1.
Introduction
Accompanying the development of III-nitrides materials and devices, increasing attention has been paid to the GaN-based resonate tunneling diode (RTD) because of its excellent properties such as wider direct band gap, larger band offset, higher peak electron velocity, higher critical breakdown voltage and higher thermal stability than those of GaAs/AlGaAs and Si/GeSi counterparts[1–10]. In particular, conduction band offsets in the InGaN/AlGaN system are larger than those in the InGaAs/AlGaAs system and a built-in electric field exists owing to the piezoelectric polarization effects, the former appeared to have very interesting negative differential resistance (NDR) related properties and became considerably attractive during the last decade[1, 3–5, 7, 8, 10]. RTD is one of the key candidates for enhancing the performance of multi-state memories (MSM) and multi-valued logic (MVL) circuits, generating THz signals, detecting THz signals, tuning RF systems and so on with reduced circuit complexity[3, 5–10].
However, the first NDR region of most GaAs and GaN-based RTD current–voltage curves reportedly consists of only a very narrow linear NDR or a very narrow linear NDR followed with an exponential tail and without a visible hysteresis loop, which is harmful to the logic stability of MSM and MVL circuits. The maximum total NDR region width (TNRW) of AlGaN/InGaN/AlGaN/InGaN/AlGaN/InGaN/AlGaN RTD on cubic GaN substrate reported by Yahyaoui et al. is observed at about 0.048 V with Al composite at 0.5[4]. The maximum TNRW of GaAs/AlGaAs ADBS RTD reported by Zhang et al. is observed at about 0.16 V with a 2.0 nm wide emitter barrier, 3.8 nm wide QW, 1.9 nm wide collector barrier, 0.35 Al composite of the emitter barrier and 0.5 Al composite of the collector barrier[6]. The maximum TNRW of GaN/AlGaN DBS RTD reported by Rached et al. is observed at about 0.25 V by considering the self-consistent effect and 0.48 V without considering the self-consistent effect with 2.0 nm thick QW, 1.0 nm thick symmetric barriers and 0.5 Al composite of barriers[7]. Sankaranarayanan and Saha proposed a GaN-based RTD with 2.0 nm thick QW and AlGaN barriers and 0.4 Al composite of barriers, which exhibits a giant peak valley current ratio (PVCR) as high as 60 and a very low valley current density of 3 mA/cm2 with a very sharp and narrow NDR region by using an InGaN layer modulating the collector barrier width[8]. Zhang et al. proposed a method to restrain polarization effects in GaN/AlGaN RTD by regulating the included angle ‘α’ between the normal vector of the original top surface on GaN substrate and c direction. The proposed method suggests that the TNRW varies with ‘α’ from about 0.11 to about 0.55 V as the original top surface on GaN substrate varies from
ight}$

To alleviate the above problem, a GaN/InGaN/AlGaN multi-quantum well (MQW) RTD was presented to both expand its TNRW and generate an obvious hysteresis loop. The non-equilibrium Green’s function (NEGF) method for solving the Schrodinger Equation was adopted to carry out simulation experiments of the proposed RTD on the TCAD platform. The obtained results indicate that its TNRW was expanded up to about 0.49 V and its NDR curve appears to have an obvious hysteresis loop at the same time, which is helpful to improve the stability of multi-logic states of MVL circuits. As the Al composite of its AlGaN barriers increases up to 0.33, its TNRW was further expanded up to about 0.60 V and the window width of the hysteresis loop increases from about 0.048 to about 0.177 V. These advantages are very helpful to further improve the stability of MVL states when it is utilized to design MSM and MVL circuits. What is more interesting is that the complement RTD pair or enhanced mode high electron mobility transistor (HEMT) controlled RTD and load RTD pair is capable of providing versatile multi-stable-state logic levels, which may be very attractive for versatile MSM and MVL related applications such as MVL gate circuits, MVL flip-flop and supply voltage programmable voltage-MVL convertor circuits and some other potential applications.
The paper is divided into 7 sections. In Section 2, the device structure of the proposed RTD is described including its interface polarization charge density and bound states distributions in equilibrium state. In Section 3, the proposed RTD is modeled with the coupled 1D Schrodinger and Poisson equations by considering the influence of polarization effects. In Section 4, the simulation experiments on the proposed RTD are presented in terms of the current–voltage characteristic and the influences of the Al composite ratio on its current-voltage characteristic including the hysteresis characteristic. In Section 5, possible MVLs provided by the proposed RTD are outlined. In Section 6, the influences of the Al composite ratio on the possible MVLs are analyzed and the mathematic type of the novel MVLs are defined, based on which some very interesting and attractive applications are pointed out. Finally, the conclusions of our study on the proposed RTD are generalized.
2.
Structure of GaN/InGaN/AlGaN MQW RTD
The cross-section view of the proposed GaN/InGaN/AlGaN MQW RTD on GaN substrate and the distributions of its interface polarization charge density and bound states are illustrated in Fig. 1. The original orientation of GaN substrate was chosen along the <0001> direction with the Ga surface. The proposed RTD was grown layer by layer in epitaxial technologies. The thickness of each layer is listed in Table 1. A 62 nm thick N+ doped GaN collector region was firstly grown on the GaN substrate. Then a 3.0 nm thick collector i-GaN (intrinsic GaN) spacer layer was secondly grown on the GaN collector region. After that Al0.3Ga0.7N/i-GaN/Al0.3- Ga0.7N DBS (double barriers) sandwich layers were thirdly grown in turn with each thickness at 1.0 nm. Then a 2.0 nm thick In0.03Ga0.97N layer was fourthly grown on the DBS sandwich, followed with a 3.0 nm thick i-GaN emitter spacer layer. Finally, a 62 nm thick N+ doped GaN emitter region was grown on the emitter spacer layer. Zubialevich et al. reported that a 0–20 nm thick GaN layer can be grown experimentally with a 3 × 2″ AIXTRON MOVPE reactor[11]. With the development of nano film growing technology, growing of 1.0 nm thickness AlGaN or InGaN film may also turn out to be realized in the future.
Region | Thickness (nm) |
n+-GaN emitter | 62 |
i-GaN spacer | 3.0 |
i-In0.03Ga0.97N | 2.0 |
i-Al0.3Ga0.7N | 1.0 |
i-GaN | 1.0 |
i-Al0.3Ga0.7N | 1.0 |
i-GaN spacer | 3.0 |
n+-GaN collector | 62 |
Table1.
Thickness of each layer.
Table options
-->
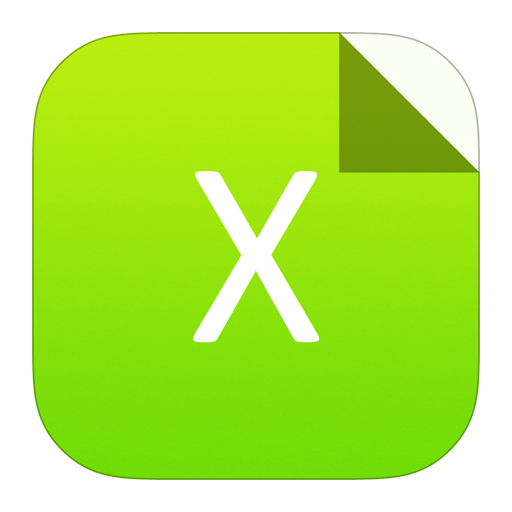
Download as CSV
Region | Thickness (nm) |
n+-GaN emitter | 62 |
i-GaN spacer | 3.0 |
i-In0.03Ga0.97N | 2.0 |
i-Al0.3Ga0.7N | 1.0 |
i-GaN | 1.0 |
i-Al0.3Ga0.7N | 1.0 |
i-GaN spacer | 3.0 |
n+-GaN collector | 62 |
The object of introducing the 2.0 nm thick In0.03Ga0.97N layer in between DBS sandwich and emitter spacer layers is to restrain the depletion extent of the emitter i-GaN spacer region since it acts as an electron reservoir. As a result, the proposed RTD is featured of a sandwich structure with triple heterogeneous junction barriers and double heterogeneous junction quantum wells, as illustrated in Fig. 1.
3.
Modelling of GaN/InGaN/AlGaN MQW RTD
As can be seen in Fig. 1, the conduction band edge appears declined and distorted in thermal equilibrium state owing to polarization effects of the GaN, AlGaN and InGaN epitaxial layers. As a result, the polarization effects lead to sheet polarization charges at each heterogeneous junction interface, which built the polarization field along the growth direction of the hetero-structures (z-axis). Along z-axis, the relationship between the vertical quantum transport of electron wave function
ight)$

