1.
Introduction
High-speed vertical-cavity surface-emitting lasers (VCSELs) are important devices in the data center, supercomputer and other optical fiber communication fields, due to its low cost, low consumption, and easy integration[1–5]. Several approaches have been utilized to enhance the modulation speed of VCSEL, such as minimizing electrical parasitics, decreasing self-heating, and minimizing damping[6]. The damping can reduce VCSELs’ small signal modulation response and limit the small signal modulation bandwidth[7, 8]. The main way is to reduce device damping by changing the photon lifetime in the whole cavity. Photon lifetime can be adjusted by changing the reflectivity of the top DBR. In 2011, Anders Larsson et al. utilized an ICP etcher to etch the surface of the top DBR with low RF power and low etching speed. It is found that in the 11 μm oxide-aperture VCSEL the small signal modulation bandwidth is improved from 15 to 23 GHz[6]. In 2016, the top DBR reflectivity is reduced for improving the modulation bandwidth up to 26.5 GHz by growing thin film with low-rate MOCVD[9]. However, all these approaches required expensive equipment with slow and accurate etch or growth speed. It is not suitable for the mass production of the VCSELs. In this paper, we utilize the wet shallow corrosion process to etch the top DBR for changing the damping of 850 nm VCSEL with 9 μm oxide-aperture. Such an approach is much simpler and low cost.
2.
Device structure and experiment
In this paper, the epitaxial structure of the 850 nm VCSEL was fabricated by metal chemical vapor deposition (MOCVD). The n+-GaAs substrate is highly doped. The high-speed 850 nm VCSELs designed with a graded separate confinement heterostructure region (SCH), which contains an active region with three unstrained 7-nm-thick GaAs MQWs separated by 8-nm-thick Al0.3Ga0.7As barriers, and it is sandwiched between p-type and n-type Al0.3Ga0.7As phase compensation layers. The SCH is in the middle of a p-doped distributed Bragg reflector (DBR) containing 20.5 pairs of Al0.12Ga0.88As/Al0.9Ga0.1As and an n-doped DBR containing 34.5 pairs of Al0.9Ga0.1As/Al0.12Ga0.88As. A 30-nm-thick Al0.98Ga0.02As layer is included in the top DBR above the SCH to selective oxidation during fabrication, thus, the transverse optical and electrical field can be confined. The topmost DBR layer is a highly p+-doped GaAs layer with the thickness of 25 nm, which is located on top of the p-type DBR as a low-contact resistance layer.
Fig. 1(a) is the cross-sectional schematic of the high speed 850 nm VCSEL. The structure of the VCSEL is a double mesas and coplanar electrode structure. The mesas were both dry etched using inductively coupled plasma (ICP) with SiCl4 and Cl2 chemistry. Then the oxide apertures were formed in a wet oxidation furnace at 430 °C in a water steam ambient, with an oxidation rate of approximately 0.27 μm/min for the 98% Al-content layers. VCSELs are planarized with benzocyclobutene (BCB), which is used to reduce VCSELs’ parasitic capacitance, and is propitious to heat dissipation[10]. Ti/Au and AuGeNi/Au were sputtered on the top surface to form p- and n-type ohmic contacts by magnetron sputtering, respectively. Then the devices were annealed in a rapid thermal annealing system in an N2 ambient at 430 °C. Coplanar ground-signal-ground (GSG) contacts were applied for direct high-frequency probe measurements, which can avoid parasitic coupling, thus the accuracy of the measurement is improved in the microwave probing test[11]. The fabricated VCSEL is shown in Fig. 1(b).

