likely to be functional with low current values. This purpose must take into account the operation of a device at high frequencies.
2.
Device structure
In this work we present the performance of 20 nm gate length for an HEMT based on InAsxP1–x in their substrate in order to study the drain current modulation as a function of structural substrate energies, this is important to present the effect of reducing the substrate energy and their effect on the drain current characteristic and all other characteristics. The cross-sectional view of the simulated structure is shown in Fig. 1, which is used in Ref. [14], except our structure differs in the substrate material that we use InAsxP1–x to reach our objective and to present the effect of reducing the structural energy of the substrate. Note that the introduction of Arsenic in the chemical composition of InP makes the reduction in the structural energy possible.

class="figure_img" id="Figure1"/>
Download
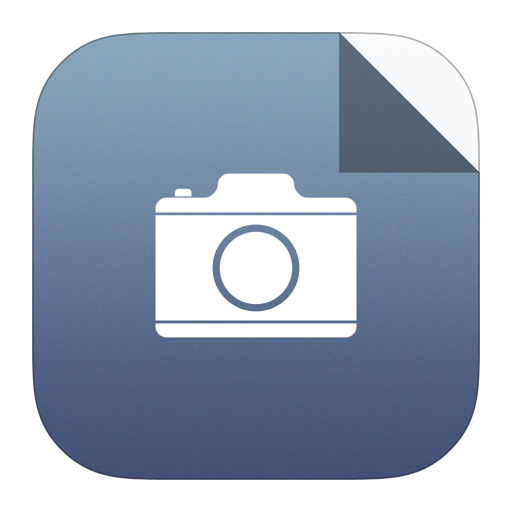
Larger image
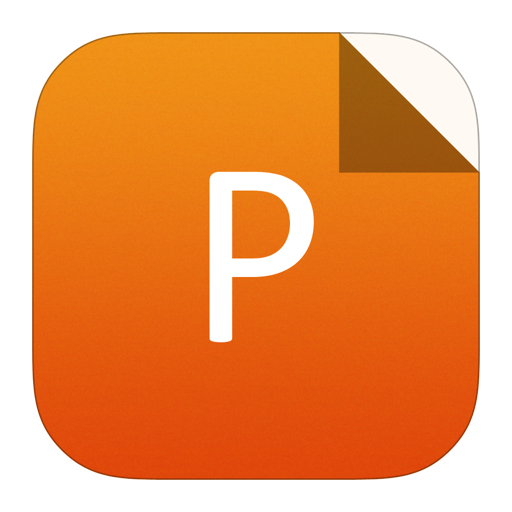
PowerPoint slide
Figure1.
(Color?online) Structure of simulated InAsP-Based HEMT.
The structure consists of 5 μm of a semi-insulating InAsxP1–x substrate, and 300 nm of intrinsic In0.52Al0.48As buffer layer, which is necessary to isolate the composite channel from the substrate defects. The structure is formed by a double Si-delta-doping level with 1 nm in thickness (5 × 1012 cm?3 for the upper and 2 × 1012 cm?3 for the lower), it is necessarily used to increase the sheet charge density in the channel that in turn causes an increase in the transconductance gm and drain current Ids[14]. The simulated device has 2 nm in both spacers (lower and upper) formed by In0.52Al0.48As, a composite channel formed by 5 nm of intrinsic InAs for the core channel, 3 and 2 nm of intrinsic In0.7Ga0.3As lower sub channel and upper sub channel respectively. The Schottky barrier layer is about 2 nm formed by the intrinsic In0.52Al0.48As and under the gate region, which was buried by 1 nm of a thin platinum metal layer. A 6 nm thick InP etch stop layer is crossed to minimize the access resistance for the both regions of source and drain electrodes. In our structure, we use a heavily doped multilayer cap formed with three levels. The first is the upper with 5 nm of thick based on the In0.7Ga0.3As layer (5 × 1019 cm?3), the second is 10 nm thick based on the In0.53Ga0.47As layer (5 × 1019 cm?3), and the third is with 5 nm of intrinsic In0.52Al0.48As layer. The source and drain ohmic contacts were formed using a Ti/Pt/Au (25 nm/25 nm/200 nm) metal stack and the T-gate is formed by a Pt/Ti/Pt/Au (7 nm/50 nm/50 nm/150 nm) metal stack. Finally, a 60 nm thick Si3N4 layer was used for device passivation. The gate length it chosen to be about 20 nm, 500 nm for the source to drain separation was taken, and 45 nm in the side recess spacing (LSIDE).
3.
Model and method
The evolution of the improvement in the devices performances is limited with their reduction to the low scale (nano order) also the limits related to the manufacturing process, hence new concepts needed inventing in order to ensure the desired continuity of improving the device performances. Among these, we found the concept related to substrate proprieties, on which we based our study.
All research into device physics has resulted in a mathematical model that operates on any semiconductor device and which consists of a set of fundamental equations[15] for this model which link together the electrostatic potential and the carrier densities, within some simulation domain. These equations, which are solved inside any general purpose device simulator such as TCAD-SILVACO, have been derived from Maxwell's laws and consist of Poisson's Equation, the continuity equations, and the transport equations. Poisson's Equation relates variations in electrostatic potential to local charge densities. The continuity and the transport equations describe the way that the electron and hole densities evolve as a result of transport processes, generation processes, and recombination processes.
Poisson's Equation relates the electrostatic potential to the space charge density and is given by the following relation:
$${ m {div}}(epsilon nabla psi ) = - ho ,$$ ![]() | (1) |
where ε is the electrostatic potential,