$$ - frac{{{hbar ^2}}}{2}frac{partial }{{partial z}}left( {frac{1}{{mleft( z ight)}}frac{partial }{{partial z}}} ight)psi left( z ight) + left( {qVleft( z ight) + Delta {E_{ m C}}left( z ight)} ight)psi left( z ight) = Epsi left( z ight),$$ ![]() | (1) |
$$frac{partial }{{partial z}}left( {varepsilon left( z ight)frac{partial }{{partial z}}} ight)Vleft( z ight) = qleft( {{N_{ m D}}left( z ight) - nleft( z ight) + sigma left( z ight)} ight).$$ ![]() | (2) |
In Eqs. (1) and (2),
ight)$

ight)$

m C}}left( z
ight)$



ight)$

m D}}left( z
ight)$

ight)$

ight)$

$$sigma left( z ight) = sumlimits_{i ,, = ,, 1}^5 {{sigma _i}} delta left( {z - {z_{ m i}}} ight).$$ ![]() | (3) |
In Eq. (3),




class="figure_img" id="Figure1"/>
Download
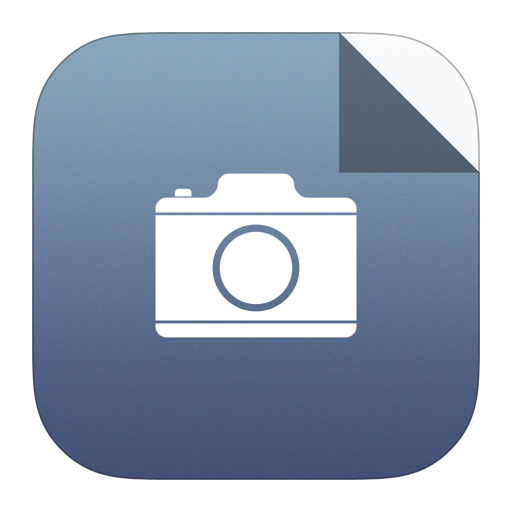
Larger image
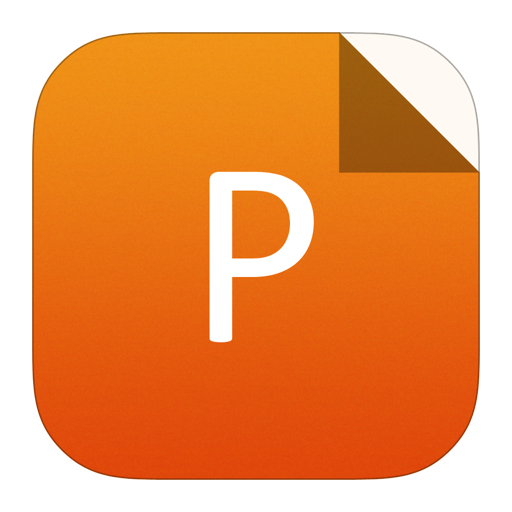
PowerPoint slide
Figure1.
(Color online) Cross-section view, interface polarization charge density and bound states distributions of the proposed GaN/InGaN/ AlGaN MQW RTD.
Since the thickness of the barrier layer is less than the wave length of a de Broglie wave, symmetrical and anti symmetrical states are generated through superposition of coherent states in the second and the last sub-bands.
4.
Simulation experiments on GaN/InGaN/AlGaN MQW RTD
Simulation experiments on GaN/InGaN/AlGaN MQW RTD were carried out with the NEGF method mentioned above. The simulated results are illustrated in Figs. 2–5. Among them, Fig. 2 demonstrates the current–voltage characteristic of the proposed GaN/InGaN/AlGaN MQW RTD. Fig. 3 shows definitions of the angle θ between the bottom side of the hysteresis loop and bias axis, the knee voltages and currents of hysteresis loop appeared in Fig. 2. The definitions of related characteristic voltages and currents are the same as in Ref. [6]. Fig. 4 illustrates the influence of the Al composite ratio on the BSE variations of the proposed GaN/InGaN/AlGaN MQW RTD. Fig. 5 illustrates the influence of the Al composite ratio on BSE distributions of the proposed GaN/InGaN/AlGaN MQW RTD.

class="figure_img" id="Figure3"/>
Download
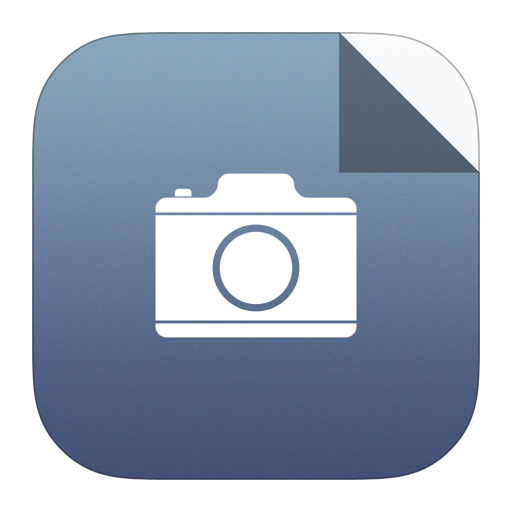
Larger image
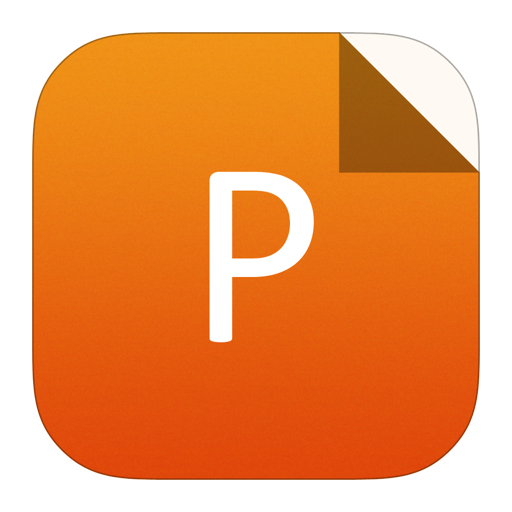
PowerPoint slide
Figure3.
(Color online) Key parameters definition of hysteresis loop in Fig. 2.
4.1
Current–voltage characteristic of the proposed RTD
As can be seen in Fig. 2, compared with the conventional GaN/AlGaN RTD, the current–voltage characteristic is observed with a wider NDR region width and tri-piecewise curve in the NDR region, which may be partitioned into three pieces:
(1) The first piece lies in [VP, Vknee1] at forward voltage variation or [VP, Vkneer2] at backward voltage variation and the current i decreases slowly with bias approximately in negative exponential as Eq. (4),
$$i = {I_{ m P}}exp left( { - frac{q}{{{k_0}T}}left( {{v_{ m {bias}}} - {V_{ m P}}} ight)} ight),$$ ![]() | (4) |
where VP is the peak voltage, which corresponds to the peak current IP.
Thus, we named it the first negative exponential NDR zone while the static resistance RDC and the dynamic NDR can be deduced respectively as Eqs. (5) and (6):
$${R_{ m {DC}}} = frac{{{v_{ m {bias}}}}}{i} = frac{{{v_{ m {bias}}}}}{{{I_{ m P}}}}exp left( {frac{q}{{{k_0}T}}left( {{v_{ m {bias}}} - {V_{ m P}}} ight)} ight) > 0,$$ ![]() | (5) |
$$begin{split}{ m {NDR}} = & frac{{{ m d}{v_{ m {bias}}}}}{{{ m d}i}} = - frac{{{k_0}T}}{{qi}} = - frac{{{k_0}T}}{{q{I_{ m P}}}}exp left( {frac{q}{{{k_0}T}}left( {{v_{ m {bias}}} - {V_{ m P}}} ight)} ight) = & - frac{{{k_0}T}}{q}frac{{{R_{ m {DC}}}}}{{{v_{ m {bias}}}}} < 0.end{split}$$ ![]() | (6) |
(2) The middle piece lies in [Vknee1, Vknee2] or [Vkneer2, Vkneer1] and the current decreases linearly and steeply with bias, thus we named it the constant NDR zone and its NDR may be expressed as Eq. (7):
$$begin{split} { m {ND{R_F}}} & = frac{{Delta {v_{{text{knee}}}}}}{{Delta {i_{{text{knee}}}}}} = frac{{{v_{{text{knee}}2}} - {v_{{text{knee}}1}}}}{{{i_{{text{knee}}2}} - {i_{{text{knee}}1}}}}; & approx ,, { m {ND{R_R}}} = frac{{Delta {v_{{text{kneer}}}}}}{{Delta {i_{{text{kneer}}}}}} = frac{{{v_{{text{kneer}}2}} - {v_{{text{kneer}}1}}}}{{{i_{{text{kneer}}2}} - {v_{{text{kneer}}1}}}}. end{split} $$ ![]() | (7) |
Therefore, the power efficiency difference of the hysteresis loop
m {hl}}}$

