1.
Introduction
Silicon quantum dots (Si QDs) are usually crystalline Si nanoparticles that are no more than ~10 nm large[1–4]. The quantum confinement of Si QDs causes them to be quasi-direct-bandgap semiconductors, in which the excitation and recombination of carriers may not always require the participation of phonons[1, 5–9]. Therefore, Si QDs exhibit remarkable optical absorption and emission[2, 8, 10–12], in stark contrast with other Si structures (e. g., bulk Si). Especially, the efficient light emission from Si QDs has been inspiring researchers to pursue high-performance Si light sources such as light-emitting diodes (LEDs) and even lasers.
Si QDs may be present in porous Si[13, 14], wide-bandgap matrices (e. g., SiO2, Si3N4 and SiC)[12, 15–21] or simply freestanding[22–24]. In recent years freestanding Si QDs have been gaining increasing popularity due to their easily accessible surface and flexible incorporation into all kinds of structures and devices[25–37]. For the use of freestanding Si QDs they are often dispersed in solvents to form colloids, which facilitates solution processing. It is well known that solution processing is critical to the fabrication of printed electronics[38–53]. Colloidal Si QDs actually hold great promise for the development of Si-based printed electronics.
Given the intriguing perspective of Si-QD-based light-emitting devices, colloidal Si QDs have been employed to fabricate LEDs. These LEDs signify that colloidal Si QDs can indeed play an important role in printed electronics. In this review we first describe the preparation of colloidal Si QDs by using surface modification. All kinds of LEDs based on colloidal Si QDs are then introduced. The improvement of the LED performance by the tuning of the QD surface and size and the device structure is discussed in detail. We finally present a brief outlook on the development of LEDs based on colloidal Si QDs.
2.
Colloidal Si QDs
As-produced freestanding Si QDs are usually passivated by hydrogen (H-Si QDs)[54–56]. The hydrogen-passivated surface does not enable the dispersion of Si QDs in either organic solvents or water. Therefore, surface modification should be carried out to render Si QDs soluble in solvents. One of the most popular surface modification schemes of Si QDs is hydrosilylation, which often replaces H atoms with hydrocarbon ligands at the QD surface[57]. Fig. 1 schematically shows the hydrosilylation of Si QDs with 1-dodecene. After, the hydrosilylation dodecyl ligands are attached to the QD surface, leading to the excellent dispersion of Si QDs in various organic solvents[58].

class="figure_img" id="Figure1"/>
Download
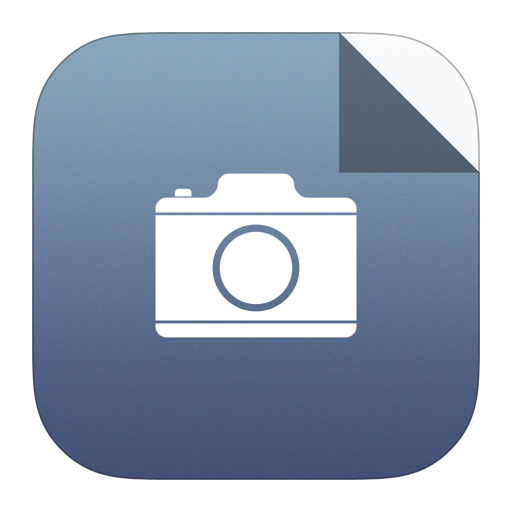
Larger image
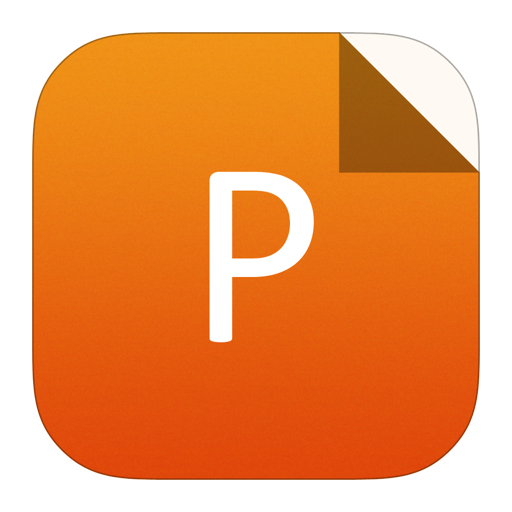
PowerPoint slide
Figure1.
(Color online) Schematic of the typical process of hydrosilylation for Si QDs.
The hydrosilylation can be mediated by heat, UV light or radicals[59–63]. Alkene is the most widely employed in hydrosilylation. Jurbergs et al.[64] carried out 1-octadecene hydrosilylation for H–Si QDs. The emission peak of their samples ranged from 700 to 800 nm. They observed the highest photoluminescence (PL) quantum yield (QY) of 62% for hydrosilylated Si QDs at the emission wavelength of ~789 nm. Greben et al. have recently shown that the 1-dodecene hydrosilylation for H-Si QDs may also lead to the PL QY of ~60% for the light emission from Si QDs at the wavelength of ~826 nm[64]. Purkait et al.[59] carried out hydrosilylation for H–Si QDs by using 1-alkene with varying chain length (C5 –C12). It was found that the emission peak of different samples remained the same regardless of the chain length, while the surface coverage of alkyl ligands was higher for Si QDs capped with shorter ligands. They concluded that functionalization was more effective with shorter ligands. Liu et al.[5] compared the PL QY of Si QDs hydrosilylated with 1-dodecene and 1-hexene. They pointed out that PL QY was higher for Si QDs with shorter ligands. Besides alkene, phenyl-containing olefins such as styrene and allylbenzene were also used in hydrosilylation. Yang et al.[65] and Mastronardi et al.[66] used styene and allylbenzene (AB) for hydrosilylation, respectively. The PL lifetime of their samples was in the order of a microsecond, comparable to their counterparts capped by alkyl ligands. Rinck et al.[67] carried out hydrosilylation with allylphenylsulfide (APS) for H–Si QDs. They found the overall PL QY could increase by 20% compared with that for AB-hydrosilylated Si QDs. This is because the vibration frequency of C–S bonds in APS is lower than that of C–C bonds in AB, leading to the reduced non-radiative vibrational relaxation in APS-hydrosilylated Si QDs.
The hydrosilylation can also be conducted by a combination of a short-chain alkyne and a long-chain alkene. Jariwala et al.[68] passivated the surface of Si QDs via in situ gas-phase hydrosilylation using the combination of acetylene and styrene, as shown in Fig. 2. They found that the surface coverage could increase to 50% after the combined hydrosilylation, whereas the maximum surface coverages were merely ~38% and ~30% in the styrene hydrosilylation and acetylene hydrosilylation, respectively. Their results indicated that the combined short-chain alkyne and long-chain alkene hydrosilylation enhanced the surface passivation of Si QDs. In a later work, Weeks et al.[63] used phenylacetylene (PA) instead of styrene in the combined hydrosilylation. The surface coverage was further enhanced for acetylene/PA hydrosilylated Si QDs, compared with acetylene/styrene hydrosilylated Si QDs. This was due to the fact that the van de Waals radius of an alkenyl chain was smaller than that of an alkyl chain.