The local space charge density is the sum of contributions from all mobile and fixed charges, including electrons, holes, and ionized impurities. The electric field is obtained from the gradient of the potential, see the following relation:
$$E = - nabla psi.$$ ![]() | (2) |
The carrier continuity equations for electrons and holes are defined by equations:
$$frac{{partial n}}{{partial t}} = frac{1}{q}{ m {div}}{J_{ m{n}}} + {G_{ m{n}}} - {R_{ m{n}}},$$ ![]() | (3) |
$$frac{{partial p}}{{partial t}} = - frac{1}{q}{ m {div}}{J_{ m{p}}} + {G_{ m{p}}} - {R_{ m{p}}},$$ ![]() | (4) |
where n and p are the electron and hole concentration,
m{n}}} $

m{p}}} $

The current density equations, or charge transport models, are usually obtained by applying approximations and simplifications to the Boltzmann Transport Equation and which lead to a result in a number of different transport models such as the drift-diffusion model, the Energy Balance Transport Model, or the hydrodynamic model.
The simplest model of charge transport that is useful is the drift-diffusion model, which is the model that will be used in our study. This model has the attractive feature that it does not introduce any independent variables in addition to Ψ, n, and p. Until recently, the drift-diffusion model was adequate for nearly all devices that were technologically feasible. The more conventional formulation of the drift-diffusion model is given by the following relations for electrons and holes:
$${J_{ m{n}}} = qn{mu _{ m{n}}}{E_{ m{n}}} + qfrac{{k{T_{ m{L}}}}}{q}{mu _{ m{n}}}{nabla _{ m{n}}},$$ ![]() | (5) |
$${J_{ m{p}}} = - qp{mu _{ m{p}}}{E_{ m{p}}} - qfrac{{k{T_{ m{L}}}}}{q}{mu _{ m{p}}}{nabla _{ m{p}}},$$ ![]() | (6) |
where μn and μp are the electron and hole mobilities, TL is the lattice temperature, and k is Boltzmann's constant.
4.
Results and discussion
In Figs. 2 and 3 we perform the electron concentration in the substrate region as a function of substrate composition under the effect of Vgs and Vds respectively. The increases of the arsenic molar fraction in InP induce the decreasing of substrate structural energies. Consequently, it leads to an increase in electrons injected towards the substrate region shown in Figs. 2 and 3. The electrons concentration under x = 0.1 and Vgs = 0.6 V are 53.9 × 1019, 51.5 × 1019, and 49.2 × 1019 cm?3 under Vds = 0.4 V, Vds = 0.6 V, and Vds = 0.8 V respectively; the increase of molar fraction to 0.5 induces increases of 10.20 %, 10.87 %, and 11.78 % in the injected electron concentration where they become 59.4 × 1019, 57.1 × 1019, and 55 × 1019 cm?3 respectively in the same condition of Vds and Vgs. Similarly for x = 0.9 where the electron concentrations are 67.1 × 1019, 65.2 × 1019, and 63.1 × 1019 cm?3 respectively. The increases of injected electron concentration with increasing Vgs is due to increasing the gate bias effect shown in Fig. 2, which will be added to the reported decrease of structural energies in the substrate material, in contrast to the case of increasing the drain bias when the injected electrons concentration decreases due to decreasing the gate bias effect with increasing the drain bias shown in Fig. 3. The outstanding values of the obtained results related to electron concentration in the substrate level can be explained by the increases of the field effect that results by the gate bias and which it is added to the reported decrease of structural energies in the substrate. Consequently, this makes an important increase in the accumulated electron concentration in the substrate.

