1.
Introduction
As transistors scaling into the nanoscale, self-heating effects (SHE) have become more and more severe, especially in non-planar devices such as FinFETs and nanowires due to the increased power density[1–3] and the deteriorated heat dissipation ability[4–7]. SHE has become a key factor concerned in device manufacture[8, 9] and circuit design[10, 11]. SHE can lead to serious device performance degradation and reliability problems[12, 13]. Many works[14–16] have investigated and characterized SHE in semiconductor devices experimentally and theoretically. The performance of semiconductor devices is sensitive to ambient temperature (TA). When TA increases, the improvement in electrostatic properties[17] and the degradation in carrier transport[18, 19] occur simultaneously, which make it complicated to study the SHE. The energy relaxation time in silicon is 0.8 ps[20]. The electron energy-relaxation-length can be 80 nm when considering the saturation velocity in silicon 107 cm/s. Therefore, in nanoscale devices, many thermal non-equilibrium carriers can reach the drain end and take away a part of input power into MOL and BEOL[21], which have an impact on the heat generation in devices. Monte Carlo (MC) simulation can perform the behaviors of thermal non-equilibrium carriers and get the non-local heat generation, which is more accurate than traditional continuum simulation methods[7, 22] in describing nanoscale devices[1].
In this work, we use a well-established electro-thermal Monte Carlo simulation framework to investigate SHE in 14 nm-node FinFETs with TA from 220 to 400 K[23]. Impurity freeze-out effects do not happen in this TA range[24]. We first investigate the heat generation under different operating voltages. Then we further explain the existence of serious non-equilibrium transport by investigating thermal power densities. What is more, we investigate the impact of TA on current degradation, ballistic factor degradation caused by SHE and the thermal resistances. This work indicates the importance of TA in studying SHE in nanoscale devices.
2.
Device structure and simulation method
Fig. 1 shows the device structure of the 14 nm-node Si bulk nFinFET[25] used in this work. HfO2/TiAlN is utilized as the high-k/metal-gate in nFinFET. The device parameters are listed in Table 1[25, 26].
Strcuture parameter | Description | Value |
Hsd | Raised S/D height | 52 nm |
Hstop | Stop layer height | 40 nm |
Hsub | Substrate layer height | 4 μm |