class="figure_img" id="Figure1"/>
Download
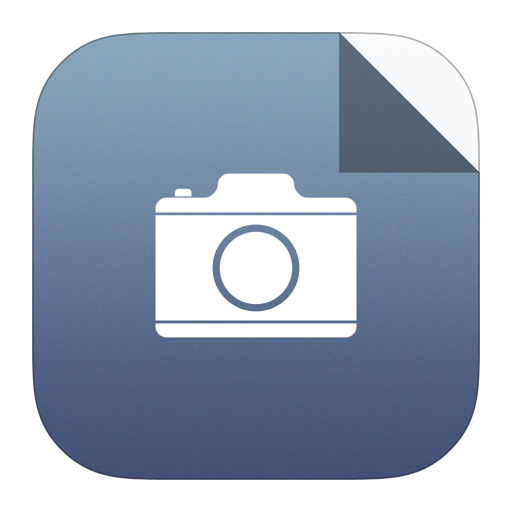
Larger image
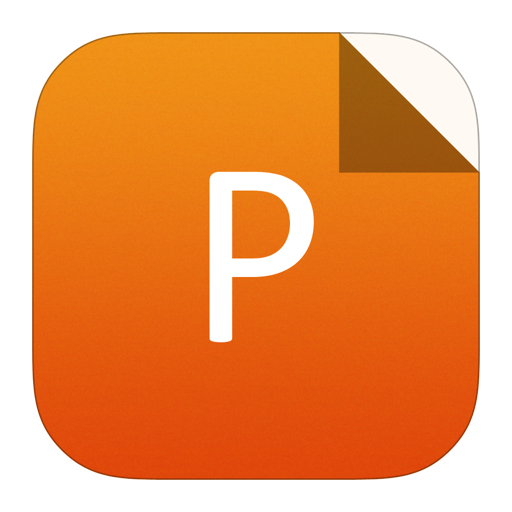
PowerPoint slide
Figure1.
(Color?online) (a) Schematic, cross-sectional view of the high speed VCSEL. (b) Microscope image of fully processed VCSEL on wafer.
After fabrication, the wafer was cleaved into several pieces, and then wet-etch shallowly the surface to reduce its reflectivity. The photon lifetime of VCSEL can be changed by wet-etch. The relation between reflectivity and photon lifetime can be written as[7]
$$frac{1}{{{tau _{ m{p}}}}} = alpha _{ m i} + frac{1}{L_{ m eff}/v_{ m g}}ln frac{1}{{sqrt {R_{ m T}R_{ m B}} }}.$$ ![]() | (1) |
The relation between photon lifetime and damping can be written as[6]
$$gamma = 4{pi ^2}left( {tau _{ m p} + frac{{varepsilon chi }}{{{nu _{ m g}}{{partial g} / {partial n}}}}} ight) f_{ m r}^2 + {gamma _0},$$ ![]() | (2) |
with
$$K = 4{pi ^2}left( {{tau _{ m p}} + frac{{varepsilon chi }}{{{nu _{ m g}}{{partial g} / {partial n}}}}} ight),$$ ![]() | (3) |
where Leff is the effective cavity length, RT is the top DBR reflectivity, RB is the bottom DBR reflectivity, ε is the gain compression factor, and χ is the transport factor. vg is the group velocity. As can be seen in Eqs. (1)–(3), top DBR reflectivity can be used to change the damping.
Fig. 2 is the top DBR reflectivity calculated as a function of etch depth into the top DBR. The structure parameters of the VCSEL, which are given in part 2, are taken into the index model to calculate the reflectivity[12]. According to theoretical calculation, as the etch depth increases, top DBR reflectivity decreases from 0.9991 at 0 nm to 0.9886 at 59.7 nm. The range of etch depth was settled between 0 and 60 nm. We etched three different depths: ~25, ~40, and ~55 nm. The formula of corrosion liquid is sulphuric acid, hydrogen peroxide and deionized water. Its volume ratio is 3 : 1 : 100. The corrosion rate is tested by corrode dummy GaAs samples. When ambient temperature is 21 ± 1 °C, the corrosion rate is ~0.51 nm/s.

class="figure_img" id="Figure2"/>
Download
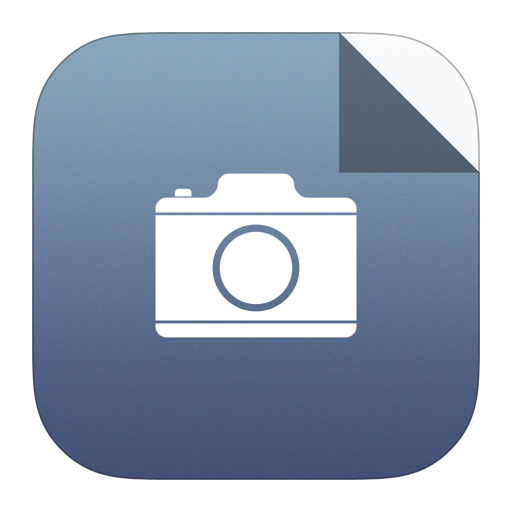
Larger image
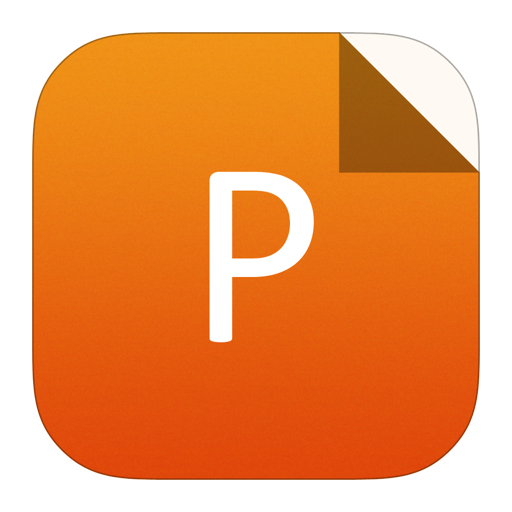
PowerPoint slide
Figure2.