class="figure_img" id="Figure2"/>
Download
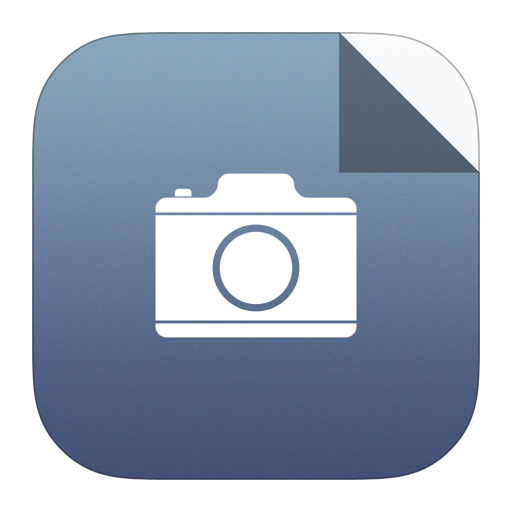
Larger image
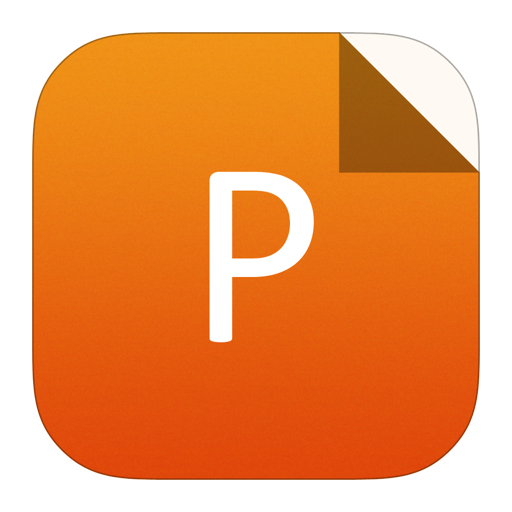
PowerPoint slide
Figure2.
(Color online) Gas-phase hydrosilylation of Si QDs using styrene and acetylene.
For hydrosilylated Si QDs, the PL energy, PL QY and PL lifetime all depend on the size of Si QDs. It has been shown that the PL energy increases with the decrease of the Si QD size[69, 70]. The PL QY was observed to decrease with the increase of the Si QD size in the wavelength region close to 1100 nm[69]. However, Mastronardi et al.[70] found that the PL QY increased with the increase of the Si QD size in the wavelength region from 600 to 800 nm. Liu et al.[5] have recently carried out hydrosilylation by using 1-dodecene for Si QDs with the sizes from 2 to10 nm. They showed that the PL energy (bandgap) of Si QDs was only associated with the quantum confinement effect and complied with an effective mass approximation (EMA), as shown in Fig. 3(a). The PL QY was in fact determined by the interplay between the quantum confinement effect and surface effect. Therefore, there existed an optimum PL QY at a certain Si QD size, as shown in Fig. 3(b). The PL lifetime monotonically decreases from more than one thousand microseconds to about ten microseconds with the decrease of the QD size from ~10 to 2 nm, as shown in Fig. 3(c).

class="figure_img" id="Figure3"/>
Download
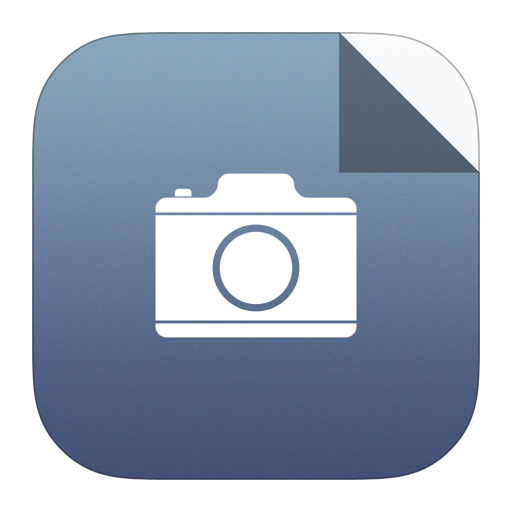
Larger image
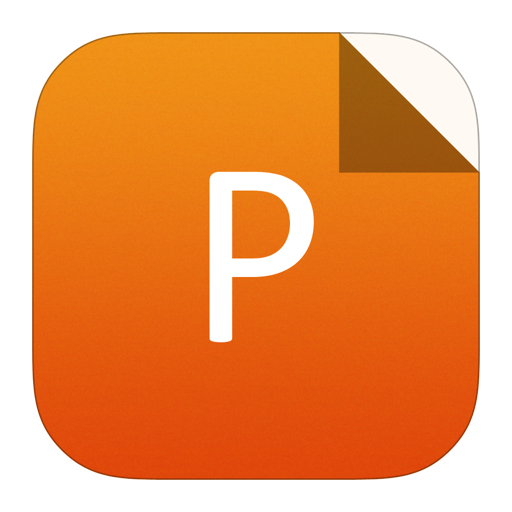
PowerPoint slide
Figure3.
(Color online) (a) Dependence of the PL energy (bandgap) on the size of dodecene-hydrosilylated Si QDs. (b) PL QY of a series of dodecene-hydrosilylated Si QDs with different sizes. (c) Dependence of the PL lifetime on the bandgap for dodecene-hydrosilylated Si QDs.
It has been often shown that the near-infrared to green light emission of Si QDs originates from quantum-confined excitons in Si QDs, while the blue emission of Si QDs was associated with surface defects. Dasog et al.[61] converted the light emission of H–Si QDs from red to blue upon exposure to nitrogen-containing reagents such as tetraoctylammonium bromide (TOAB) and ammonium bromide (NH4Br). They found that the PL lifetime of Si QDs emitting blue light was in the range of nanoseconds. The presence of trace nitrogen and oxygen in Si QDs gave rise to the blue emission of Si QDs. In their later work[71], they tuned the PL from Si QDs by varying the surface moieties without changing the size of Si QDs, as shown in Fig. 4. They proposed that the blue PL resulted from charge transfer states involving silicon oxynitride species, while the green and yellow PL originated from oxide-related surface defects. Their study highlighted the importance of surface states for the optical properties of Si QDs.

class="figure_img" id="Figure4"/>
Download
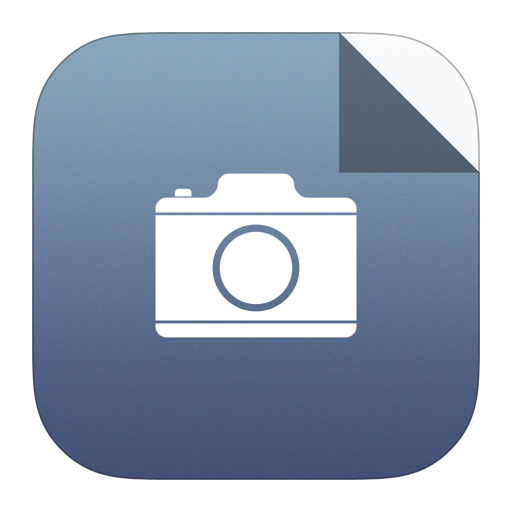
Larger image
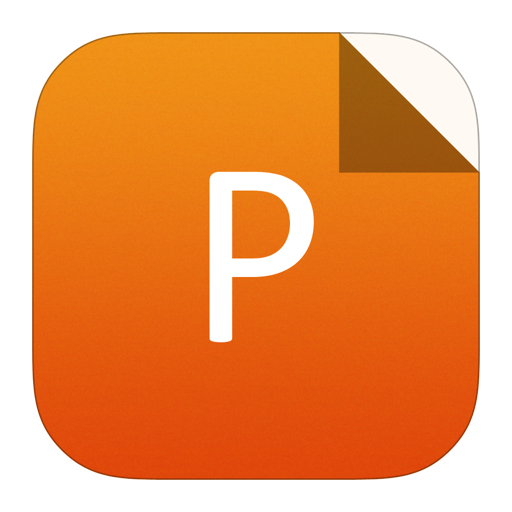
PowerPoint slide
Figure4.
(Color online) Photograph of 3–4 nm Si QDs functionalized with various surface groups dispersed in toluene under UV illumination. From left to right: blue, dodecylamine; blue-green, acetal; green, diphenylamine; yellow, TOPO; orange, dodecyl (air); red, dodecyl (inert).
Locritani et al.[72] attached pyrene chromophores at the surface of Si QDs to enhance light absorption. They obtained that the PL QY of the pyrene-treated Si QDs was 40% at the wavelength of ~970 nm. Li et al.[73] achieved the PL QY up to 90% with a narrow luminescence bandwidth (~40 nm) at the wavelength of ~555 nm for 1, 2, 3, 4-tetrahydrocarbazol-4-one modified Si QDs. They argued that the high PL QY of Si QDs was induced by nitrogen-related surface states. The PL energy of Si QDs depended on the structure of nitrogen-containing ligands at the QD surface. Fig. 5 schematically compares the PL induced by the surface states and that induced by the conventional quantum confinement.

