

First author contact:
Received:2019-10-06Revised:2019-11-13Accepted:2019-11-14Online:2020-02-28

Abstract
Keywords:
PDF (483KB)MetadataMetricsRelated articlesExportEndNote|Ris|BibtexFavorite
Cite this article
Changsheng Chen(陈昌盛), Jing Li(李敬), Xianren Zhang(张现仁). The existence and stability of bulk nanobubbles: a long-standing dispute on the experimentally observed mesoscopic inhomogeneities in aqueous solutions. Communications in Theoretical Physics, 2020, 72(3): 037601- doi:10.1088/1572-9494/ab6183
1. Introduction
Bulk nanobubbles that disperse in a bulk solution are normally reported to have a size less than 1 μm and can be generated via a number of techniques: electrolysis [1, 2], agitation dissolution [3], mixed pressurization [4], the cyclone shear method and ultrasonic technique [5–7]. The tiny bubbles exhibit numerous structural properties that are entirely different from ordinary macrobubbles, including a large specific surface area, low buoyancy and slow rising velocity, high surface zeta potential, high gas solubility and a large amount of hydroxyl radicals generated by bubble collapse [7–12]. These unique properties lead to a massive range of current and expected applications of nanobubbles, including, but not limited to, ecological restoration [9, 13, 14], sewage treatment [9, 13–15], biomedicine [16–27], aquaculture [13, 28], plant cultivation [13, 28, 29], the cleaning industry [2, 9, 15, 30–32], the food and beverage industry [33, 34], interface slip [35, 36], mineral flotation [37, 38] and enhanced chemical reactions [39]. In particular, for ecological restoration and wastewater treatment, nanobubbles have the potential to become a revolutionary technology in the field of environmental protection. One of the most surprising properties of bulk nanobubbles is their unexpected long-term stability, which is essential for their applications but inconsistent with classical theories. Consequently, stability mechanisms and applications of bulk nanobubbles have recently become a hot topic.The discovery of bulk nanobubbles originated from the early studies on ultrasound and cavitation, even though direct observations of bulk nanobubbles were reported much later. In 1962, Sette and Wanderlingh [40] found that high-energy neutrons can reduce the acoustic energy required to initiate water cavitation, and the reduction was ascribed to the cavitation nuclei formed by the deposition energy of neutrons. The experimental observations [41], of which exerting high pressure to solution can significantly increase the oversaturation threshold for cavitation, was also interpreted as a consequence of the pre-existing small cavities: the high pressure increases the solubility of the gas in water and thus forces the gas confined in small cavities to dissolve. It was not until 1981 that Johnson and Cooke [42] reported indirect evidence for the existence of bulk micro-nanobubbles less than 1000 nm in seawater. They proved that the encapsulated cavities were filled with gas, which would expand under negative pressure and shrink under positive pressure. However, our understanding of bulk nanobubbles has been rather limited for a long time. After 2000, experimental reports on bulk nanobubbles emerged due to the extensive use of advanced experimental techniques, such as dynamic light scattering (DLS), phase microscopy, infrared spectroscopy and nanoparticle tracking analyzers [4, 5, 43, 44].
2. Nanobubble stability contradicts classical theories on bubble stability
In recent years, stability mechanisms of bulk nanobubbles have become a hot topic. Although numerous reports appeared, the existence of bulk nanobubbles is always in dispute due to their unexpected stability. According to the classical Young–Laplace equation, the Laplace pressure Δp is given by Δp=2γ/R for a spherical bubble with a radius R and a liquid/gas interfacial tension γ. This means that a smaller bubble would experience a higher additional (Laplace) pressure. For example, the Young–Laplace equation predicts a Δp of ∼3 MPa for nanobubbles of R=100 nm, and 30 MPa for nanobubbles of 10 nm. This, in turn, requires a very high gas concentration in solution to reach equilibrium with the extremely high gas pressure in the interior of the nanobubble as a consequence of Henry’s law. According to Henry’s law, the concentration of dissolved gas is proportional to the partial pressure of gas inside nanobubbles. However, even if the gas distributions inside and outside of the nanobubbles attain equilibrium, the equilibrium is in fact unstable. For instance, when the bubble begins to shrink due to thermal fluctuation, the internal pressure increases because the Laplace pressure is inversely proportional to bubble size, causing the gas in the bubble to diffuse out and leading to further shrinkage of the bubble. This positive feedback mechanism causes the bubbles to shrink quickly, leading to the disappearance of the bulk nanobubbles. For the same reason, the initially slight expansion will cause the nanobubbles to move further away from the unstable equilibrium state, leading to unbounded bubble growth. Therefore, the nanobubbles cannot be stable in an open system from the view of thermodynamics.Epsten and Plesset studied the dynamic evolution of bubbles [45], and they pointed out that bubbles in solution will shrink or expand, depending on whether the dissolved gas in solution is oversaturated or not. The time required for complete dissolution of a bubble can be determined with the Epsten and Plesset (E-P) equation [46], which predicts that the survival time for nano-sized bubbles should be less than 0.02 s.