Calculated top DBR reflectivity as a function of etch depth into the top DBR.
3.
Result and analysis
Fig. 3 is the P–I characteristics of the 9 μm oxide-aperture VCSEL with different etch depths and a close-up to the threshold region. As the etch depth increases, the maximum output power and SE (slope efficiency) are: 4.03 mW and 0.399 W/A at detch = 0 nm; 4.70 mW and 0.421 W/A at detch = ~25 nm; 6.35 mW and 0.547 W/A at detch = ~40 nm; and 7.20 mW and 0.568 W/A at detch = ~55 nm. More light related with a reduction of reflectivity is coupled out from the resonator, so an increasing of output power and SE is observed. It can be seen from the inset, the threshold increases with etch depth. At a higher optical loss rate, more carriers are required to reach the threshold, and therefore, the threshold current increases with etch depth, rising from 0.565 mA at detch = 0 nm to 1.570 mA at detch = ~55 nm. Because of the increasing of optical loss rate, less light is reflected back to the internal resonator, and the internal absorption contributes less to self-heating[13]. We observed an increase of thermal roll-over current with etch depth, it increases from 14.4 mA at detch = 0 nm to 18.5 mA at detch = ~55 nm. The resistance of the VCSEL cannot be changed by corrosion of the top layer DBR, so the I–V characteristics are the same before and after shallow etching.

class="figure_img" id="Figure3"/>
Download
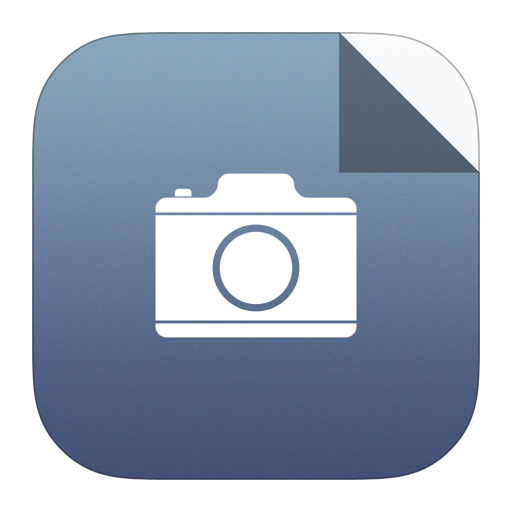
Larger image
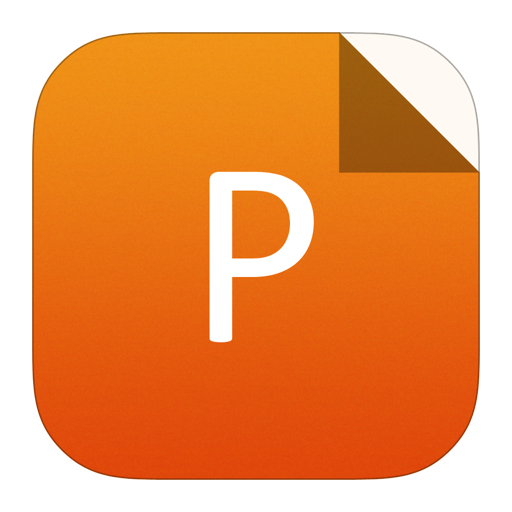
PowerPoint slide
Figure3.
(Color?online) Output power versus current for the 9 μm oxide-aperture VCSELs with different etch depth. Inset: close-up of the threshold region.
The small signal modulation response (S21) is measured by using a 40 GHz vector network analyzer (R&S ZVA 40). A testing system[14] is built. The driving current is supplied by a DC source which was connected with the network analyzer. Then the network analyzer supplies the RF signal, which is combined with the driving current. Both the driving current and RF signal are transmitted by a high frequency microwave probe to the VCSEL, and the output power is coupled by a multimode fiber connected to a 25 GHz detector (New Focus 1414-50).