class="figure_img" id="Figure5"/>
Download
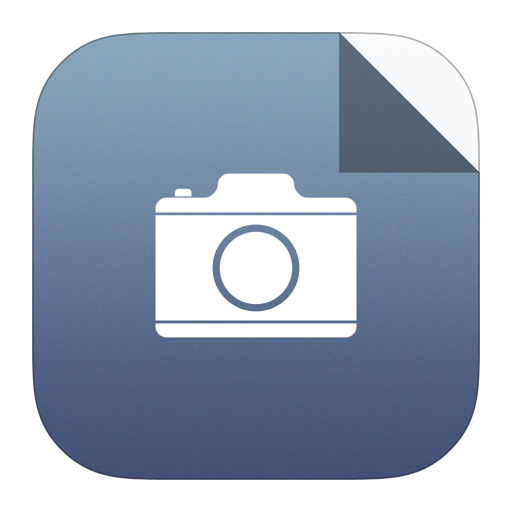
Larger image
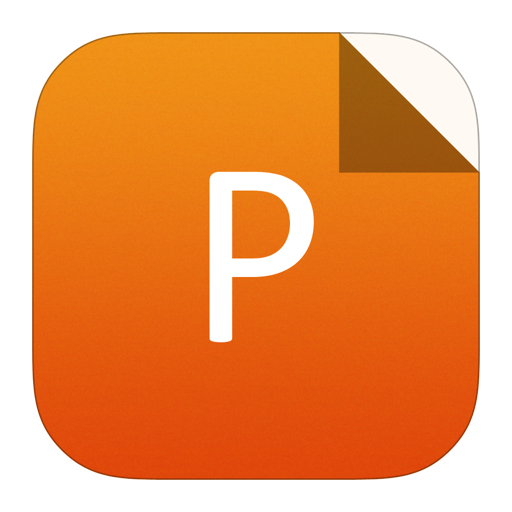
PowerPoint slide
Figure5.
(Color online) (a) Scheme of the PL from Si QDs with nitrogen-containing ligands at the surface. (b) Scheme of the PL from conventional Si quantum dots.
3.
LEDs based on colloidal Si QDs
3.1
QD surface ligands
Liu et al.[74] recently prepared colloidal Si QDs that were hydrosilylated with octyl and phenylproyl (PhPr) ligands (Fig. 6(a)). It was found that the PL QY and PL lifetime of PhPr-Si QDs were higher and longer than those of octyl-Si QDs, respectively (Fig. 6(b)). The surface of PhPr-Si QDs was more easily oxidized and less defective than that of octyl-Si QDs. LEDs based on octyl-Si QDs and PhPr-Si QDs with the device structure of indium tin oxide (ITO)/PEDOT:PSS/PVK/Si QDs/ZnO/Ag were fabricated. Owing to the enhancement of electron transport in phenylpropyl ligands, the optical power density of PhPr-Si QD LEDs was larger than that of octyl-Si QD LEDs (Fig. 6(c)). However, the benzene rings in phenylproyl ligands suppressed the hole transport and exacerbated the unbalance between the electron injection and hole injection in PhPr-Si QDs, leading to a lower EQE for PhPr-Si QD LEDs than that for octyl-Si QD LEDs, as shown in Fig. 6(d). In Liu et al.’s work[74], the highest power density of ~0.64 mW/cm2 from PhPr-Si QD LEDs and the highest EQE of ~6.2% from octyl-Si QD LEDs were achieved. These devices could compete with the best LEDs based on colloidal Si QDs in terms of the overall device performance.

class="figure_img" id="Figure6"/>
Download
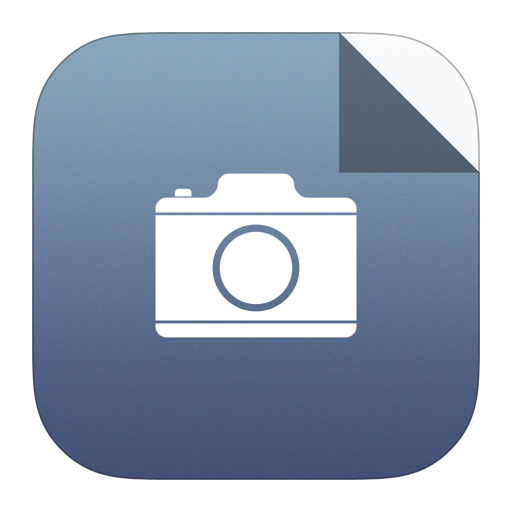
Larger image
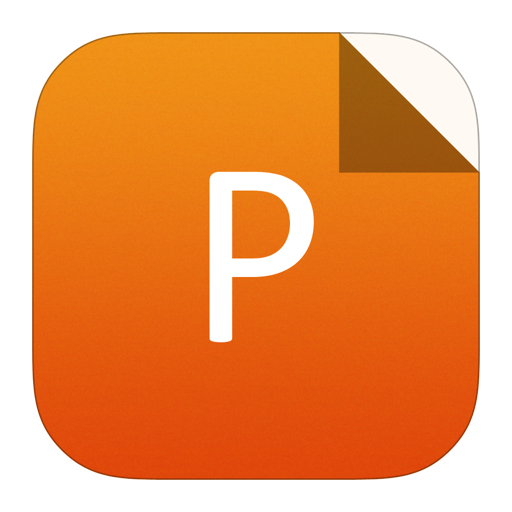
PowerPoint slide
Figure6.
(Color online) (a) Schematic of the Si QDs hydrosilylation with 1-octene and allylbenzene. (b) The PL decay curves of octyl-Si QDs and PhPr-Si QDs. (c) Optical power density (P) versus voltage (V) for an octyl-Si QD LED and a PhPr-Si QD LED. (d) EQE versus voltage (V) for an octyl-Si QD LED and a PhPr-Si QD LED.
3.2
QD size
Maier-Flaig et al.[75] demonstrated that the light emission from Si-QD LEDs could be tuned from the deep red to yellow-orange by using size-separated Si QDs. The QD sizes were approximately 1.8, 1.6, and 1.3 nm, corresponding to the light emissions at 680, 650, and 625 nm, respectively (Fig. 7). It was shown that the size of Si QDs had a significant impact on the valence band position of Si QDs. A high external quantum efficiency (EQE) of 1.1% as well as a low turn-on voltage of ~2 V were obtained for Si-QD LEDs with the size of ~1.8 nm (Fig. 8(a)). It was shown that the EQE of Si-QD LEDs fabricated with the smaller Si QDs (1.3 and 1.6 nm) was lower than that with 1.8 nm Si QDs. This was due to the reduced PL QY of the smaller Si QDs. In addition, the positions of the conduction band minimum (CBM) and valence band maximum (VBM) changed with the decrease of the QD size, leading to a mismatched configuration of energy levels of the device for small Si QDs. As a result, the carrier injection from the electrodes and carrier transport layers to the emission layer for devices with smaller Si QDs was not as efficient as that fabricated with large Si QDs.