In contrast to both thermodynamic and kinetic arguments that both indicate instability of bulk nanobubbles, numerous experiments reported the existence of bulk nanobubbles [3, 4, 47–50]. More importantly, the existence period of observed nanobubbles ranges from several minutes to weeks, significantly longer than the theoretical prediction. To interpret the unexpected observations, different conjectures have been proposed [5, 6, 8], although there has not yet been a unified conclusion [39, 51, 52].
3. Nanobubbles or nanodroplets?
We also need to point out that in some experimental designs, although unintentional, the inability to distinguish nanobubbles from nanodroplets often induces misunderstanding of nanobubbles. This may partly be the reason for the controversy on the existence and stability of nanobubbles. There must be some reported nanobubbles that are in fact nanodroplets, namely the dispersed nanostructures that are assembled by amphiphilic molecules or that came from other sources of contamination, such as nano-sized particles [39]. This speculation is confirmed by numerous studies, which show nanoclusters, such as aggregates of organic molecules, nanoparticles, organic contaminants or their mixture, also cause light scattering. For example, Häbich et al [51] found that nano-sized particles causing light flashing were observed when mixing organic solvent and water, but the particles did not disappear after degassing, indicating that the particles might be organic pollutants instead of nanobubbles. As consistent evidence, Sedlák and Rak [43, 52] found the existence of nano-sized particles of ∼100 nm in many organic, inorganic and charged as well as non-charged solutions, normally showing a lifetime from several minutes to weeks. These particles were found to change insignificantly with degassing, and the density difference between the particles and the solution was also inconsistent with that for gas bubbles, as demonstrated by centrifugal separation. Therefore, the authors speculated that the nanoparticles are aggregates of some solid particles, rather than nanobubbles. In aqueous tertiary butyl alcohol (TBA) solution nanoparticles of ∼100 nm were also observed [53], which were similarly considered as aggregates but not nanobubbles. These aggregates were hypothesized to be clusters of mixed clathrate-hydrates that are formed through trace amounts of propylene oxide, an impurity expected to be present in all commercial samples of TBA.On the other hand, an increasing number of reports exist that support the fact that some inhomogeneous meso-structures observed in solutions are indeed bulk nanobubbles. Qiu et al [15] confirmed that the nanoclusters they observed were indeed gas-containing nanobubbles, since the concentration of nanoclusters produced by ethanol and water exchange without degassing was significantly increased when compared to that after degassing. In their well-designed experiments, Eklund and Swenson produced bulk nanobubbles with both hydrodynamic cavitation and vigorous shaking [54], and they concluded that organic contaminants play an essential role in stabilizing the nanobubbles. Xiao et al [55] proved that nanobubbles can be generated by mixing solutions with different gas contents, and they found through comparative study that both adding solid nanoparticles and increasing the gas concentration facilitate the formation of stable nanobubbles. The above experimental results do demonstrate the existence of stable nanobubbles, and at least for particular cases the known or unknown impurities are needed to stabilize the formed nanobubbles, through lowering of the surface tension or by acting as bubble nucleation sites.