class="figure_img" id="Figure3"/>
Download
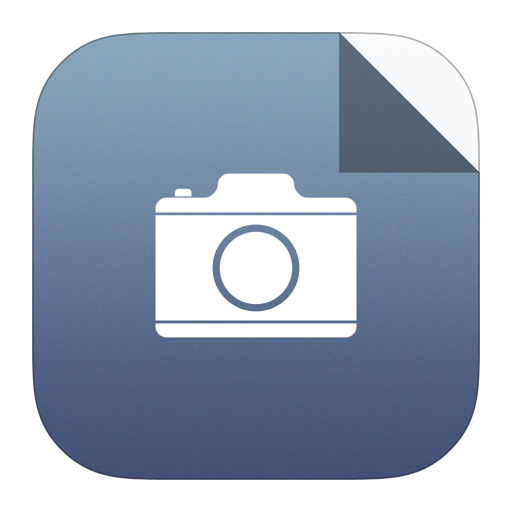
Larger image
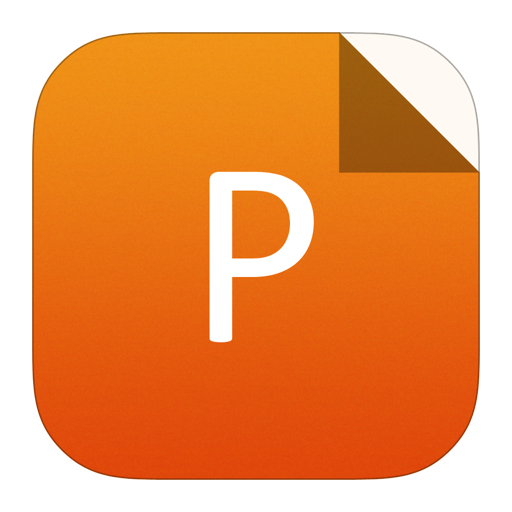
PowerPoint slide
Figure3.
(Color?online) Electron concentration in substrate under Vgs = 0.6 V.