Hfin | Fin height | 42 nm |
Lsd | Raised S/D length | 20 nm |
Lext | S/D length | 10 nm |
Lg | Gate length | 20 nm |
Wpitch | Fin pitch | 42 nm |
Wfin | Fin width | 8 nm |
Nchannel | Channel doping, p type | 1 × 1015 cm?3 |
Nstop | Stop layer doping, p type | 2 × 1018 cm?3 |
Nsd | Source/Drain doping, n type | 1 × 1020 cm?3 |
Nsub | Substrate doping, p type | 1 × 1015 cm?3 |
EOT | Equivalent oxide thickness | 0.85 nm |
Tox | Gate oxide thickness | 5.4 nm |
Table1.
Structure parameters of the 14 nm-node nFinFET.
Table options
-->
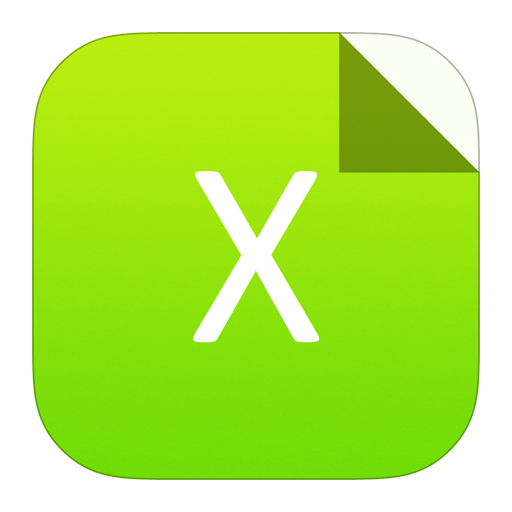
Download as CSV
Strcuture parameter | Description | Value |
Hsd | Raised S/D height | 52 nm |
Hstop | Stop layer height | 40 nm |
Hsub | Substrate layer height | 4 μm |
Hfin | Fin height | 42 nm |
Lsd | Raised S/D length | 20 nm |
Lext | S/D length | 10 nm |
Lg | Gate length | 20 nm |
Wpitch | Fin pitch | 42 nm |
Wfin | Fin width | 8 nm |
Nchannel | Channel doping, p type | 1 × 1015 cm?3 |
Nstop | Stop layer doping, p type | 2 × 1018 cm?3 |
Nsd | Source/Drain doping, n type | 1 × 1020 cm?3 |
Nsub | Substrate doping, p type | 1 × 1015 cm?3 |
EOT | Equivalent oxide thickness | 0.85 nm |
Tox | Gate oxide thickness | 5.4 nm |

class="figure_img" id="Figure1"/>
Download
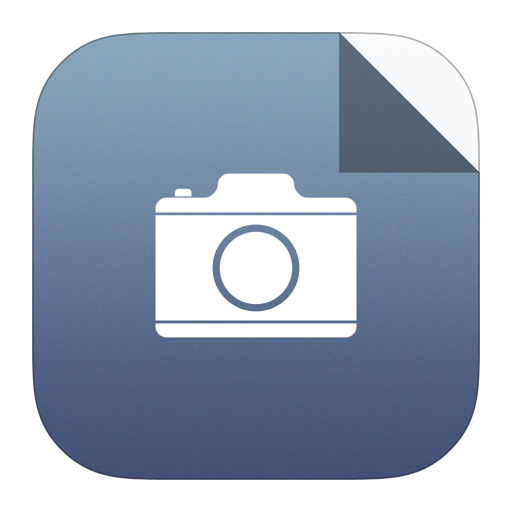
Larger image
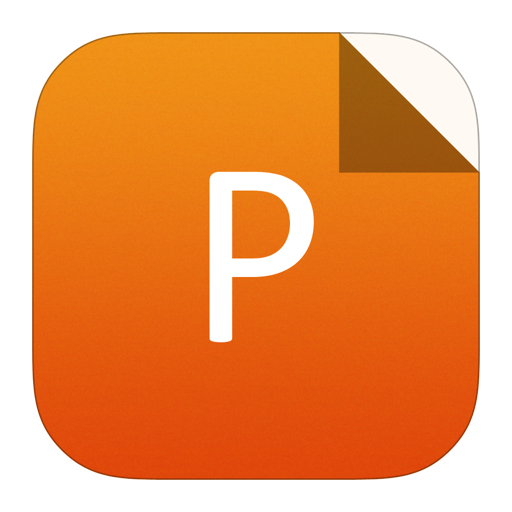
PowerPoint slide
Figure1.
(Color online) The 14 nm-node Si bulk nFinFET structure used in this work.
Fig. 2 shows the electro-thermal simulation framework used in this work. Our in-house three-dimensional (3D) full-band ensemble MC simulator[27, 28] and 3D Fourier heat conduction equation solver are used to perform the electro-thermal simulation. In this work, the operating voltage is less than 1 V. Therefore, the energy of most carriers is smaller than the bandgap of silicon (1.12 eV) and the generation–recombination (G–R) process can be ignored[17]. Only the heat generation from the carrier-phonon scatterings is taken into account. Thermal convection is not considered inside the solid-state devices and thermal radiation can be ignored due to the non-existence of the G–R process in this situation. We only utilize heat conduction to obtain the spatial temperature distribution.

class="figure_img" id="Figure2"/>
Download
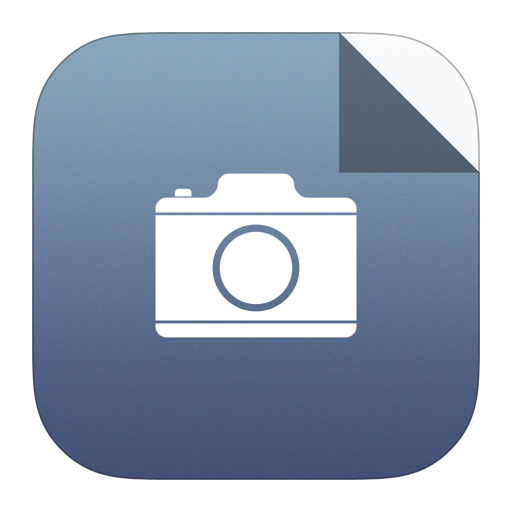
Larger image
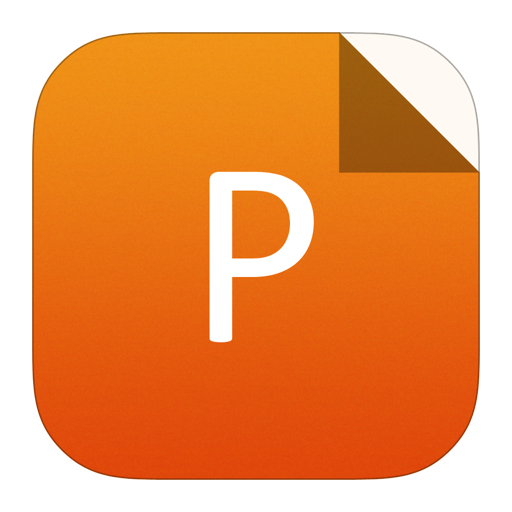
PowerPoint slide
Figure2.
(Color online) Electro-thermal simulation framework used in this work.