4. Stability mechanisms of bulk nanobubbles
Several mechanisms for stabilizing gas nanobubbles have been proposed, but for most of them there is experimental evidence to the contrary. Four main mechanisms for nanobubble stability will be discussed here, including the contaminant mechanism [43, 44, 56], skin mechanism [47, 49, 57–60], surface zeta potential mechanism [3, 4, 61] and high density mechanism [62–64].4.1. Contaminant mechanism
Organic contaminants or other sources of amphiphilic particles have been invoked to interpret the long-term lifetime of bulk nanobubbles, since it is the enhanced adsorption at the liquid–gas interface that effectively lowers the surface tension and thus affects stability. Using dynamic laser light scattering (DLS), Jin et al [44] found that nano-sized particles exist in tetrahydrofuran (THF)/water, urea/water, ethanol/water and α-cyclodextrin/water mixtures. They demonstrated, using isothermal compression measurement, that the measured density for those nanoparticles is much smaller than that of the liquid phase, proving that what they observed were bulk nanobubbles. Furthermore, these bubbles can be removed by filtration or regenerated by aeration, and thus the authors suggested that the nanobubbles can exist stably because amphiphilic surface active molecules (THF, ethanol, urea and α-cyclodextrin) probably exist on the surface of bubbles, which reduce the surface tension γ of bubbles and lower Δp (Δp=2γ/R) accordingly, thus preventing gas molecules from diffusing out of the nanobubbles.By adding small amounts of ethanol to pure water, Nirmalkar et al [56] found a sharply increased number of stable bulk nanobubbles which could stably survive for more than three months. In addition, the number of bulk nanobubbles reached a maximum at an intermediate solvent concentration, generally around 10%–50%. Beyond this maximum, the bubble number density sharply decreases. This can be partially interpreted by the dependence of surface tension on the ethanol concentration as a result of the preferable presence of ethanol molecules on the nanobubble interface. Qiu et al [15] also experimentally reported the appearance of bulk nanobubbles in a particular range of ethanol fraction, revealing the significant roles that amphiphilic molecules played on the formation and stability of bulk nanobubbles.
With resonance mass spectrometry, Alheshibri and Craige [65] observed the existence of nanobubbles in the presence of phospholipids. They argued that the phospholipid layer surrounding a nanobubble produces a barrier resistance that counteracts the Laplace pressure, whereas the low solubility of phospholipids can keep the bubble coating stable and prevent gas from diffusing.
Yasui et al [5, 66] suggested that nanobubbles can stably exist within the framework of dynamic equilibrium, under the condition when the coverage rate of hydrophobic material on the nanobubble surface ranges from 50% to 100%. This mechanism resembles the mechanism of stabilizing macrobubbles with polystyrene particles that fully cover the bubble surface [67]. This seems to be reasonable since sufficiently large organic scaffolds on bubble interfaces produce repulsive force, which counteracts the Laplace pressure and stabilizes the bubble from dissolution in air-saturated water. A similar effect was also found for microbubbles: the added surfactants and particles are able to stabilize microbubbles [68, 69].
Although it is accepted that interface enhanced adsorption of surface active agents can help to stabilize nanobubbles, the exact mechanism of how the surface active agents work is still not available. However, the mechanism that was proposed by Guo et al [70] to interpret how organic pollutants (also surfactants) stabilize surface nanobubbles may shed important insights into the stability of bulk nanobubbles. The proposed stability mechanism is illustrated in figure 1, for which the enrichment of surface active molecules on the bubble surface is ascribed to the stability of surface nanobubbles, which is called the self-restoring mechanism. The self-restoring mechanism reveals that, due to constant adsorption of trace impurities on the nanobubble interface, the nanobubble growing or shrinking causes the increase and decrease of interfacial tension, acting as a restoring force to bring the nanobubble to its equilibrium size [70]. The mechanism can be easily generalized to explain the stability of bulk nanobubbles.
Figure 1.
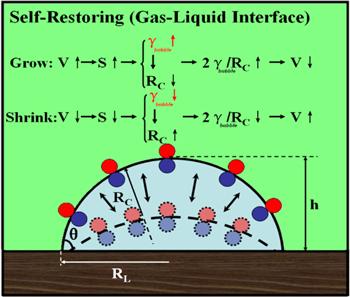
Figure 1.The stabilization mechanism of nanobubbles due to adsorption of trace impurities [70]. Copyright 2019 American Chemical Society.