Fig. 4 is small signal modulation bandwidth of the 9 μm oxide-aperture VCSEL with different etch depths at room temperature. The common three-pole transfer function is given by[15]:
$$Hleft( f ight) ={ m {const}} cdot frac{{f_{ m r}^2}}{{f_{ m r}^2 - {f^2} + jleft( {{f / {2pi }}} ight)gamma }} cdot frac{1}{{1 + jleft( {{f / {{f_{ m p}}}}} ight)}},$$ ![]() | (4) |
where fr is relaxation oscillation frequency, γ is damping, and fp is parasitic frequency. It is used for fittings to the measured response and then to extract its fr, γ as a function of the current.
Fig. 5(a) is the damping rate plotted against resonance frequency squared. The damping increases with the resonance frequency and sets an intrinsic upper limit to the modulation bandwidth. The rate of damping increasing is qualified by the K factor[6]. From Fig. 5(a), a nonlinear relation between γ and fr2 is observed at high resonance frequency. This is due to a changing of photon lifetime. When the carrier injection level is high, it causes severe self-heating due to internal absorption[16], which influences the photon lifetime. Thus, the K factor is extracted from the liner regime at lower resonance frequency, where the self-heating is negligible. The extracted K factor at different etch depth is shown in Fig. 5(b). A reduction of the K factor is observed as etch depth increases due to the decrease of photon lifetime.
As the K factor decreases, the damping also decreases. In theory, the small signal modulation bandwidth should be increased. In Fig. 4, we can observe an increase of bandwidth at detch = ~25 nm, and also a decrease at detch = ~40 nm and ~55 nm.

class="figure_img" id="Figure4"/>
Download
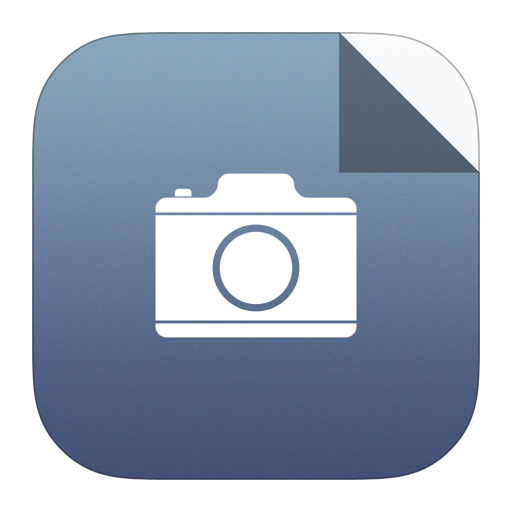
Larger image
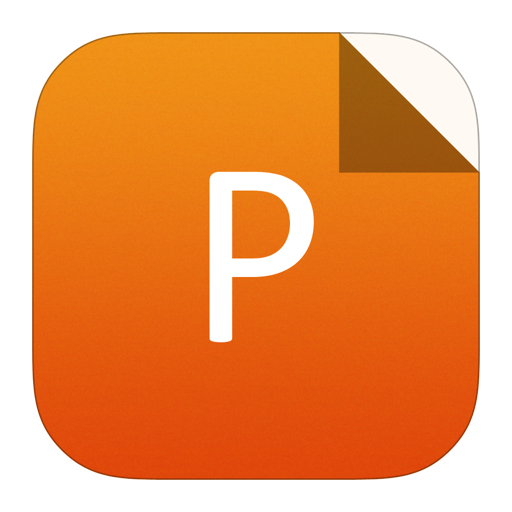
PowerPoint slide
Figure4.
(Color?online) Small signal modulation bandwidth of the 9 μm oxide-aperture VCSEL with different etch depths at room temperature.
The resonance frequency is extracted and plotted against the square root of bias current above the threshold for VCSELs at different etch depths in Fig. 5(c). The dependence of the resonance frequency on current can be expressed as[17]
$${f_{ m r}} = Dsqrt {I - {I_{ m th}}} ,$$ ![]() | (5) |
with
$$D = frac{1}{{2pi }} sqrt {frac{{{eta _{ m i}}varGamma {nu _{ m g}}}}{{q{V_{ m a}}}} frac{{{{partial g} / {partial n}}}}{chi }} ,$$ ![]() | (6) |
where ηi is internal quantum efficiency, Γ is the optical confinement factor, and υg is the group velocity.