$$begin{split} {P_{{text{hl}}}}& approx Delta {v_{{text{FR}}}} frac{{Delta {i_{{text{knee}}}} + Delta {i_{{text{kneer}}}}}}{2}cos theta hfill & = frac{{Delta {v_{{text{knee}}}} + Delta {v_{{text{kneer}}}}}}{2} times frac{{Delta {i_{{text{knee}}}} + Delta {i_{{text{kneer}}}}}}{2}cos theta . end{split} $$ ![]() | (8) |
(3) The last piece lies in closed interval [Vknee2, VV] or [Vkneer1, VV] and the current decreases slowly as a short current tail. Thus, we named it as the last exponential NDR zone and its curve can be deduced out with the method similar to Eqs. (4)–(6).
The current density quantity of the proposed RTD is close to those reported in references[5, 7] and one order higher than that reported in Ref. [4]. Its PVCR = 6.17 is about 50% higher than those reported in Refs. [4, 5, 7] and about an order lower than that reported in Ref. [8]. While its NDR region width is about 1 time higher than that reported in Ref. [7] and more than 10 times higher than those reported in Refs. [4, 8]. What is more is that the proposed GaN/InGaN/AlGaN MQW RTD appears to have an obvious hysteresis characteristic in the linear NDR zone of its current–voltage curve at room temperature. No hysteresis characteristic in the linear NDR zone of GaN-based RTD’s current–voltage curve was reported in Refs. [3–10] at all. While Xiang Wei et al. reported a kind of InAs/AlSb/GaSb resonate inter-band tunneling diode (RITD) on InAs (001) substrate with the mesa of 20 × 20 μm2, which appears to have an obvious extrinsic bi-stability hysteresis loop at low temperatures around 77 K and none at room temperature[13].
4.2
Mechanism of electron transportation of the proposed RTD
As a bias voltage was applied between collector and emitter electrodes of the proposed RTD, separation, degeneration and jumping of the BSE levels will happen with increasing bias voltage and the variation law seems somewhat confused, as illustrated in Fig. 4. However, if only the bias voltage is big enough, current transportation was shouldered by electrons on those BSE levels across the conduction band bottom of the collector barrier layer with exciton assisted[14, 15]. Assume that
m j}}$

m j}}$


class="figure_img" id="Figure4"/>
Download
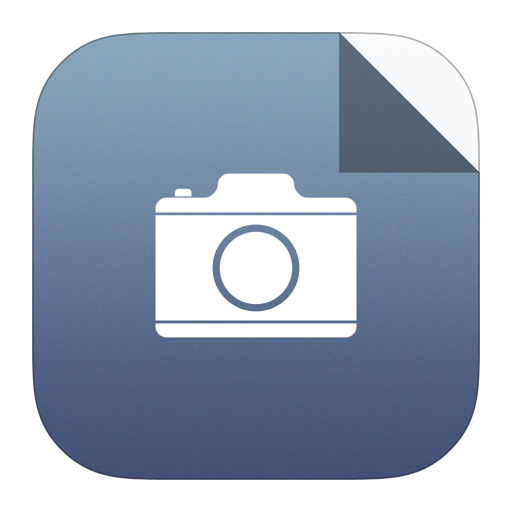
Larger image
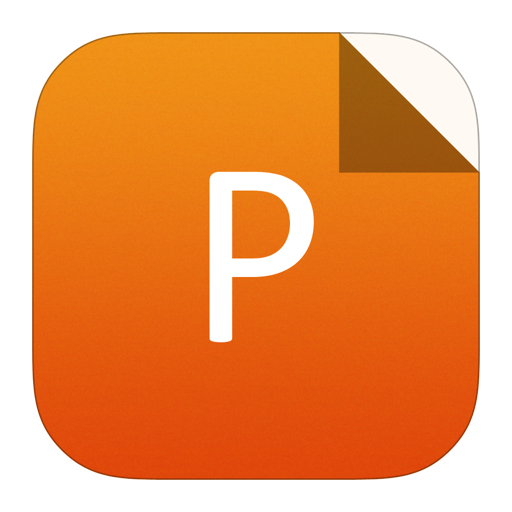
PowerPoint slide
Figure4.
(Color online) Separation, degeneration and jumping of the BSE levels vs. bias voltage.
$$Q = left{!!! {begin{array}{*{20}{c}} {1,qquad , {text{linear}};{text{energy}};{text{reducing}};{text{zone}},, quad quad quad ;} {2,qquad , {text{seperation}};{text{position}};{text{of}};{text{BSE}};{text{level}},quad ;,,} displaystyle{frac{1}{2},qquad {text{degeneration}};{text{position}};{text{of}};{text{BSE}};{text{level}}.;,} end{array}} ight.$$ ![]() | (9) |
m j}}-V_{
m {bias}}$


class="figure_img" id="Figure5"/>
Download
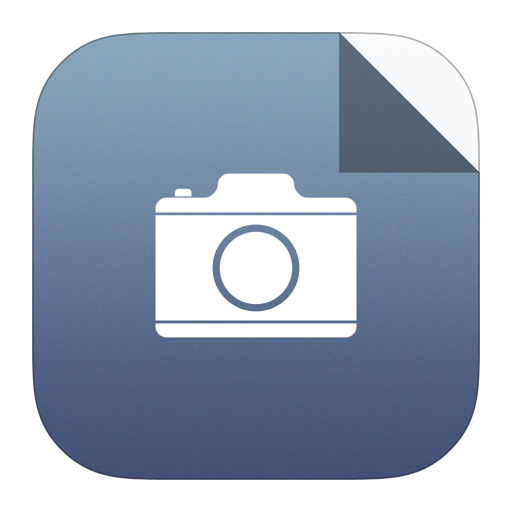
Larger image
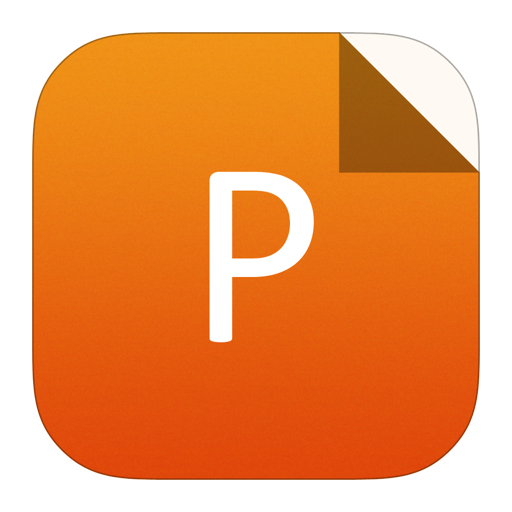
PowerPoint slide
Figure5.
(Color online) The BSE distribution of the proposed GaN/InGaN/AlGaN MQW RTD.
$$begin{split}{E_{ m j}} = & {E_{ m j0}} - sum {Delta {E_{ m j}}} - frac{1}{{{n_{ m j}}}}frac{{q{V_{ m {bias}}}}}{{{k_{ m B}}T}} hfill [-3pt]& times prod {{Q_{ m S}}Uleft( {{V_{ m bias}} - {V_{ m {bs}}}} ight){Q_{ m d}}Uleft( {{V_{ m {bias}}} - {V_{ m {bd}}}} ight)} . end{split} $$ ![]() | (10) |
Here
m j}}$