4.2. Skin mechanism
The skin model for bubble stability was probably first proposed by Fox and Herzfeld [57] to interpret the particularly easy occurrence of decompression sickness (DCS), a disease caused by rapid decompression from high atmospheric pressures. Since nucleation theory predicts a much higher gas supersaturation required for nucleating a bubble than that which could be achieved in a conventional diving exposure, the pre-existing gas micronuclei model was proposed to interpret the difference between theory and experiment. However, such a model of micronuclei also suffers from a stability problem as nanobubbles do: the long-term existence of these gas micronuclei needs an extra stabilizing force. Fox and Herzfeld thus suggested that an organic impermeable skin that formed on the bubble surface could stabilize the bubble nuclei, but soon the idea seemed to be questionable as Strasberg demonstrated that a cyclic change in pressure significantly affected the stability of micronuclei [58].Ohgaki et al [20] proposed a new version of the skin model to interpret the nanobubble stability, based on their experimental measurements on nanobubbles using scanning electron microscopy (SEM) and Raman spectroscopy. They found nanobubbles of ∼50 nm in N2/H2O, CH4/H2O and Ar/H2O systems, which could exist stably for two weeks. The experimental results seemed to indicate the appearance of a strong hydrogen bond structure that formed on the surface of bulk nanobubbles under a gas supersaturated environment. The so-called ‘hard hydrogen bond’ structure, which was assumed to serve as a shielding film that stabilizes bulk nanobubbles, was proposed, which would reduce the diffusivity of gas from the nanobubble and maintain a kinetic balance against the high internal pressure of nanobubbles. Similarly, Zhang et al [59] also proposed a super-skin model, which believes that nanobubbles are surrounded by a layer of super-stable skin. The skin can keep nanobubbles stable dynamically, slows down their dynamic motion and promotes the stabilization of nanobubbles. A shielding effect was proposed by Weijs et al [60] based on their molecular dynamics simulations, which show that when the spacing of nanobubbles is sufficiently small, the shield effect stabilizes the nanobubbles by preventing gas from diffusing.
The skin shield mechanism can partly explain the stability of bulk nanobubbles under higher additional pressure. However, this kind of model has also been questioned because the number of nanobubbles after degassing is significantly reduced in ethanol–water exchange systems [15]. Further, the skin-like effects have not been found for microbubbles and macrobubbles, giving rise to the open question why and how the hard skin-like structures appear when the bubble size becomes microscopic.
4.3. Zeta potential mechanism
Regarding the stability of bulk nanobubbles, the electrostatic repulsion between neighboring nanobubbles will certainly play an important role. Zeta potential is a measure of the magnitude of the electrostatic repulsion and attraction between neighboring particles (see figure 2), and thus is one of the fundamental variables inferring nanobubble stability.Figure 2.
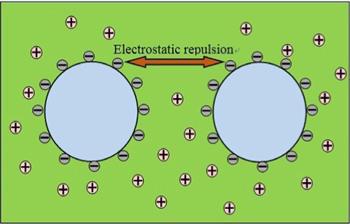
Figure 2.The zeta potential model diagram. The negative charges on bubble surfaces prevent adjacent nanobubbles from coalescing, which encourages them to stabilize.
Oh and Kim [3] found that mixing pure water and CO2 in a certain way can produce CO2 nanobubbles of diameter 60–120 nm with a lifetime more than 24 h, much longer than the theoretical prediction. Based on the measured ζ-potential for nanobubbles ∼−20 mV, the authors suggested that the surface of nanobubbles is enriched with negative charges, which induces a repulsive force between adjacent bubbles and keeps the nanobubbles stable. It was also reported [71] that the zeta potential for hydrogen nanobubbles generated in gasoline fuel was about −30 mV, even after 121 days of observation, and again their stability was ascribed to the inter-nanobubble repulsion that is parameterized by the zeta potential.