class="figure_img" id="Figure2"/>
Download
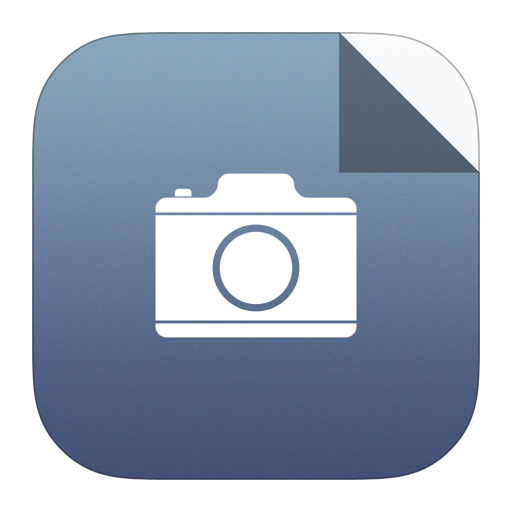
Larger image
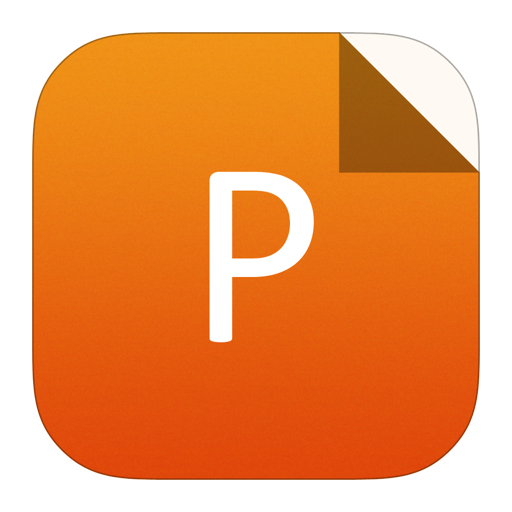
PowerPoint slide
Figure2.
(Color?online) Electron concentration in substrate under Vds = 0.6 V.
Increasing the electron injection with decreases in the substrate structural energies by increasing the arsenic concentration in the chemical composition of the substrate induce the decreases of characteristic device transconductance; consequently the drain current will be reduced, as shown in Figs. 4 and 5, the transconductance decreases from 1654.4, 1673.2, and 1685 mS/mm when x = 0.1 and Vds = 0.4 V, Vds = 0.6 V, and Vds = 0.8 V respectively to 998.3, 1010.6, and 1028 mS/mm under x = 0.7 and Vds = 0.4 V, Vds = 0.6 V and Vds = 0.8 V respectively as shown in Fig. 4. The obtained results of transconductance show that the increases of drain bias induce the increases of characteristic transconductance; this is due to the best conditions of confinement caused by the spacer/channel barrier, where it increases with the increasing of drain bias as shown in Fig. 4. In Figs. 6 and 7 we perform the curves of drain current as a function of drain voltage and gate voltage respectively. Also, in Fig. 8 where the curve of transconductance as a function of gate voltage is reported. These three figures are reported in order to present the consistency of obtained results. Similarly to Fig. 4, the drain current decreases with increasing the arsenic concentration in the substrate due to the electron injection phenomena, the drain current decreases when Vds = 1 V and x = 0.1 from 0.854, 3.018, and 3.419 mA/mm under Vgs = 0.4, Vgs = 0.6, and Vgs = 0.8 respectively to 0.43, 1.768, and 2.057 mA/mm respectively when x = 0.7 and under the same Vds bias.

class="figure_img" id="Figure5"/>
Download
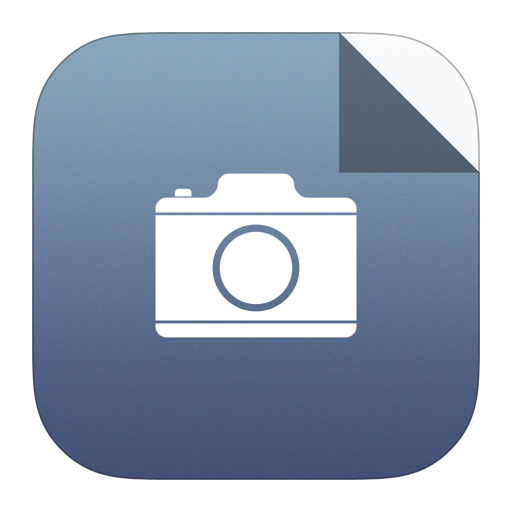
Larger image
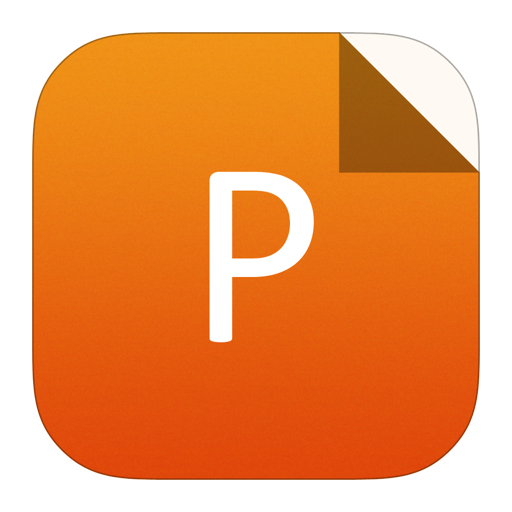
PowerPoint slide
Figure5.
(Color?online) Drain current as a function of substrate molar fraction in InAsxP1–x under Vds = 1 V.