m j}}$

$$begin{split} {I_{ m {B1}}}left( t ight) = & ,,,, Afrac{{qm_{text{n}}^*{k_{text{B}}}T}}{{2{pi ^2}{hbar ^3}}}left{ {sumlimits_j {{T_{ m {B1j}}}left( {{E_{text{j}}}} ight)} } ight. hfill [-7pt] & times {left( {fleft( {{E_{text{j}}}} ight) - fleft( {{E_{text{j}}} + E_{{text{C}}2}^ + - E_{{text{C}}3}^ - } ight)} ight){N_{{text{ej,}};{text{InGaN}}}}} Bigggr}{20} ,end{split} $$ ![]() | (11) |
$$begin{split} {I_{{text{B}}2}}left( t ight) =, & ,, Afrac{{qm_{text{n}}^*{k_{text{B}}}T}}{{2{{text{p}}^2}{hbar ^3}}}sumlimits_j {left{ {{T_{{text{B2j}}}}left( {{E_{text{j}}}} ight)left[ {fleft( {{E_{text{j}}} + E_{{text{C}}2}^ + - E_{{text{C}}3}^ - } ight) - fleft( {{E_{text{j}}} + E_{{text{C}}3}^ + - E_{{text{C}}4}^ - } ight)} ight]} ight.} & times left( {{N_{{text{ej,, GaN}}}}left{ {1 - {T_{{text{B2j}}}}left( {{E_{text{j}}}} ight)left[ {fleft( {{E_{text{j}}} + E_{{text{C}}2}^ + - E_{{text{C}}3}^ - } ight) - fleft( {{E_{text{j}}} + E_{{text{C3}}}^{{text{ + }}} - E_{{text{C4}}}^ - } ight)} ight]} ight}} ight. & left. {left. { + {T_{{ m {B}}{text{1j}}}}left( {{E_{text{j}}}} ight)left[ {fleft( {{E_{text{j}}}} ight) - fleft( {{E_{text{j}}} + E_{{text{C}}2}^ + - E_{{text{C}}3}^ - } ight)} ight]{N_{{text{ej,;InGaN}}}}} ight)} ight}.end{split} $$ ![]() | (12) |
Here
m B1}}left( t
ight)$

m B2}}left( t
ight)$



m {Bij}}}left( {{E_{
m j}}}
ight)$

m j}}$

m {ej}}}$

m j}}$

$$left{ begin{gathered} T_{{text{B2j}}}^2left( {{E_{text{j}}}} ight)F_{2{text{j}}}^2 - left( {{N_{{text{ej,}};,{text{GaN}}}} + {T_{{text{B1j}}}}left( {{E_{text{j}}}} ight){F_{{text{1j}}}}{N_{{text{ej,}};;{text{InGaN}}}}} ight){T_{{text{B2j}}}}left( {{E_{text{j}}}} ight){F_{{text{2j}}}} + {T_{{text{B1j}}}}left( {{E_{text{j}}}} ight){F_{1{text{j}}}}{N_{{text{ej,}};;{text{InGaN}}}} = 0 ,hfill {F_{{text{1j}}}} = fleft( {{E_{text{j}}}} ight) - fleft( {{E_{text{j}}} + E_{{text{C}}2}^ + - E_{ m C3}^ - } ight) ,hfill {F_{2{text{j}}}} = fleft( {{E_{text{j}}} + E_{{text{C}}2}^ + - E_{{text{C}}3}^ - } ight) - fleft( {{E_{text{j}}} + E_{{text{C3}}}^{text{ + }} - E_{{text{C4}}}^ - } ight) .hfill end{gathered} ight.$$ ![]() | (13) |
Solve Eq. (13) and it will be found as Inequality (14):
$$0 < {T_{{text{B2j}}}}left( {{E_{text{j}}}} ight) = frac{{{N_{{text{ej,}};;{text{GaN}}}} + {T_{{text{B1j}}}}left( {{E_{text{j}}}} ight){F_{{text{1j}}}}{N_{{text{ej,}};;{text{InGaN}}}} pm sqrt {{{left( {{N_{{text{ej,}};;{text{GaN}}}} + {T_{{text{B1j}}}}left( {{E_j}} ight){F_{1{text{j}}}}{N_{{text{ej,}};;{text{InGaN}}}}} ight)}^2} - 4{T_{{text{B1j}}}}left( {{E_{text{j}}}} ight){F_{1{text{j}}}}{N_{{text{ej,}};;{text{InGaN}}}}} }}{{2{F_{{text{2j}}}}}} < 1$$ ![]() | (14) |
$$begin{split}& because frac{{{N_{{text{ej,GaN}}}} + {T_{{text{B1j}}}}left( {{E_{text{j}}}} ight){F_{{text{1j}}}}{N_{{text{ej,}};;{text{InGaN}}}}}}{{2{F_{2{text{j}}}}}} > 1 hfill & therefore {T_{{text{B2j}}}}left( {{E_{text{j}}}} ight) = frac{{{N_{{text{ej,}};{text{GaN}}}} + {T_{{text{B1j}}}}left( {{E_{text{j}}}} ight){F_{{text{1j}}}}{N_{{text{ej,}};;{text{InGaN}}}} - sqrt {{{left( {{N_{{text{ej,}};;{text{GaN}}}} + {T_{{text{B1j}}}}left( {{E_{text{j}}}} ight){F_{1{text{j}}}}{N_{{text{ej,}};;{text{InGaN}}}}} ight)}^2} - 4{T_{ m {B1j}}}left( {{E_{ m j}}} ight){F_{ m {1j}}}{N_{ m {ej,InGaN}}}} }}{{2{F_{2{text{j}}}}}} .hfill end{split} $$ ![]() | (15) |
Assume that
m e}}left( t
ight)$

m e}}left( { t}
ight)/q$

$$ - frac{{{Q_{text{e}}}left( t ight)}}{q} = sum {{N_{{text{ej,}};;{text{GaN}}}}(t) = sum {{N_{{text{ej,}};;{text{GaN}}}}} } + sum {left( {{T_{{text{B1j}}}}{F_{{text{1j}}}}{N_{{text{ej,}};;{text{InGaN}}}} - {T_{{text{B2j}}}}{F_{2{text{j}}}}{N_{{text{ej,}};;{text{GaN}}}}(t)} ight) } t.$$ ![]() | (16) |
Thus, the controlled charge density model and corresponding boundary conditions can be expressed as Eq. (17):
$$left{ {begin{array}{*{20}{c}} displaystyle { - frac{1}{q}frac{{{ m d}{Q_{text{e}}}}}{{{ m d}t}} = sum {{T_{{text{b1j}}}}{F_{1{text{j}}}}{N_{{text{ej,}};;{text{InGaN}}}} - sum {frac{{{T_{{text{B2j}}}}{F_{2{text{j}}}}{N_{{text{ej,}};;{text{GaN}}}}}}{{1 + {T_{{text{B2j}}}}{F_{{text{2j}}}}t}}} } - sum {frac{{{T_{{text{B2j}}}}{F_{{text{2j}}}}{T_{{text{b1j}}}}{F_{{text{1j}}}}{N_{{text{ej,}};;{text{InGaN}}}}t}}{{1 + {T_{{text{B2j}}}}{F_{2{text{j}}}}t}}} }, displaystyle{{{left. { - frac{1}{q}frac{{{ m d}{Q_{text{e}}}}}{{{ m d}t}}} ight|}_{t = 0}} = sum {left( {{T_{{text{B1j}}}}{F_{{text{1j}}}}{N_{{text{ej, InGaN}}}} - {T_{{text{B2j}}}}{F_{{text{2j}}}}{N_{{text{ej,GaN}}}}} ight), ,;qquad qquad qquad quad quad quad quad quad quad } quad} displaystyle{{{left. { - frac{1}{q}frac{{{ m d}{Q_{text{e}}}}}{{{ m d}t}}} ight|}_{t = infty }} = 0 , ,;;;qquad qquad qquad qquad qquad qquad qquad qquad qquad qquad qquad qquad qquad qquad quad} displaystyle{{Q_{text{e}}}left( 0 ight) = - q{N_{{text{ej,}};;{text{GaN}}}},,;,qquad qquad qquad qquad qquad qquad qquad qquad qquad qquad qquad qquad qquad quad , } displaystyle{{Q_{text{e}}}left( infty ight) leqslant - qsum {{N_{{ m C}{text{j}}}}quad {text{with}};{E_{text{j}}};{text{instead}};{text{of}};{E_{{text{j0}}}};{text{in}};{text{Eq.}};(9).qquad qquad qquad qquad qquad qquad qquad} } end{array}} ight.$$ ![]() | (17) |
Then
m e}}left( t
ight)$