The liner fit of fr to
m th}}} $

$$frac{{partial g}}{{partial n}} = {left( {2pi D} ight)^2} frac{{q{V_{ m a}}chi }}{{{eta _{ m i}}varGamma {nu _{ m g}}}},$$ ![]() | (7) |
assuming ηi = 0.8, vg = 8.8 × 109 cm/s, χ = 1[18], and taking Va from the epitaxial layer specification, the differential gain is calculated from the D factor.
In Fig. 5(d), as the etch depth increases, the differential gain is reduced due to the increase of threshold current, associated with the increase of mirror loss. At detch = 0 nm, the differential gain is 13.051 × 10?16 cm2. At detch = ~55 nm, the differential gain is reduced to 4.767 × 10?16 cm2. The decrease of the differential gain leads to the decrease of the resonance frequency, and also limits the small signal modulation bandwidth.

class="figure_img" id="Figure5"/>
Download
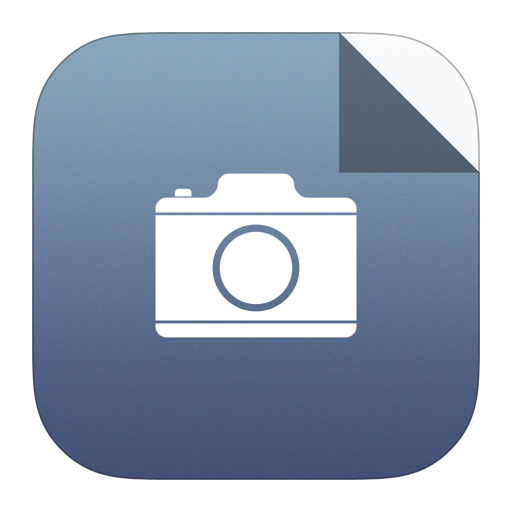
Larger image
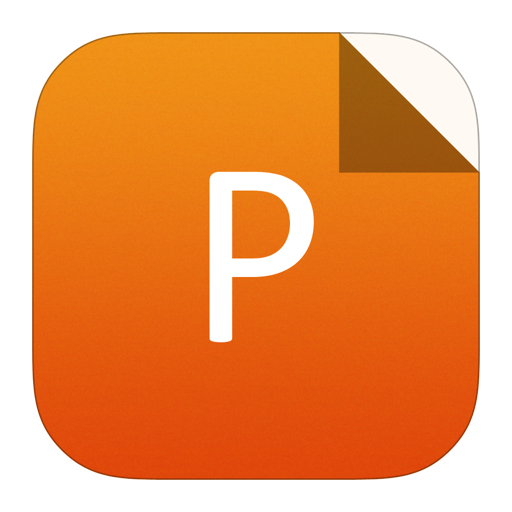
PowerPoint slide
Figure5.
(Color?online) (a) Damping rate versus resonance frequency squared. (b) K-factor values for 9 μm oxide-aperture VCSELs with different etch depth. (c) Resonance frequency versus square root of bias current density above the threshold for the 9-μm oxide aperture VCSELs with different etch depths. Fits for extracting the D-factor are indicated. (d) Differential gain versus etch depth for the 9-μm aperture VCSEL calculated from the D-factor.
The damping of VCSELs is reduced by wet-etching the surface of the top DBR. It is found that the small signal modulation bandwidth of 9 μm oxide-aperture VCSEL has maximum growth at detch = ~25 nm, its bandwidth increases by approximately 1 GHz. At 0 and ~25 nm, damping is the dominant factor to limit the small signal modulation bandwidth. So when the damping is decreased, the limitation is weak, modulation bandwidth increases. At somewhere between ~25 and ~40 nm, the differential gain becomes the dominant factor to limit the bandwidth. When the etch depth continues to increase, differential gain is the dominant factor to limit the bandwidth.
4.
Conclusion
We have investigated the impact of damping on high-speed 850 nm VCSEL performance. The damping of VCSEL is changed by wet etching the top DBR. According to theoretical calculation, the reflectivity of the top DBR decreases from 0.9991 at an etch depth of 0 nm to 0.9886 at an etch depth of 59.7 nm. It makes the K factor decreased, and damping is reduced correspondingly. As the etch depth increases, the different gain is reduced which leads to a reduction of small signal modulation bandwidth, and the damping is also reduced which could partly increase the small signal modulation bandwidth. We conclude that there is a tradeoff between the damping and differential gain, which makes the VCSEL have a maximum modulation bandwidth. Finally, we demonstrated a modulation bandwidth increase from 15.46 to 16.37 GHz using a damping-optimized VCSEL.