class="figure_img" id="Figure7"/>
Download
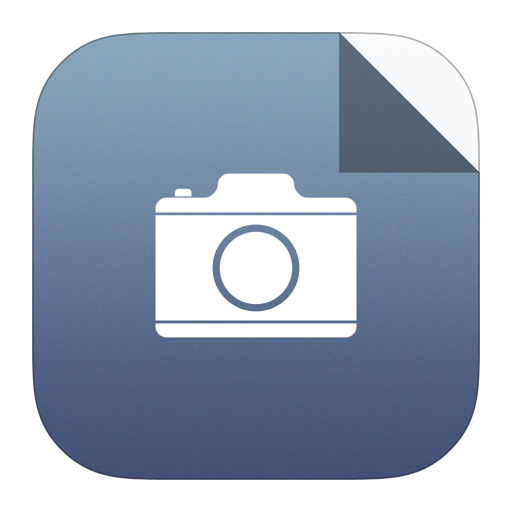
Larger image
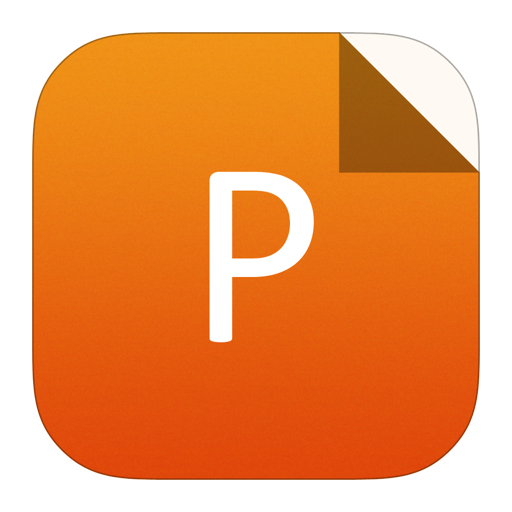
PowerPoint slide
Figure7.
(Color online) Size-separated Si QDs and their corresponding PL spectra. (a) Si QDs dispersed in toluene showing intense luminescence from the deep red to the yellow spectral region. (b) PL spectra of the three samples used for Si-QD LEDs fabrication. Excitation: (a) 365 nm LED and (b) 355 nm Nd:YAG laser. (c) High-resolution cross-section TEM image of a real device (scale bar: 50 nm).
When size separation was carried out for Si QDs, the device lifetime might be increased up to at least 40 h[75]. In contrast, LEDs based on non-size-selected Si QDs exhibited significantly shorter lifetimes (15 h), as shown in Fig. 8(b). The short lifetime was attributed to larger Si QDs in the Si-QD ensemble because they induced short circuits inside the devices, leading to the rapid device degradation. Maier-Flaig et al.[76] observed the drastic morphological changes and degradation of LEDs based on non-size-selected Si QDs at high driving voltages. Instead, LEDs built by using size-separated Si QDs showed no morphological and compositional changes. Their work indicated that a wider distribution of the Si-QD size might additionally facilitate interlayer element diffusion, which is detrimental to the device stability. Therefore, the size distribution of Si QDs should be taken into serious account in the optimization of the performance of Si-QD LEDs.

class="figure_img" id="Figure8"/>
Download
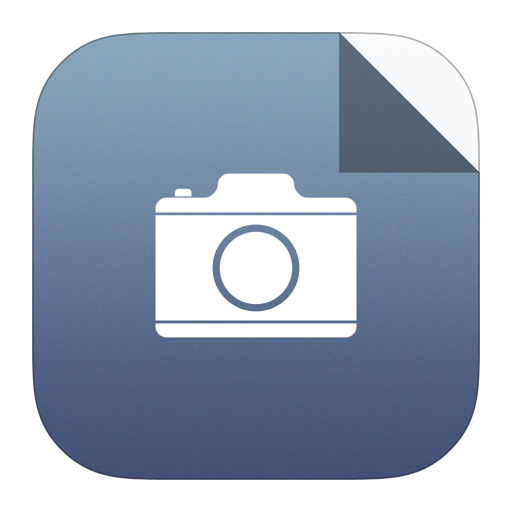
Larger image
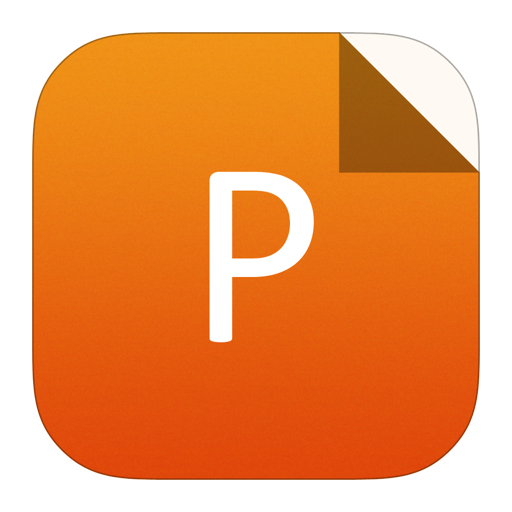
PowerPoint slide
Figure8.
(Color online) (a) External quantum efficiency (EQE) of devices containing different-sized Si QDs as emitters. (b) EL intensity of size-separated and non-size-separated Si QDs over time at constant current of 1.6 mA/cm2.
3.3
Device structure
3.3.1
QD-layer thickness
Cheng et al.[77] used Si QDs that were synthesized by a nonthermal plasma process and hydrosilylated with 1-dodecene to fabricate Si-QD LEDs. It was found that the EL and EQE of the devices exhibited a strong dependence on the QD-layer thickness, as shown in Figs. 9(a) and 9(b). As the concentration of Si QDs increased, the Si-QD layer became thicker. But the device current decreased. This suggested that the device resistance increased with the increase of the Si-QD layer thickness. In addition, with the increase of the Si-QD layer thickness, light emission at the wavelength of ~865 nm from the Si-QD layer became more intense. However, light emission at the wavelength of ~630 nm from the hole transport layer (HTL) of MEH-PPV (poly[2-methoxy-5-(2’-ethylhexyloxy)-p-phenylene vinylene]) became weaker. It was clear that a thin Si-QD layer could not solely act as the recombination zone. Carriers injected to the Si-QD layer might transfer to the neighboring HTL layer. The Si-QD layer should be thick enough to minimize the transfer of injected carriers to the neighboring HTL.Fig. 9(c) shows that the EQE up to 0.6% could be obtained for the Si-QD layer spun-cast from the Si-QD solution with the rather high concentration of 20 mg/ml. Apparently, the thickness of the Si-QD layer produced with the 20 mg/ml Si-QD solution effectively mitigated the transfer of injected carriers from the Si-QD layer to the HTL.

class="figure_img" id="Figure9"/>
Download
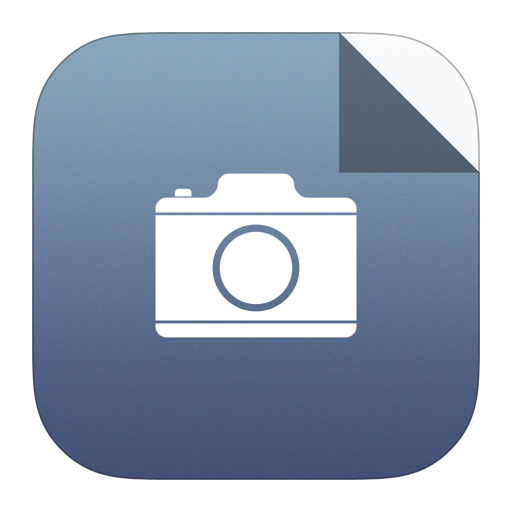
Larger image
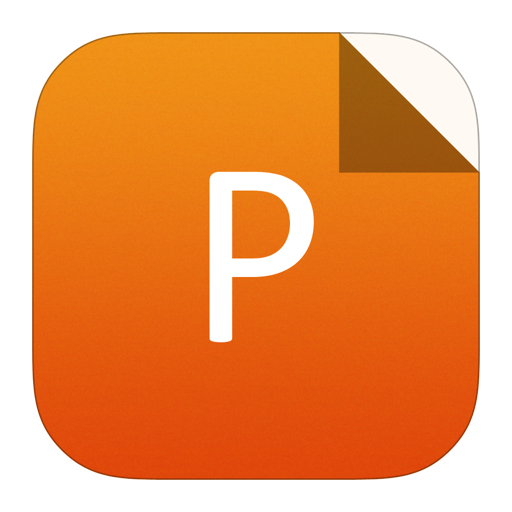
PowerPoint slide
Figure9.
(a) Current–voltage characteristics for hybrid Si-QD LEDs having emissive layers spun-cast from solutions with varying Si QDs concentration. (b) EL spectra for the devices (a) at an applied current density of 10 mA/cm2. The arrow denotes the direction of increasing Si-QD concentration. (c) EQE for a hybrid Si-QD LEDs with an emissive layer spun-cast from a Si-QD solution with the concentration of 20 mg/mL.
3.3.2
Carrier transport layers
Puzzo et al.[78] utilized two kinds of HTLs to optimize the performance of Si-QD LEDs. Poly(vinylcarbazole) (PVK) or poly(3, 4-ethylenedioxythiophene):poly-(styrenesulfonate) (PEDOT:PSS) was spun-cast onto ITO as the HTL. A 1, 3, 5-Tris(1-phenyl-1H-benzimidazol-2-yl)benzene (TPBi) layer was used as the electron transport layer (ETL). The luminance of the Si-QD LED containing PVK as the HTL was significantly higher than that containing PEDOT as the HTL, as shown in Fig. 10. This phenomenon was due to the following reasons. (1) For PEDOT-containing LEDs, an energy barrier for holes existed between PEDOT and Si QDs, which could hinder the efficient hole-injection. However, the hole injection from PVK to Si QDs was straightforward due to the lack of an energy barrier. (2) In contrast to the PEDOT, the PVK provided an energy barrier to confine electrons in the Si-QD layer, enabling a narrow light emission only from Si QDs. (3) Compared with the PEDOT, the PVK did not corrode the ITO electrode.