$$begin{split} {Q_{text{e}}}left( t ight) = & - qleft[ {sum {left( {frac{{{T_{{text{b1j}}}}{F_{1{text{j}}}}{N_{{text{ej,}};;{text{InGaN}}}}}}{{{T_{{text{B2j}}}}{F_{{text{2j}}}}}} - {N_{{text{ej,}};;{text{GaN}}}}} ight)} } ight. hfill & times {ln left( {1 + {T_{{text{B2j}}}}{F_{{text{2j}}}}t} ight) + sum {{N_{{text{ej,}};;{text{GaN}}}}} } Bigggr]{16}. end{split} $$ ![]() | (18) |
If we let
m e}}left( {
m t}
ight) = - qsum {{N_{
m {Cj}}}} $

$$begin{split} {t_{min }} =& frac{1}{{{T_{{text{B2j}}}}{F_{{text{2j}}}}}} hfill & times left[ {exp left( {frac{{left( {sum {{N_{{text{Cj}}}} - sum {{N_{{text{ej,}};{mkern 1mu} ;{mkern 1mu} {text{GaN}}}}} } } ight)}}{{sum {left( {frac{{{T_{{text{b1j}}}}{F_{{text{1j}}}}}}{{{T_{{text{B2j}}}}{F_{{text{2j}}}}}}{N_{{text{ej,}};{mkern 1mu} ;{mkern 1mu} {text{InGaN}}}} - {N_{{text{ej,}};{mkern 1mu} ;{mkern 1mu} {text{GaN}}}}} ight)} }}} ight) - 1} ight]. hfill end{split} $$ ![]() | (19) |
As can be seen in Eq. (19) that the minimum time for electron to be full of quantum states in i-GaN QW at a given positive bias voltage appears to have an exponential relationship with the ratio between the initial concentration of empty quantum states and an increment of electron concentration per time in i-GaN QW. Here,
m {B2j}}}{F_{
m {2j}}}$

Therefore, the total electron accumulation velocity in i-GaN QW can be deduced as Eq. (20):
$${v_{{text{Acc}}}}left( t ight) = sum {frac{{{T_{{text{B1j}}}}{F_{{text{1j}}}}{N_{{text{ej,InGaN}}}} - {T_{{text{B2j}}}}{F_{{text{2j}}}}{N_{{text{ej,GaN}}}}}}{{1 + {T_{{text{B2j}}}}{F_{2{text{j}}}}t}}} .$$ ![]() | (20) |
Eqs. (16)–(20) characterize the electron accumulation effect in i-GaN QW at the given positive bias voltage[16], which makes the appearance of the hysteresis loop possible. Substitute Eq. (15) back into Eq. (11) or (12) and let

4.3
Influences of Al composite ratio on current–voltage characteristic of the proposed RTD
With the current–voltage characteristic of GaN/InGaN/ Al0.3Ga0.7N MQW RTD as the reference, influences of Al composite ration on its current–voltage characteristic were analyzed through simulation experiments by regulating the Al composite ratio of AlGaN barriers simultaneously only. The simulated results are illustrated in Figs. 6 and 7. As can be seen in Fig. 6 that decreasing the Al composite ratio of AlGaN barriers leads to obviously reduced VF, VP and VV, elevated IP and IV and a narrowed exponential NDR region, while increasing the Al composite ratio of AlGaN barriers leads to obviously elevated VF and VV, reduced IP and IV, a widened exponential NDR region and a narrowed linear NDR region. Fig. 7 illustrates the hysteresis characteristics of the proposed RTD with the Al composite ratio at 0.28 and 0.33 respectively. As can be seen from Figs. 2 and 7 that decreasing the Al composite ratio of AlGaN barriers leads to narrowing of the hysteresis loop and vice versa. Fig. 7(a) indicates that the hysteresis characteristics of the proposed RTD with Al composite ratio at 0.28 is ignorable and the relative variation of its total NDR region width is very little. While Fig. 7(b) indicates that the total NDR region width of the proposed RTD with the Al composite ratio at 0.33 is increased at about 50%.

class="figure_img" id="Figure6"/>
Download
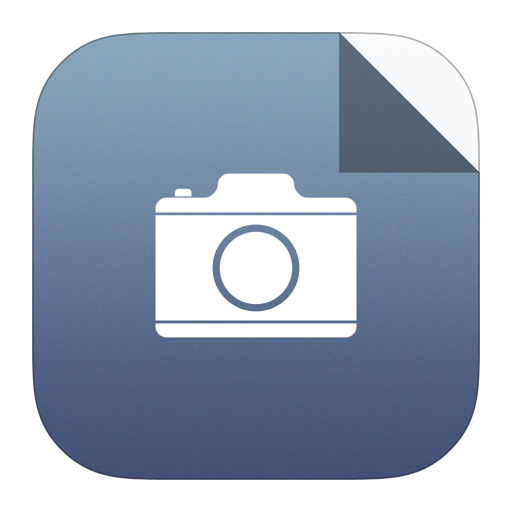
Larger image
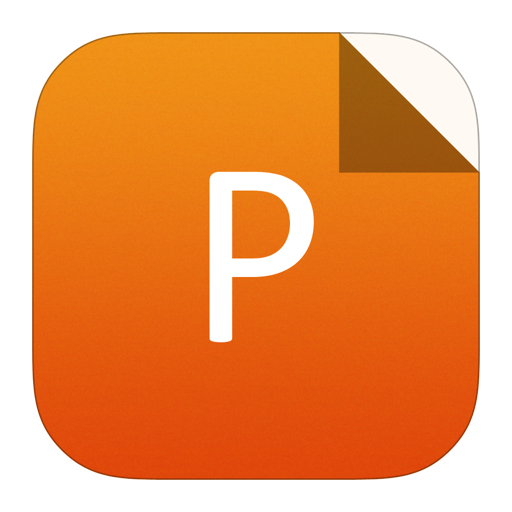
PowerPoint slide
Figure6.
Influence of Al composite ratio on current–voltage characteristic of the proposed RTD.