In addition, Ushikuboa et al [4] showed that NMR spin–lattice relaxation time increased with the introduction of micro- and nano-bubbles in a manganese ion solution, indicating the adsorption of manganese ions onto the newly introduced gas–liquid interfaces. Summarizing their experimental results the authors speculated that ζ-potential on the nanobubble’s surface induces a repulsive interaction between adjacent nanobubbles and prevents them from accumulating, leading to the extreme stability of nanobubbles. Replacing ethanol–water solutions (70%–100% V/V) with deionized water [61] is capable of generating bulk nanobubbles as a result of the increase in air supersaturation induced by the solvent exchange. In addition, the experiments investigated the effect of charged and polar solutes (salt and sugar) on the formation and surface chemistry of nanobubbles, confirming that cations adsorbed on the bubble’s surface participate in stabilizing nanobubbles.
Using the ultrasonic cavitation technique Nirmalkar et al [6, 72] produced nanobubbles of 100–120 nm, which could exist stably for several months without significant size changes. The nanobubble solutions in their experiments exhibit a considerable zeta potential of about −24 mV, which remains constant over time. Therefore, the authors speculated that the constant surface charges are probably the reason behind their stability. The conjecture was confirmed by the observations that both alkaline medium and the anionic surfactant sodium dodecyl sulfate (SDS) increased the electrostatic stability of nanobubbles. Further, the authors showed that the addition of salts leads to the screening of the electric double layer, and as expected, destabilizes the nanobubbles.
Nanobubbles that were internally generated by ultrasonication were reported in aqueous electrolyte and surfactant solutions [73]. The bubble sizes were found to be slightly increased when salts were added and to be sharply reduced when surfactant was added. The created nanobubbles showed a lifetime up to 1 h, and their stability was interpreted with the measured zeta potential. Ahmed et al. [74] also ascribed the zeta potential to the stability of nanobubbles. They suggested that both the ionic strength and organic components in solution can affect the stability of nanobubbles by changing the zeta potential of bubbles. Wu et al [75] produced hydrodynamic cavitation form ∼500 nm nanobubbles by high-intensity agitation. Again, the ζ-potential and surfactant on bubble surfaces have shown important effects on the stability of bubbles. Jin et al [76] also demonstrated the dual role that the ζ-potential and surfactant played in the stability of nanobubbles. In addition, Bunkin et al [48, 50, 77], Yurchenko et al [78], Meegoda et al [79], Boshenyatov et al [80], Uchida et al [81] and Liu et al [82] found ion-stabilized nanobubbles, and demonstrated the effect of charges on nanobubble stability in different electrolyte solutions.
However, the zeta potential alone is not enough to infer nanobubble stability. This mechanism can explain the problem of aggregation of adjacent nanobubbles since zeta potential is an indicator of the interaction between neighboring particles. But this mechanism is never capable of solving the problem of stability for a single bubble: how individual nanobubbles reduce the dissolution caused by the high Laplace pressure.
4.4. High internal gas density model
To interpret the abnormal nanobubble stability, Zhang et al [62] argued that the lifetime of nanobubbles can be increased by at least four orders of magnitude, even close to the time scale of experimental observation if the internal gas density of nanobubbles is sufficiently high. Huang et al [63] also suggested that hydrogen gas inside hydrogen nanobubbles is in a ‘dense gas’ phase. In agreement with the high gas density model, Zhang et al [64] found that the stability of nanobubbles is very sensitive to the concentration of nitrogen molecules in water. The nanobubbles can be stable for a long time when the concentration of nitrogen in the solution exceeds a critical concentration, and the density of nitrogen inside the nanobubbles is expected to be far greater than that under normal conditions.5. Summary