class="figure_img" id="Figure10"/>
Download
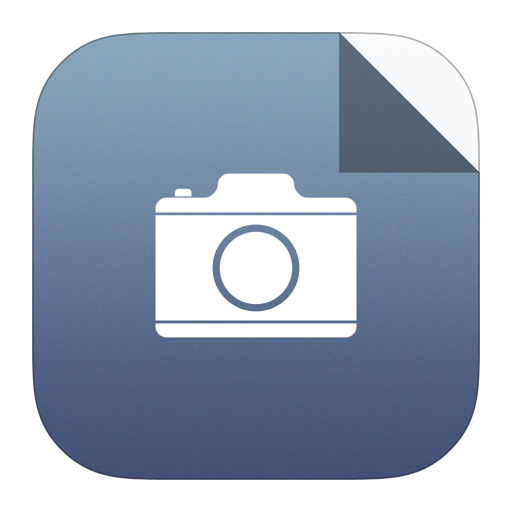
Larger image
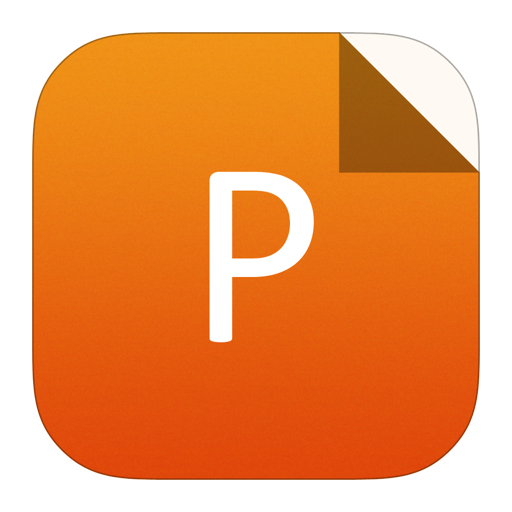
PowerPoint slide
Figure10.
Luminance and current density versus voltage plots of devices with PVK and PEDOT:PSS as HTL, respectively. The insets were the proposed energy level diagrams of the devices at zero field.
Cheng et al.[79] fabricated Si-QD LEDs with 5 and 3 nm Si QDs. The device structure was ITO/PEDOT:PSS/poly(N, N’-bis(4-butylphenyl)-N, N’-bis(phenyl)benzidine (poly-TPD)/Si QDs/ETL/Al. In this device structure a 30 nm thick PEDOT/PSS layer and a 25 nm thick poly-TPD layer acted as the HTL. The authors spun-cast tris-(8-hydroxyquinolinato) aluminum (Alq3), 4,4’,4"-tris-(N-carbazolyl)-triphenlyamine (TCTA) or N, N’-dicarbazolyl-4-4’-biphenyl (CBP) onto the emission layer as the ETL. Fig. 11(a) shows the energy level diagram of the devices. Figs. 11(b) and 11(c) compared the performance of Si-QD LEDs with the emission layer of 5 nm Si QDs coupled with the ETLs of Alq3, TCTA and CBP. It was found that the best EQE of ~8.6% could be obtained from the Alq3-containing LEDs. The values of the optical power density of the devices were similar when Alq3 and CBP were used as the ETLs. The turn-on voltage for TCTA-based devices (~2.0 V) was higher than those for Alq3- and CBP-based devices (~1.5 V). The highest efficiency and lowest-voltage operation for the Alq3-based device were induced by the best energy level alignment. The lower energy barrier between Alq3 and Si QDs benefited the electron injection from the electrode to Si QDs.

class="figure_img" id="Figure11"/>
Download
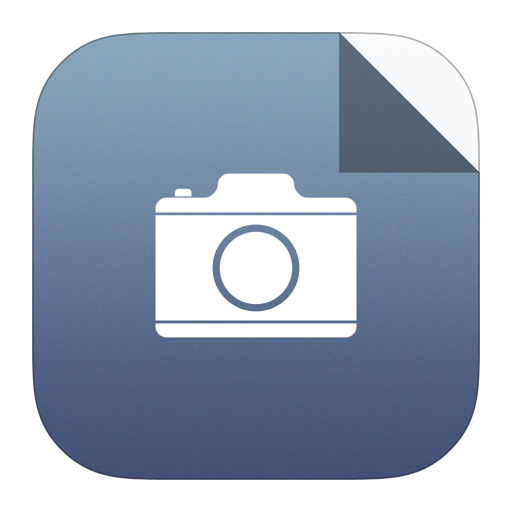
Larger image
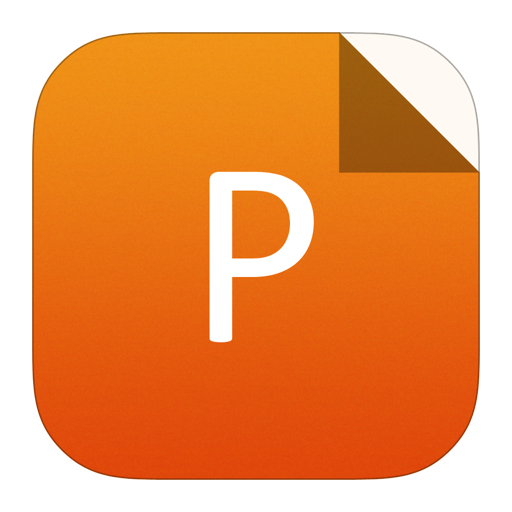
PowerPoint slide
Figure11.
Impact of the electron-transporting materials. (a) Proposed energy level diagram under zero applied bias for devices containing Si QDs with a diameter of 5 nm, and an ETL consisting of either Alq3, TCTA, or CBP. (b) EQE versus current density for devices containing either Alq3 (solid line), TCTA (broken line), or CBP (dotted line). (c) Current density voltage and optical power density voltage characteristics for the devices in (b).
3.3.3
Interlayers
The incorporation of an interlayer is an added means to improve the performance of Si-QD LEDs. The interlayer usually acts as a carrier-blocking layer, electrode-modifying layer or isolation layer. Gu et al.[80] demonstrated that Si-QD LEDs could work much more efficiently after using the interlayers between ITO and PEDOT:PSS to enhance the hole transport of the devices. They used dipyrazino (2, 3-f:2’, 3′-h) quinoxaline-2, 3, 6, 7, 10, 11-hexacarbonitrile (HAT-CN) or MoO3 as the interlayer. As presented in Fig. 12(a), the device structure was ITO/interlayer/PEDOT:PSS/poly-TPD/Si QDs/ ZnO/Ag. It was found that the work function of ITO increased after the insertion of the interlayer of HAT-CN or MoO3 (Fig. 12(b)).