class="figure_img" id="Figure7"/>
Download
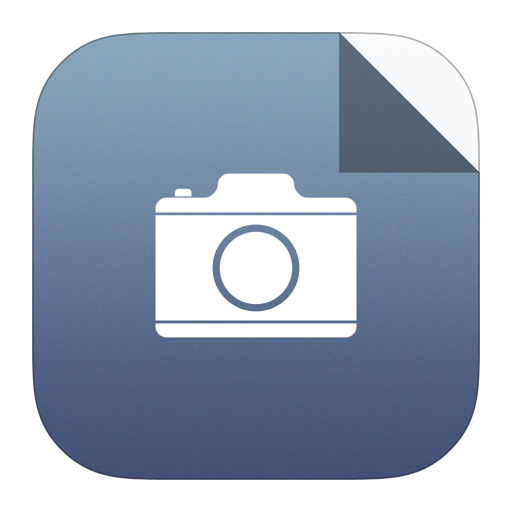
Larger image
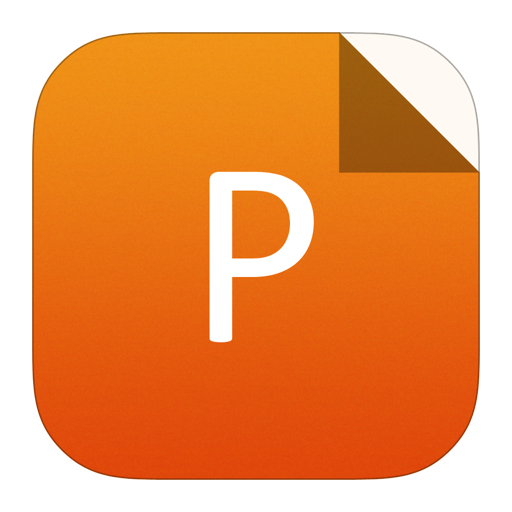
PowerPoint slide
Figure7.
Influence of Al composite ratio on the hysteresis characteristic of the proposed RTD. (a) Al composite ratio at 0.28. (b) Al composite ratio at 0.33.

class="figure_img" id="Figure2"/>
Download
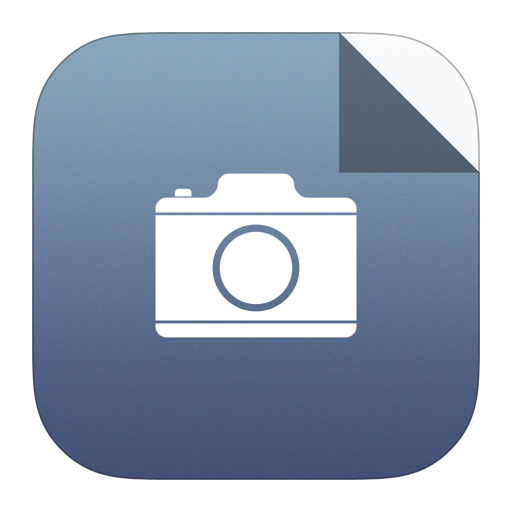
Larger image
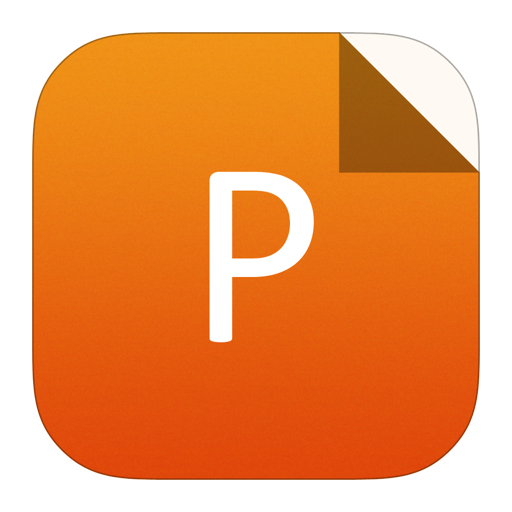
PowerPoint slide
Figure2.
(Color online) Current–voltage curve of the proposed RTD.
This advantage is very helpful to restrain the apparent positive resistance phenomenon and to improve the stability of MVL when it is utilized to design MVL circuits. This advantage is also very attractive for programmable and auto resistance compensation (PRC & ARC) applications.
5.
Possible MVLs provided by the proposed RTD and matched devices
By utilizing the nonlinear NDR characteristic of the proposed GaN-based RTD, the complement RTD pair (or a pair of corresponding RTD-enhanced mode high electron mobility transistor (HEMT) controlled RTD or RTHEMT[5]) is capable of generating one kind of stable 5-valued logic, 7-valued logic, one kind of weak stable 9-valued logic and 3 kinds of stable 3-valued logics at different supply voltages less than 3.3 V, as illustrated in Figs. 8(a)–8(f). Among them, as the current-voltage curve of the driver cuts the hysteresis loop of the current-voltage curve of the load RTD or vice versa, the corresponding logic states appear to have two different hysteresis middle logic values (HMLV) in different bias variation directions as illustrated in Figs. 8(a), 8(c), 8(e) and 8(f) due to the influence of the hysteresis characteristic. However, the cases of 7-valued logic illustrated in Fig. 8(a) and 9-valued logic illustrated in Fig. 8(f) are not practical due to their poor dynamic bias voltage scope to sustain stable logic states.

class="figure_img" id="Figure8"/>
Download
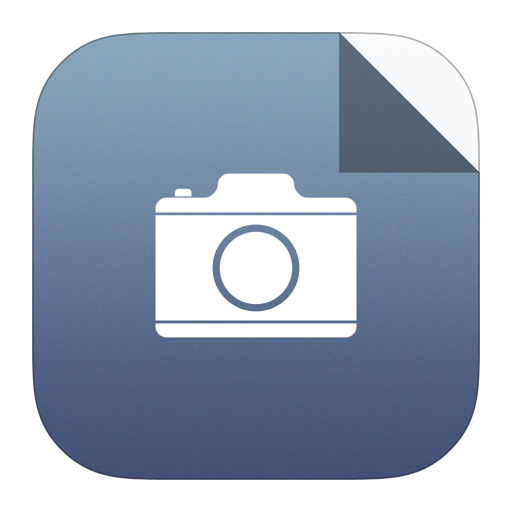
Larger image
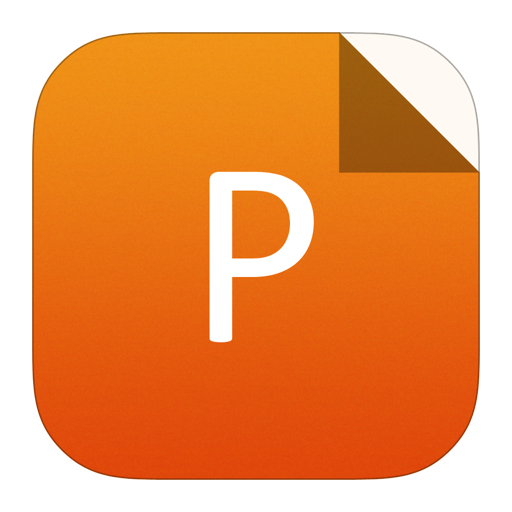
PowerPoint slide
Figure8.
(Color online) Possible MVL provided by the complement RTD pair with the proposed GaN-based RTD at different supply voltage. (a) 7-valued logic levels at about 1.84 V supply voltage. (b) 3-valued logic levels at about 2.0 V supply voltage. (c) 3-valued logic levels with different middle logic values at the window edges of hysteresis loop at about 2.35 V supply voltage. (d) 3-valued logic levels at about 2.6 V supply voltage. (e) 5-valued logic levels at about 3.0 V supply voltage. (f) 9-valued logic levels at about 3.1 V supply voltage.
6.
Influences of Al composite ratio on MVL characteristics of the proposed complement RTD pair
With the current–voltage characteristic of GaN/InGaN/ Al0.3Ga0.7N MQW RTD as the reference, influences of Al composite ratio on MVL characteristics of the proposed complement RTD pair were analyzed through drive-load matching, as illustrated as Figs. 9 and 10.
Fig. 9 illustrates the flexible MVL characteristics realized by the proposed complement RTD pair with the barrier layer Al composite ratio of the proposed RTD at 0.28. Fig. 9(a) is similar to Fig. 6(a), Fig. 9(b) is similar to Fig. 6(d) and Fig. 9(c) is similar to Fig. 6(e). The MVL characteristics similar to Figs. 6(b), 6(c) and 6(f) disappear since the hysteresis characteristic of the proposed RTD with Al composite ratio at 0.28 is ignorable and its first exponential NDR zone is shorted into being approximately linear.