class="figure_img" id="Figure6"/>
Download
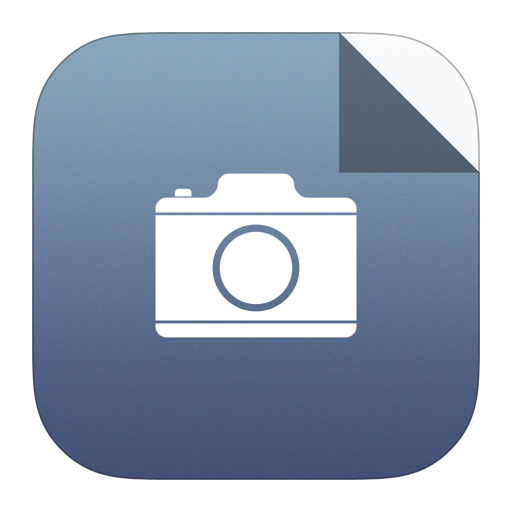
Larger image
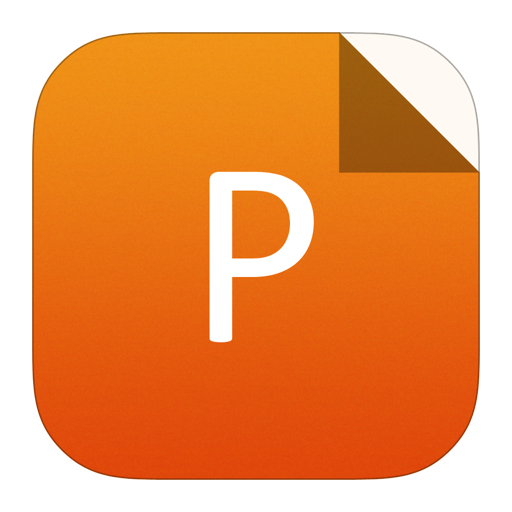
PowerPoint slide
Figure6.
(Color?online) Drain current as a function of drain voltage.

class="figure_img" id="Figure7"/>
Download
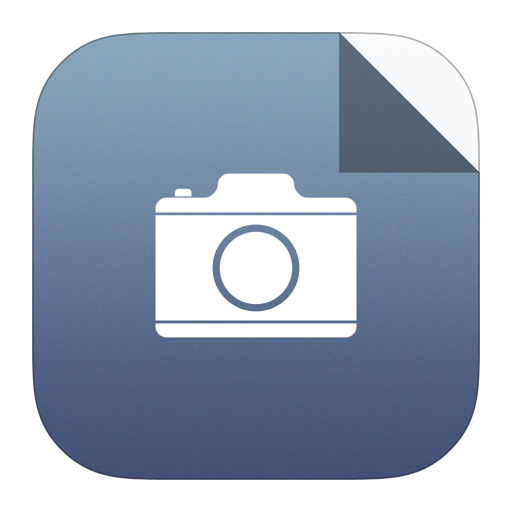
Larger image
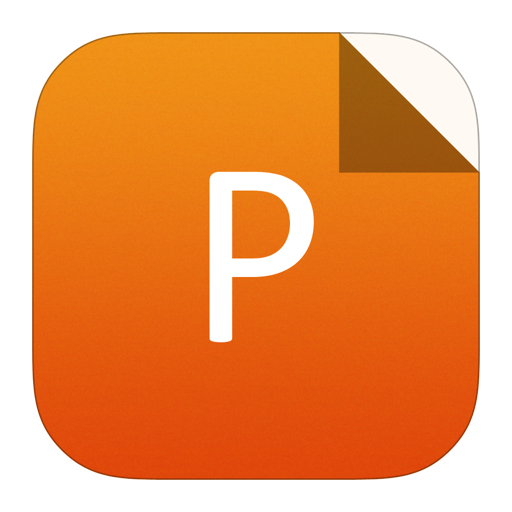
PowerPoint slide
Figure7.
(Color?online) Drain current as a function of gate voltage.