class="figure_img" id="Figure12"/>
Download
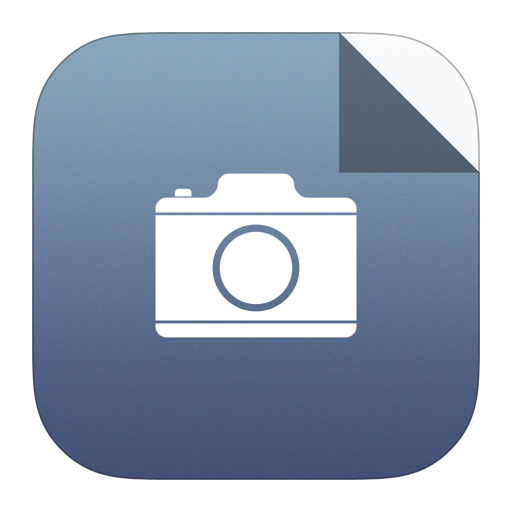
Larger image
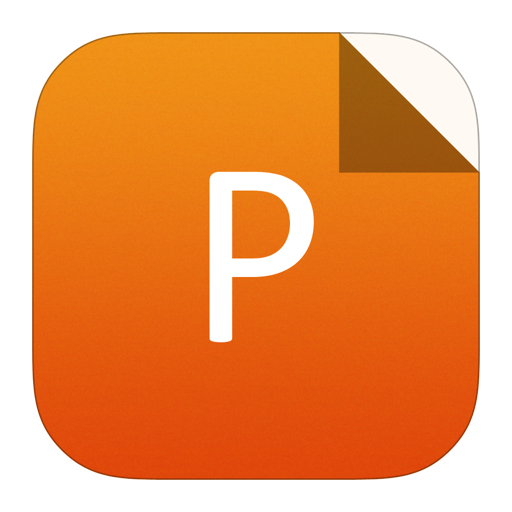
PowerPoint slide
Figure12.
(Color online) (a) Schematic of the structure of a Si-QD LED. (b) Work function of ITO (WA) modified by HAT-CN/MoO3 with varying film thickness.
When HAT-CN (MoO3) was deposited onto the ITO substrate, there might be a charge redistribution around the interface because of the work function (WA) difference. Electrons were transferred from ITO to HAT-CN (MoO3) and a dipole layer was formed, leading to an upward shift of the vacuum level (VL). Thus, the WA of ITO was increased. However, the shifted VL tended to be constant with the further deposition of HAT-CN (MoO3), giving rise to a stabilized WA. Once the WA hardly increased with the further increase of the interlayer thickness, the interlayer would induce an increase of the series resistance of the device. The trade-off between the increase of the WA of ITO and that of the interlayer-induced additional series resistance gave rise to the optimum interlayer thickness in Si-QD LEDs. It was shown that the optimum thickness for HAT-CN and MoO3 were ~5 and ~3 nm, respectively. The interlayers of ~5 nm thick HAT-CN and ~3 nm thick MoO3 had led to the maximum enhancement of the EQE of their Si-QD LEDs by ~22% and 170%, respectively. The maximum EQE of ~1.1% and ~2.4% were obtained when ~5 nm HAT-CN and ~3 nm thick MoO3 were used, respectively (Fig. 13).

class="figure_img" id="Figure13"/>
Download
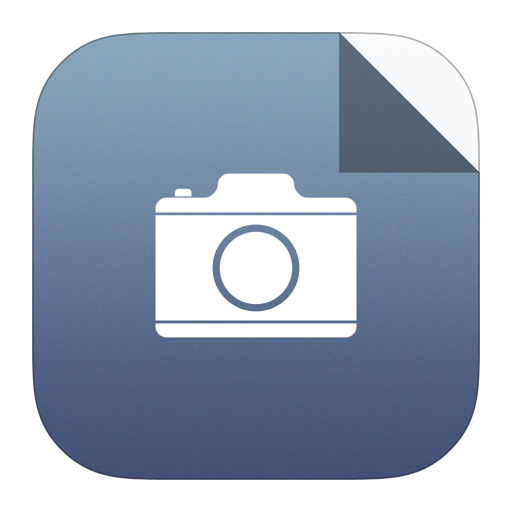
Larger image
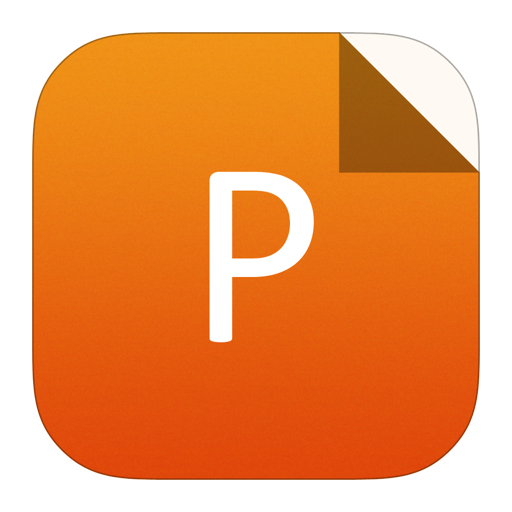
PowerPoint slide
Figure13.
(Color online) EQE versus current density characteristics for Si-QD LEDs without and with (a) HAT-CN and (b) MoO3 interlayers.
MoO3 could more effectively mitigate the charge unbalance than HAT-CN. Therefore, the increase of the device stability induced by MoO3 was more significant than that induced by HAT-CN, as shown in Fig. 14.

class="figure_img" id="Figure14"/>
Download
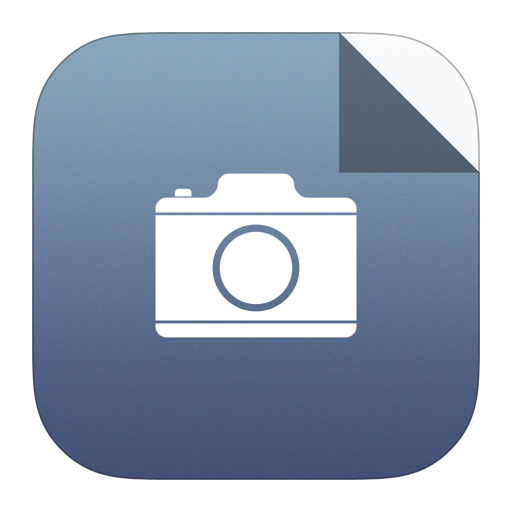
Larger image
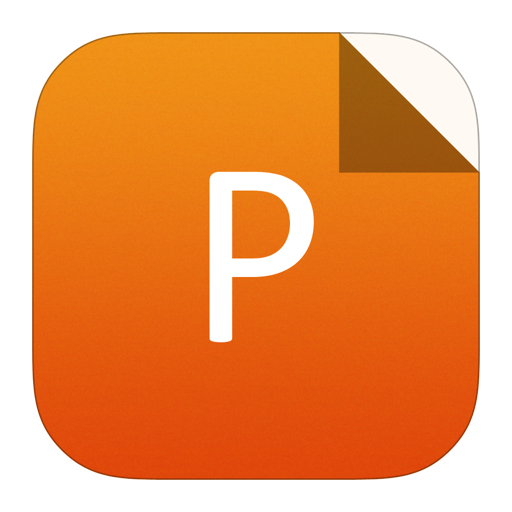
PowerPoint slide
Figure14.
(Color online) Stability data for Si-QD LEDs without any interlayer, with ~5 nm thick HAT-CN, and with ~3 nm thick MoO3 at the operating voltage of 8 V.
Zhao et al.[81] fabricated all-inorganic Si-QD LEDs by using Al2O3 as the interlayer. The device structure of ITO/NiO/interlayer (Al2O3)/Si QDs/ZnO/Ag was presented in Fig. 15(a). In this work, the NiO and ZnO were the HTL and ETL, respectively. It was found that the LED performance might be significantly improved by the atomic layer deposition (ALD) of an Al2O3 interlayer between Si QDs and NiO. It was shown that the passivation of the traps at the NiO surface by Al2O3 played a dominant role when the Al2O3 thickness increased from 0 to 5.7 nm. It was also found that the hole injection from the NiO layer into the QD layer was usually not very efficient, giving rise to a hole accumulation at the NiO/QD interface. The accumulated holes might effectively quench the excitons in Si QDs. It was clear that the insertion of Al2O3 between NiO and Si QDs could diminish the exciton quenching induced by the hole accumulation. Therefore, the decrease of the exciton quenching that was caused by both the traps at the NiO surface and accumulated holes made the optical power density and EQE of Si-QD LEDs increase when the Al2O3 interlayer with a thickness up to 5.7 nm was used. In addition, Al2O3 might limit the leakage of electrons toward the NiO layer and confine electrons in the Si-QD layer by introducing a large energy barrier for electrons (Fig. 15(b)). This should effectively suppress the carrier leakage induced by protuberance at the NiO surface. Clearly, the Al2O3-induced decrease of the carrier leakage also contributed to the improvement of the device performance as the Al2O3 interlayer thickness is up to 5.7 nm (Figs. 15(c) and 15(d)).