Although there are numerous experimental reports of bulk nanobubbles, their stability remains a controversial topic that is subject to the classical predictions of high Laplace pressure. There are three or four main stability mechanisms for bulk nanobubbles, as summarized in this review. (1) The contaminant mechanism: amphiphilic surfactants or other sources of organic contamination would be enriched on nanobubble surfaces, and this layer of surface active agents not only reduces the effective surface for gas to diffuse through and thus prevents gas loss, but also decreases the surface tension of the gas–liquid interface, inducing the reduction of the Laplace pressure and stabilizing the nanobubbles. (2) The skin mechanism, in which the organic adsorbents form an impermeable or sometimes partially permeable skin or, in its more recent version, in which the skin is formed by hard hydrogen bonds. This skin can shield the nanobubbles, preventing gas diffusing through the interface layer, and thereby stabilizing the nanobubbles. (3) The surface zeta potential mechanism: the cations are more likely to leave the gas–liquid interface of nanobubbles, leading to an interface with more negative charges. The charged surface tends to adsorb the counter ions in solution, forming an electric double layer that can be described by the ζ-potential. The large magnitude of the ζ-potential accounts for the repulsive force between the adjacent nanobubbles, which inhibits the accumulation of nanobubbles. (4) The high internal gas density model: high gas density inside nanobubbles would significantly lengthen the lifetime of the nanobubbles. The stability of bulk nanobubbles is still an open challenge. The use of advanced probe techniques would certainly deepen our understanding of nanobubbles. To deal with this open question, an impressive amount of experimental work on surface and bulk nanobubbles has been conducted by Hu and Zhang and their co-authors in the past [7, 15, 55, 83–85]. As a complementation of the experimental exploration, theoretical and computational studies on surface and bulk nanobubbles emerged recently [86–92].Although the above mechanisms shed insights into understanding the stability of bulk nanobubbles, several challenges and limitations still remain. First, there has not yet been a unified answer to nanobubble stability. Although several mechanisms have been proposed, for most of them there is experimental evidence to the contrary. Different nanobubble experiments often seem to indicate different stabilization mechanisms, and we have no idea under what conditions the particular mechanism governs the bubble stability. What is more complicated is that two or more stability mechanisms sometimes seem to work in a single experiment, namely, they do not contradict each other. In certain situations, we indeed have a better understanding of the nanobubble stability when combining different governing mechanisms. Another challenge is the unavoidable existence of trace contamination or impurity, which is expected to be present in most commercial samples. The undesired contamination is normally sensitive to nanobubble surfaces, and exerts unknown effects on nanobubble stability. Due to the complexity involved in nanobubbles, including unknown impurity, high Laplace pressure, the multi-components involved, uncontrollable gas dissolution and polydispersity of bubble size, more effort is required to fully understand the stability mechanisms of bulk nanobubbles.
Acknowledgments
This research was supported by the National Natural Science Foundation of China (No. 91434204).Reference By original order