class="figure_img" id="Figure9"/>
Download
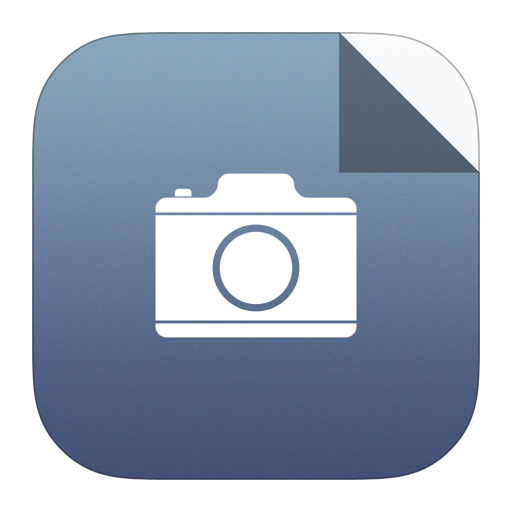
Larger image
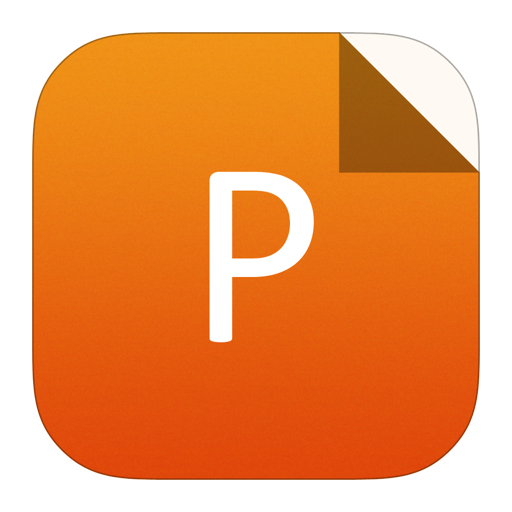
PowerPoint slide
Figure9.
(Color online) Flexible MVL characteristics of the proposed complement GaN/InGaN/AlGaN MQW RTD pair with its barrier layer Al composite ratio at 0.28. (a) 7-valued logic levels at about 1.62 V supply voltage. (b) 3-valued logic levels at about 2.3 V supply voltage. (c) 3-valued logic levels at about 2.6 V supply voltage.
Figs. 10(a)–10(p) illustrate the flexible MVL characteristics and related critical conditions realized by the proposed complement RTD pair with the barrier layer Al composite ratio of the proposed RTD at 0.33 as supply voltage increases in the load-pull method. The type of MVL and its stability of each case were outlined in Table 2 at the corresponding supply voltage, in which
$${ m{Edge}}leftlangle {x|y} ight angle , $$ ![]() | (21) |
represents the number ‘