class="figure_img" id="Figure8"/>
Download
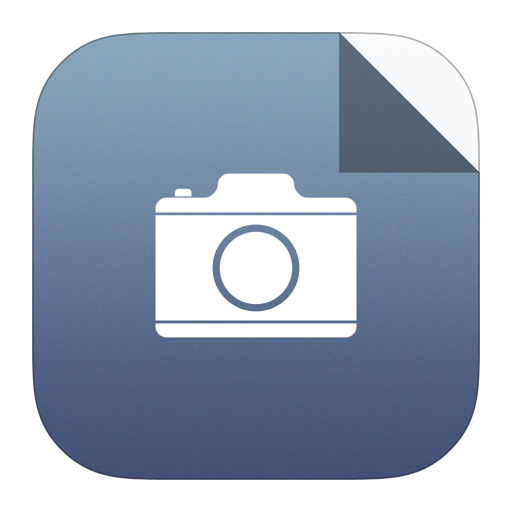
Larger image
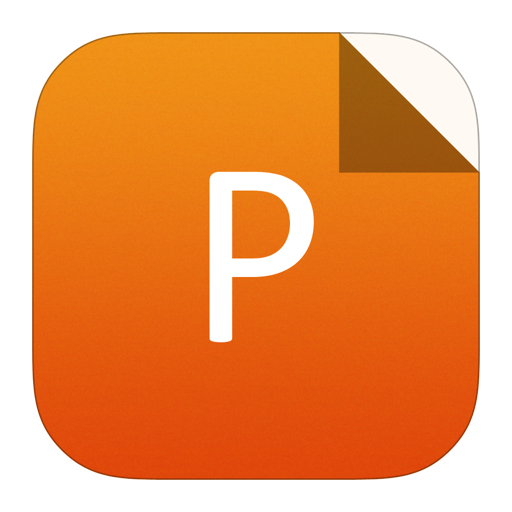
PowerPoint slide
Figure8.
Transconductance as a function of gate voltage.

class="figure_img" id="Figure4"/>
Download
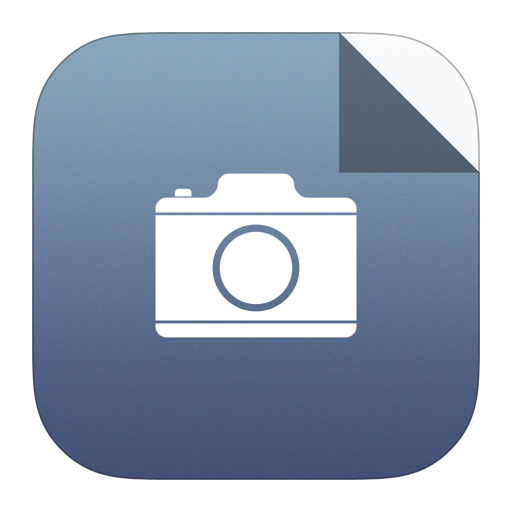
Larger image
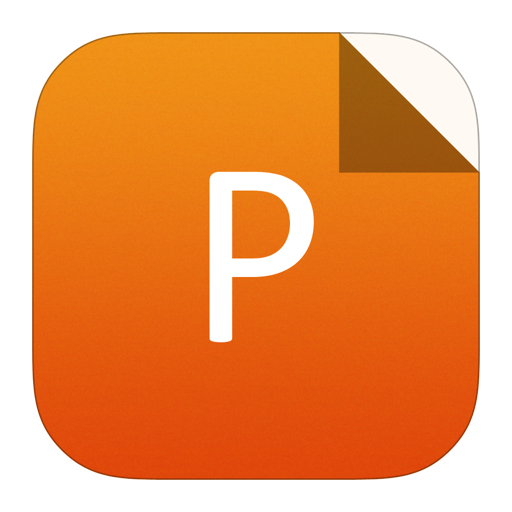
PowerPoint slide
Figure4.
(Color?online) Transconductance as a function of substrate molar fraction in InAsxP1–x.
5.
Conclusion
The obtained results for our study based on the drain current modulation as a function of substrate structural energies for an HEMT device using TCAD SILVACO at room temperature and the drift-diffusion model shows that the decreases of substrate structural energies by increasing the molar fraction induce the decreases of device drain current, and consequently the reported increases of electron injection towards the substrate region. This is presented as an advantageous conclusion such that it allows the production of an HEMT device which can operate with a low value of drain current. The reported novelty of this work has made the first HEMT that brings together the AC characteristic of an HEMT with a weak current, which is important in the field of bioengineering; it is characterized by a low signal amplitude and high frequency, and we can find this objective when we use an HEMT with low structural energies in its substrate.