By published year
By cited within times
By Impact factor
DOI:10.1016/S0022-0728(01)00517-4 [Cited within: 1]
DOI:10.1021/acs.langmuir.6b01004 [Cited within: 2]
DOI:10.1021/acs.langmuir.7b00510 [Cited within: 4]
DOI:10.1016/j.colsurfa.2010.03.005 [Cited within: 5]
DOI:10.1016/j.ultsonch.2018.05.038 [Cited within: 4]
DOI:10.1021/acs.langmuir.8b01163 [Cited within: 2]
DOI:10.1088/1674-1056/27/11/118104 [Cited within: 3]
DOI:10.1002/cphc.201100900 [Cited within: 1]
DOI:10.1016/j.chemosphere.2011.05.054 [Cited within: 3]
DOI:10.1103/PhysRevE.91.033008
DOI:10.1103/PhysRevLett.101.214505
DOI:10.1016/j.jaci.2010.12.343 [Cited within: 1]
DOI:10.1016/j.ces.2013.02.004 [Cited within: 4]
DOI:10.2175/106143014X14062131177953 [Cited within: 1]
DOI:10.1002/cphc.201700010 [Cited within: 6]
DOI:10.1016/j.ultras.2007.11.002 [Cited within: 1]
DOI:10.1016/j.ultrasmedbio.2005.08.009
DOI:10.1016/j.ultras.2003.11.011
DOI:10.1016/S0735-1097(13)60107-2 [Cited within: 1]
DOI:10.1021/acs.langmuir.6b02489
DOI:10.1371/journal.pone.0103606
DOI:10.1371/journal.pone.0051869
DOI:10.1074/jbc.M111.338012
DOI:10.1016/j.bpj.2011.11.3712
DOI:10.1002/jbio.201000093 [Cited within: 1]
DOI:10.1371/journal.pone.0065339 [Cited within: 2]
DOI:10.1016/j.tplants.2015.01.008 [Cited within: 1]
DOI:10.1021/la802529q [Cited within: 1]
DOI:10.1016/j.jcis.2008.08.064
DOI:10.1021/jp805143c [Cited within: 1]
DOI:10.1016/j.colsurfa.2013.06.043 [Cited within: 1]
DOI:10.1271/bbb.63.421 [Cited within: 1]
DOI:10.1039/B917017K [Cited within: 1]
DOI:10.1016/j.jcis.2012.09.077 [Cited within: 1]
DOI:10.1016/j.mineng.2009.02.006 [Cited within: 1]
DOI:10.1016/j.mineng.2014.02.002 [Cited within: 1]
DOI:10.1039/C4RA17257D [Cited within: 3]
DOI:10.1103/PhysRev.125.409 [Cited within: 1]
DOI:10.1063/1.321323 [Cited within: 1]
DOI:10.1126/science.213.4504.209 [Cited within: 1]
DOI:10.1021/jp500953m [Cited within: 3]
DOI:10.1021/jp068665w [Cited within: 3]
DOI:10.1063/1.1747520 [Cited within: 1]
DOI:10.1016/B978-0-12-801530-8.00003-7 [Cited within: 1]
DOI:10.1016/j.ces.2009.10.003 [Cited within: 2]
DOI:10.1063/1.4739528 [Cited within: 1]
DOI:10.1063/1.4739530 [Cited within: 1]
DOI:10.1038/296636a0 [Cited within: 2]
DOI:10.1021/jp911868j [Cited within: 2]
DOI:10.1021/jp4002093 [Cited within: 2]
DOI:10.1021/jp2041795 [Cited within: 1]
DOI:10.1021/acs.langmuir.8b01724 [Cited within: 1]
DOI:10.1021/acs.jpcb.8b11385 [Cited within: 2]
DOI:10.1021/acs.langmuir.8b03113 [Cited within: 2]
DOI:10.1121/1.1907466 [Cited within: 2]
DOI:10.1121/1.1907688 [Cited within: 1]
DOI:10.1021/acs.langmuir.6b01660 [Cited within: 1]
DOI:10.1002/cphc.201100807 [Cited within: 2]
DOI:10.1002/slct.201801504 [Cited within: 2]
DOI:10.1007/s11425-007-0197-y [Cited within: 2]
DOI:10.1039/c3sm50906k [Cited within: 1]
DOI:10.1007/s10483-013-1757-x [Cited within: 2]
DOI:10.1016/j.jcis.2018.10.108 [Cited within: 1]
DOI:10.1021/acs.langmuir.5b04703 [Cited within: 1]
DOI:10.1103/PhysRevLett.99.188301 [Cited within: 1]
DOI:10.1016/j.msec.2012.06.019 [Cited within: 1]
DOI:10.1021/la302674g [Cited within: 1]
DOI:10.1021/acs.langmuir.9b02761 [Cited within: 3]
DOI:10.1016/j.fuel.2015.05.072 [Cited within: 1]
DOI:10.1039/C8SM01949E [Cited within: 1]
DOI:10.1016/j.colsurfa.2005.06.063 [Cited within: 1]
DOI:10.1089/ees.2017.0377 [Cited within: 1]
DOI:10.1016/j.cis.2012.06.012 [Cited within: 1]
DOI:10.1021/jp074260f [Cited within: 1]
DOI:10.1021/acs.jpcb.5b11103 [Cited within: 1]
DOI:10.1021/acs.langmuir.6b01644 [Cited within: 1]
DOI:10.1089/ees.2018.0203 [Cited within: 1]
DOI:10.1007/s11182-019-01618-x [Cited within: 1]
DOI:10.3390/nano6020031 [Cited within: 1]
DOI:10.1002/cphc.201201032 [Cited within: 1]
[Cited within: 1]
DOI:10.1021/acs.jpcc.8b05688
DOI:10.1021/acs.langmuir.9b00144 [Cited within: 1]
DOI:10.1063/1.4773249 [Cited within: 1]
DOI:10.1063/1.4863448
DOI:10.1063/1.4922905
DOI:10.1021/acs.langmuir.5b04162
DOI:10.1063/1.4971207
DOI:10.1063/1.4896937
DOI:10.1063/1.4981788 [Cited within: 1]