class="figure_img" id="Figure15"/>
Download
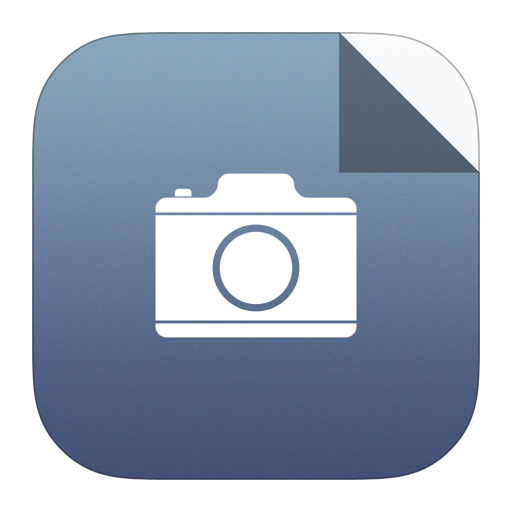
Larger image
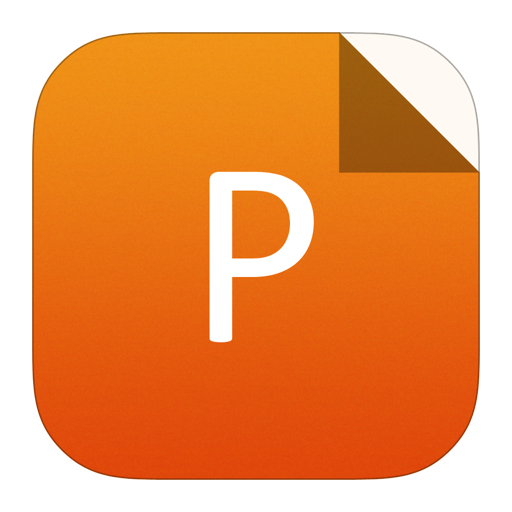
PowerPoint slide
Figure15.
(Color online) (a) Schematic of the structure of an all-inorganic Si-QD LED. (b) Flat-band energy-level diagram. (c) Power density versus voltage for all-inorganic Si-QD LEDs without and with Al2O3. (d) EQE versus current density for all inorganic Si-QD LEDs without and with Al2O3.
However, when the Al2O3 interlayer thickness increased from 5.7 to 9.0 nm, both the PL intensity and PL lifetime decreased. It is generally believed that positive charges existed in ALD grown Al2O3. For a rather thick Al2O3 layer the number of positive charges in Al2O3 might be too large to quench excitons in Si QDs, leading to the decrease of both the PL intensity and PL lifetime, as shown in Fig. 16. Moreover, it was expected that Al2O3 might weaken the hole transport because of the insulating nature of Al2O3.

class="figure_img" id="Figure16"/>
Download
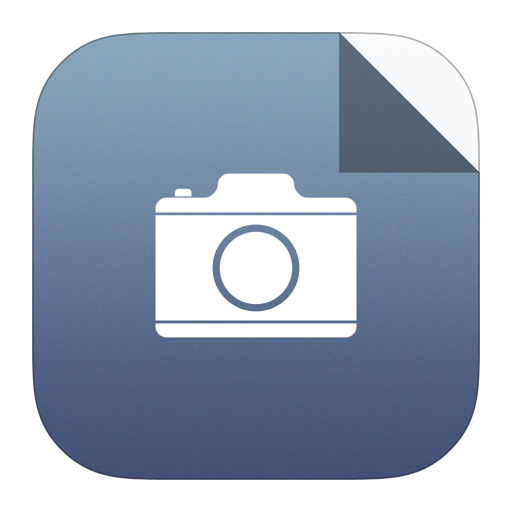
Larger image
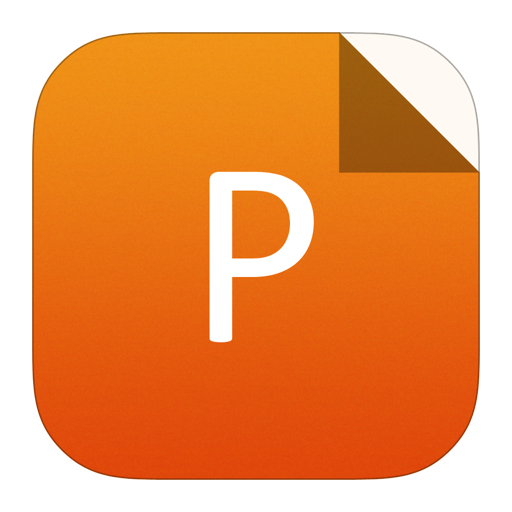
PowerPoint slide
Figure16.
(Color online) (a) PL spectra and (b) PL decay curves of Si QDs on NiO and Al2O3/NiO. The thickness of Al2O3 changed from 1.6 to 9.0 nm.
By using the optimum 5.7 nm Al2O3 interlayer the authors had also fabricated all-inorganic Si-QD LEDs on a flexible substrate of PET. The EQE and optical power density of the Si-QD LED on PET with a 5.7 nm Al2O3 interlayer may reach 0.01% and 5.5 μW/cm2, respectively (Figs. 17(a) and 17(b)). In addition, it was evident that the all-inorganic Si-QD LEDs with a 5.7 nm Al2O3 interlayer exhibited good work and storage stabilities (Fig. 17(c) and 17(d)).