$${V_{ m {m}l}} = frac{{{V_{ m S}}}}{2} . $$ ![]() | (22) |
Besides, P represents the output characteristic of the corresponding driving RTD or enhanced mode HEMT/RTD varies with increasing bias voltage (positive edge) and N represents the output characteristic of the corresponding driving RTD or enhanced mode HEMT/RTD varies with decreasing bias voltage (negative edge). As can be seen in Fig. 8 and Table 2, it is very attractive that the type of MVLs and its stability of the proposed complement RTD pair vary with supply voltage in the range of [1.75 V, 3.38 V]. The logic voltage value also varies with supply voltage in the range of [1.75 V, 3.38 V] simultaneously as outlined inTable 2.
From Fig. 8 to Fig. 10 and Table 2 it can be deduced that the proposed RTD with the obvious hysteresis loop is suitable for some very interesting and attractive applications as follows:
Graph order in Fig. 10 | Type of MVLs | Supply voltage scope (V) | Logic voltage scope (V) | Logic stability |
(a) | Tangle peak | 1.75 | [0.79, 0.97] | unstable |
(b) | Low normal 3-value-logic | 1.75–1.86 | (0.73,0.79), (0.875,0.93), (0.97,1.13) | stable |
(c) | Edge $leftlangle {2|1} ight angle $4-value-logic | 1.86 | P:0.62, 0.73, 0.93, 1.12, N:1.23 | unstable |
(d) | Edge $leftlangle {3|1} ight angle $5-value-logic | 1.86–1.96 | P:(0.59,0.62),P:(0.62,0.71),(0.71,0.73),(0.93,0.98), N:(1.12,1.23),N:(1.23,1.37) | stable |
(e) | Edge $leftlangle {2|2} ight angle $5-value-logic | 1.96 | 0.59, N:0.71, 0.98, P:1.23, 1.37 | unstable |
(f) | Edge $leftlangle {1|3} ight angle $5-value-logic | 1.96–2.08 | (0.59,0.60), N:(0.70,0.71), (0.98,1.04),P:(1.23,1.37), (1.38,1.47) | stable |
(g) | Edge $leftlangle {1|2} ight angle $4-value-logic | 2.08 | 0.60, N:0.70, 1.04, P:1.37, 1.47 | unstable |
(h) and (i) | top normal 3-value-logic | 2.08–2.46 | (0.60,0.66], (1.04,1.23], (1.47,1.82] | stable |
(i), (j) and (k) | Edge $leftlangle {1|2} ight angle $3-value-logic | 2.46–2.78 | [0.66, 0.73], N/P:[1.23, 1.39], [1.82, 2.05] | stable |
(l) | Edge $leftlangle {2|2} ight angle $5-value-logic | 2.78–3.16 | (0.73,0.83), N:1.23, P:1.37, (1.39,1.58),N:(1.39,1.78), P:(1.39,1.91), (2.05,2.32) | stable |
(m) | Edge $leftlangle {3|3} ight angle $7-value-logic | 3.16 | 0.83,1.04, N:1.23, P:1.37, 1.58,N:1.78, P:1.91, 2.17,2.32 | unstable |
(n) | Edge $leftlangle {4|4} ight angle $9-value-logic | 3.16–3.25 | (0.83,0.87), (0.87,1.04), (1.04,1.23), N:1.23P:1.37, (1.58,1.645), N:(1.78,1.88), P:(1.91,2.06), (2.06,2.17), (2.17,2.31), (2.31,2.32) | stable |
(o) | Edge $leftlangle {3|2} ight angle $6-value-logic | 3.25 | 0.87, 1.23, P:1.37, 1.645, N:1.88, 2.06, 2.31 | unstable |
(p) | Edge $leftlangle {2|0} ight angle $3-value-logic | 3.25–3.38 | P:(1.23,1.36), P:(1.36,1.37), (1.645,1.69),N:(1.88,1.98), N:(1.98,2.06) | stable |
_ | Edge $leftlangle {1|0} ight angle $2-value-logic | 3.38 | P:1.36, 1.69, N:1.98 | unstable |
Table2.
The flexible MVL characteristics of the proposed complement RTD pair with its barrier layer Al composite ratio at 0.33.
Table options
-->
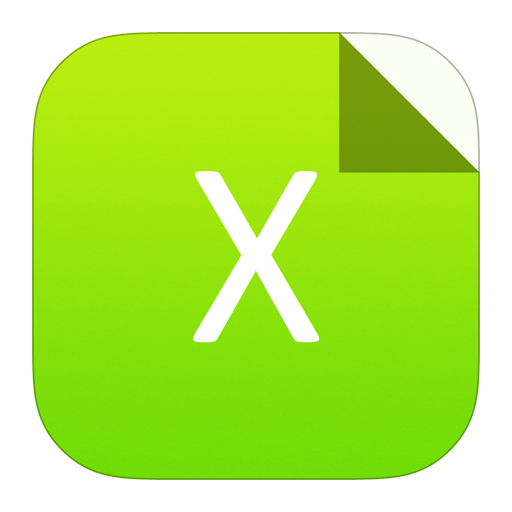
Download as CSV
Graph order in Fig. 10 | Type of MVLs | Supply voltage scope (V) | Logic voltage scope (V) | Logic stability |
(a) | Tangle peak | 1.75 | [0.79, 0.97] | unstable |
(b) | Low normal 3-value-logic | 1.75–1.86 | (0.73,0.79), (0.875,0.93), (0.97,1.13) | stable |
(c) | Edge $leftlangle {2|1} ight angle $4-value-logic | 1.86 | P:0.62, 0.73, 0.93, 1.12, N:1.23 | unstable |
(d) | Edge $leftlangle {3|1} ight angle $5-value-logic | 1.86–1.96 | P:(0.59,0.62),P:(0.62,0.71),(0.71,0.73),(0.93,0.98), N:(1.12,1.23),N:(1.23,1.37) | stable |
(e) | Edge $leftlangle {2|2} ight angle $5-value-logic | 1.96 | 0.59, N:0.71, 0.98, P:1.23, 1.37 | unstable |
(f) | Edge $leftlangle {1|3} ight angle $5-value-logic | 1.96–2.08 | (0.59,0.60), N:(0.70,0.71), (0.98,1.04),P:(1.23,1.37), (1.38,1.47) | stable |
(g) | Edge $leftlangle {1|2} ight angle $4-value-logic | 2.08 | 0.60, N:0.70, 1.04, P:1.37, 1.47 | unstable |
(h) and (i) | top normal 3-value-logic | 2.08–2.46 | (0.60,0.66], (1.04,1.23], (1.47,1.82] | stable |
(i), (j) and (k) | Edge $leftlangle {1|2} ight angle $3-value-logic | 2.46–2.78 | [0.66, 0.73], N/P:[1.23, 1.39], [1.82, 2.05] | stable |
(l) | Edge $leftlangle {2|2} ight angle $5-value-logic | 2.78–3.16 | (0.73,0.83), N:1.23, P:1.37, (1.39,1.58),N:(1.39,1.78), P:(1.39,1.91), (2.05,2.32) | stable |
(m) | Edge $leftlangle {3|3} ight angle $7-value-logic | 3.16 | 0.83,1.04, N:1.23, P:1.37, 1.58,N:1.78, P:1.91, 2.17,2.32 | unstable |
(n) | Edge $leftlangle {4|4} ight angle $9-value-logic | 3.16–3.25 | (0.83,0.87), (0.87,1.04), (1.04,1.23), N:1.23P:1.37, (1.58,1.645), N:(1.78,1.88), P:(1.91,2.06), (2.06,2.17), (2.17,2.31), (2.31,2.32) | stable |
(o) | Edge $leftlangle {3|2} ight angle $6-value-logic | 3.25 | 0.87, 1.23, P:1.37, 1.645, N:1.88, 2.06, 2.31 | unstable |
(p) | Edge $leftlangle {2|0} ight angle $3-value-logic | 3.25–3.38 | P:(1.23,1.36), P:(1.36,1.37), (1.645,1.69),N:(1.88,1.98), N:(1.98,2.06) | stable |
_ | Edge $leftlangle {1|0} ight angle $2-value-logic | 3.38 | P:1.36, 1.69, N:1.98 | unstable |
Design versatile MVL circuits and systems based on those MVLs illustrated in Fig. 8 and Fig. 10.
Design hysteresis MVL circuits and comparators with very few transistors, which are characterized stable HMLV states.
Design programmable voltage-MVL convertor circuits by combining with a programmable controlled discreet amplitude pulse power supply and an MVL latch.
Design super high speed linear or nonlinear parallel ADC by combining with super high speed sample-hold circuit and an MVL latch.
Design analogue or pulse voltage amplitude sensors in a similar principle to those mentioned above.
Design segmental function hysteresis arithmetic operation circuits by combining with integrated OPAMP when negative exponential operation, negative linear operation and/or parabolic operation are necessary simultaneously.
Design mixed MVL and arithmetic operation circuits and systems by synthesizing the MVL circuits and systems, and the analogue circuits and systems mentioned above.
7.
Conclusion
In this paper, we proposed a GaN/InGaN/AlGaN MQW RTD. The proposed RTD is characterized by a 1D MQW structure with stacked GaN/InGaN/AlGaN/GaN/AlGaN/GaN multi-layers. As the Al composite ratio of the double barriers of the proposed RTD drops in [0.28, 0.33], its current-voltage characteristic curve is featured of a tri-piecewise NDR region, which consists of a linear NDR zone sandwiched with two negative exponential NDR zones. While the Al composite ratio of the double barriers of the proposed RTD drops in [0.3, 0.33], its linear NDR zone appears to have enough of an obvious hysteresis loop. If the Al composite ratio of the double barriers of the proposed RTD increases continuously, its NDR region will degenerate into a bi-piecewise curve little by little.
As comparing with those conventional GaN-based RTDs, the TNRW of the proposed RTD was improved 1 to 14 times. Both the increased TNRW and hysteresis loop window width are beneficial to improving the stability of multi-logic states of multi-valued logic circuits by designing with the proposed GaN-based RTD. Moreover, based on the tri-piecewise NDR characteristic and hysteresis characteristic in the linear NDR zone of the proposed RTD, it is very suitable for versatile MVL applications such as MVL circuits and systems based on those MVLs illustrated in Fig. 8 and Fig. 10, MVL circuits and comparators with very few transistors, which are characterized by stable HMLV states, programmable voltage-MVL convertor circuits by combining with a programmable controlled discreet amplitude pulse power supply and an MVL latch, analogue voltage amplitude sensors by combining with super high speed sample-hold circuit and an MVL latch, pulse voltage amplitude sensors in the similar principle, super high speed linear or nonlinear parallel ADC by combining with a super high speed sample-hold circuit and an MVL latch, and mixed MVL and arithmetic operation circuits and systems, by synthesizing the MVL circuits and systems, and analogue circuits and systems based on the proposed RTD.

class="figure_img" id="Figure10"/>
Download
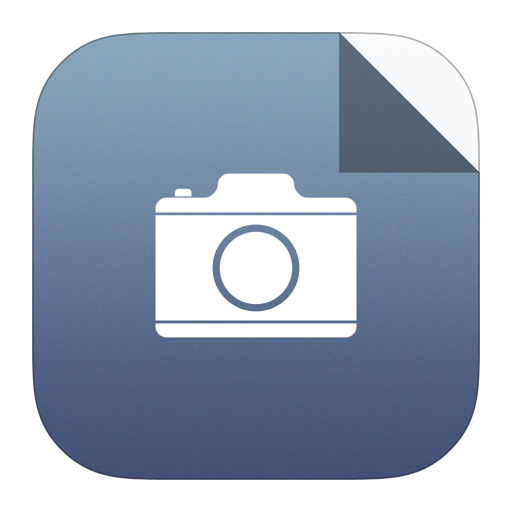
Larger image
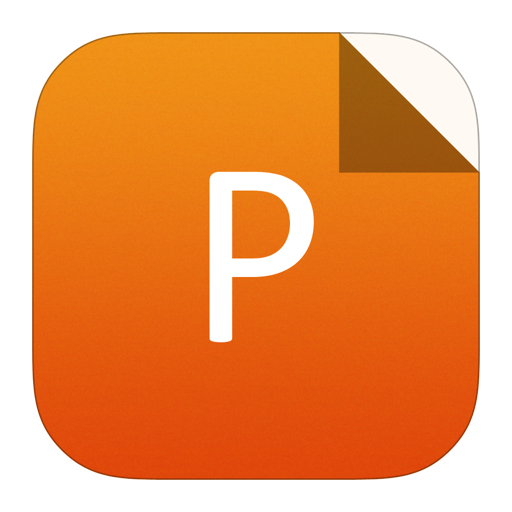
PowerPoint slide
Figure10.
(Color online) Flexible MVL characteristics of the proposed complement GaN/InGaN/AlGaN MQW RTD pair with its barrier layer Al composite ratio at 0.33 (outlined in Table 2). (a) Tangle peak. (b) Low normal 3-value-logic. (c) Edge
ight
angle $
ight
angle $
ight
angle $
ight
angle $
ight
angle $
ight
angle $
ight
angle $
ight
angle $
ight
angle $
ight
angle $
ight
angle $
ight
angle $
Acknowledgements
The authors acknowledge the support of Silvaco TCAD by Silvaco China.