class="figure_img" id="Figure17"/>
Download
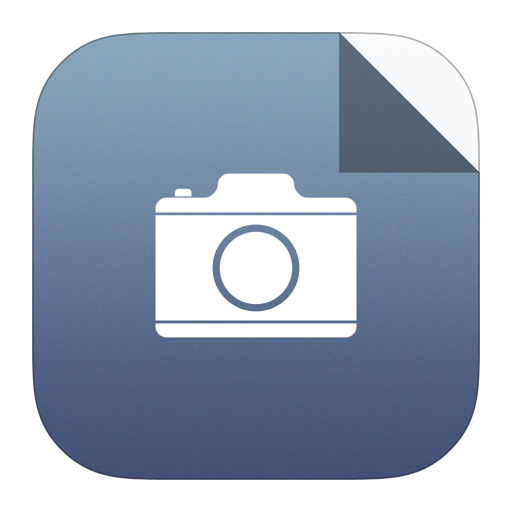
Larger image
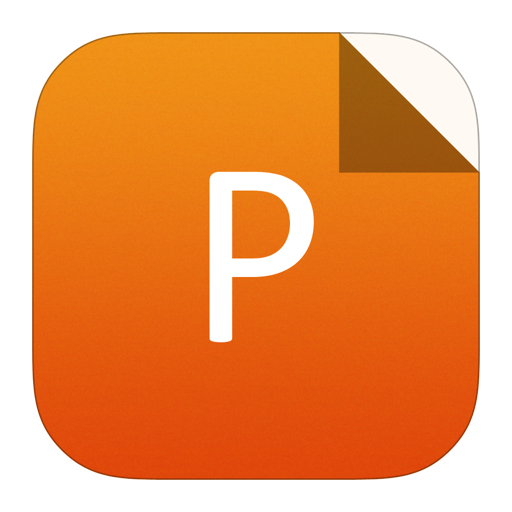
PowerPoint slide
Figure17.
(Color online) (a) Current density and power density versus voltage for flexible Si-QD LEDs without and with 5.7 nm thick Al2O3. (b) EQE versus current density for flexible Si-QD LEDs without and with 5.7 nm thick Al2O3. (c) Operation lifetime and (d) storage lifetime for Si-QD LEDs with 5.7 nm Al2O3. The driving voltage was 8 V.
Yao et al.[82] fabricated inverted Si-QD LEDs with the device structure of ITO/ZnO/Si QDs/1, 1-Bis[(di-4-tolylamino)phenyl]cyclohexane (TAPC)/MoO3/Al, as shown in Fig. 18(a). To improve the emission efficiency of Si-QD LEDs, poly(ethylene imine) (PEI) was used to modify the ITO cathode. It was known that the PEI which contained simple amine groups could substantially reduce the WA of various conductors including ITO. As shown in the energy level diagram, the WA of ITO was reduced from 4.59 to 3.13 eV, changing the electron injection barrier between ITO and ZnO to an electron well (Fig. 18(b)).

class="figure_img" id="Figure18"/>
Download
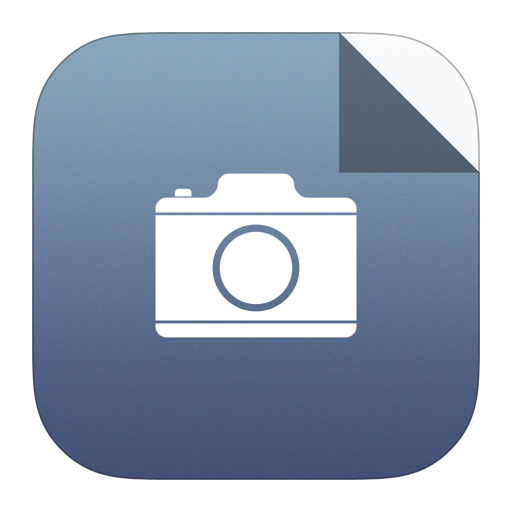
Larger image
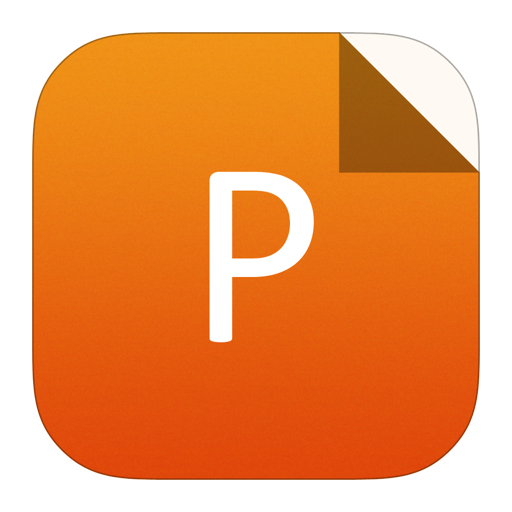
PowerPoint slide
Figure18.
(Color online) (a) A schematic structure diagram for the modified Si-QD LEDs with the structure of glass/ITO/PEI/ZnO NPs/SiQDs/TAPC/MoO3/Al. (b) The UPS spectra of pristine ITO and PEI modified ITO.
Therefore, after the modification of the ITO cathode with PEI, the maximum EQEs of the Si-QD LEDs were improved from 1.1% to 2.7%. In addition, the maximum optical power density of the Si-QD LEDs increased from 0.04 mW/cm2 to 0.11 mW/cm2 at the forward bias of 18 V (Fig. 19).

class="figure_img" id="Figure19"/>
Download
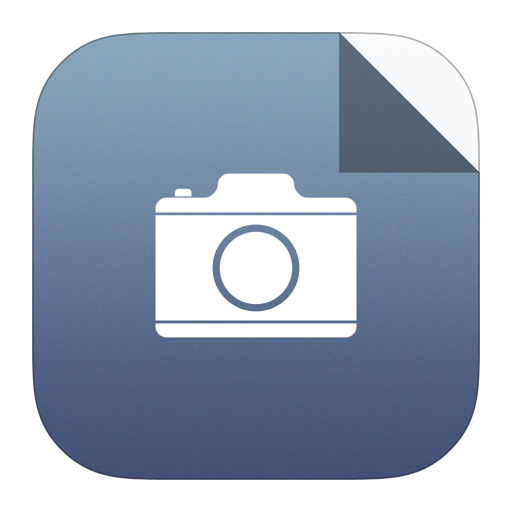
Larger image
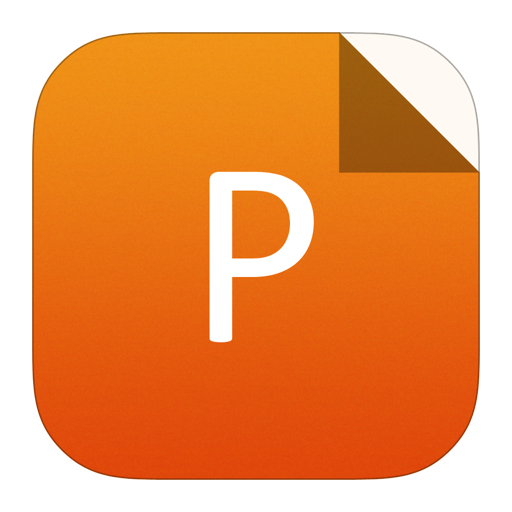
PowerPoint slide
Figure19.
(Color online) (a) External quantum efficiency versus current density, (b) optical power density versus voltage characteristics for the modified Si QD-LEDs (red circles) and the reference Si QD-LEDs (blue rectangles).
4.
Outlook
Impressive progress has been achieved in the development of LEDs based on colloidal Si QDs. It has been realized that the tuning of the size, surface of Si QDs and the design of device structures are critical to the further optimization of the performance of colloidal Si-QD LEDs. First of all, novel surface modification schemes for Si QDs need to be studied to realize the excellent dispersibility of Si QDs in common solvents, the high PL QY of Si QDs and the effective carrier transport between Si QDs at the same time. Although the size separation has been carried out for Si QDs, the resulting size distribution remains larger than that usually obtained in the study on other types of QDs such as II–VI QDs. Both the synthesis and processing of Si QDs should be optimized to narrow the size distribution of Si QDs.
The unbalance between the hole injection and electron injection routinely occurs to colloidal Si-QD LEDs because the hole mobility of HTLs is often smaller than the electron mobility of ETLs. Novel HTLs with high hole mobility are urgently needed. It seems that interlayers have become indispensable for QD LEDs. Interlayers can prevent exciton transfer and quenching. They can also modify electrodes and improve carrier injection. Compared with the traditional interlayers, novel interlayer materials such as PMMA, Al2O3 and PEIE[83] are bound to play important roles in the enhancement of the device performance. Interlayers may be prepared by using a series of methods such as spin-coating, ALD, thermal evaporation and printing. It may be anticipated that novel interlayer materials prepared with appropriate methods will significantly contribute to the improvement of the performance of colloidal Si-QD LEDs.