The simulation flow begins with a self-consistent MC simulation at a user-defined TA, which is to achieve the steady electric profiles including potential profile and carrier profile, which are results without SHE. After that, a fixed-potential MC simulation is performed based on the steady electric profiles to achieve heat spatial distribution, which can eliminate errors from unphysical oscillations due to the MC nature in the self-consistent mode. The heat profile is achieved by counting the phonon emission (Eem) and absorption (Eab), which is then sent to the heat conduction equation solver to achieve the temperature profile. To achieve the electric profiles with SHE, the temperature profile is then fed back to a new MC simulation with the same operating voltages to get the degraded electric properties where electric profiles in the previous loop are used as the initial condition. Steady results with SHE can be achieved after 2–3 times coupling loops.
The full band structure of silicon is considered in our MC simulator. It is obtained by the empirical pseudopotential calculation including spin-orbit interactions. Besides, main scattering mechanisms including acoustic and optical phonon scattering, ionized impurity scattering, impact ionization scattering and surface roughness scattering are involved, which are verified and calibrated in Refs. [29, 30].
The carrier-phonon scatterings are calculated according to Ref. [31]. The inter-valley acoustic phonon scatterings are treated in accordance with the optical phonon scatterings with constant deformation potentials, which are expressed in Eq. (1). The corresponding information of inter-valley acoustic phonons and optical phonons are listed in Table 2.
$$begin{split}{S_{text{η} , ,,{ m b}', ,,{ m b}}}left( {{ {k}'} { m{|}} { {k}}} ight) = frac{pi }{{{ m{Omega }}varrho {omega _eta }}}{left( {{D_t}{K_eta }} ight)^2}left( {nleft( {hbar {omega _eta }} ight) + frac{1}{2} pm frac{1}{2}} ight)delta left( {{epsilon _{ m b'}}left( {{ {k}'} } ight) - {epsilon _{ m b}}left( { {k}} ight),, pm,, hbar {omega _eta }} ight)rleft( {eta ,nu left( {{ {k}'} } ight),nu left( { {k}} ight)} ight),end{split}$$ ![]() | (1) |
where Ω and ? are the normalized volume in k space and the mass density of silicon, respectively. η represents the phonon type. ωη is the wave vector of phonons.


ight),nu left( { {k}}
ight)}
ight)$

η | Phonon type | DtK (1010 eV/m) | $ hbar omega $ (meV) | r |
1 | TA | 0.47 | 12.1 | g |
2 | LA | 0.74 | 18.5 | g |
3 | LO | 10.23 | 62.0 | g |
4 | TA | 0.28 | 19.0 | r |
5 | LA | 1.86 | 47.4 | r |
6 | TO | 1.86 | 58.6 | r |
Table2.
Optical phonon and inter-valley acoustic phonon in silicon.
Table options
-->
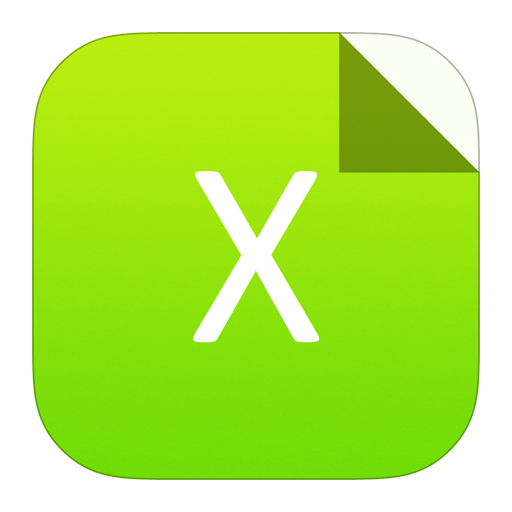
Download as CSV
η | Phonon type | DtK (1010 eV/m) | $ hbar omega $ (meV) | r |
1 | TA | 0.47 | 12.1 | g |
2 | LA | 0.74 | 18.5 | g |
3 | LO | 10.23 | 62.0 | g |
4 | TA | 0.28 | 19.0 | r |
5 | LA | 1.86 | 47.4 | r |
6 | TO | 1.86 | 58.6 | r |
The intra-valley acoustic phonon scattering is regarded as an elastic scattering due to its small phonon vector, which is expressed in Eq. (2).
$${S_{text{η} ,,,{ m b}',,, { m b}}}left( { {k'} { m{|}}{ k}} ight) = frac{{2pi {K_{ m B}}T{{ m{Xi }}^2}}}{{{ m{Omega }}hbar varrho {u^2}}}delta left( {{epsilon _{{ m b}'}}left( { {k'} } ight) - {epsilon _{ m b}}left( { k} ight)} ight){delta _{nu left( { {k'} ,,, { k}} ight)}},$$ ![]() | (2) |
where the deformation potential Ξ is 8.37 eV and the phonon group velocity is u = 9050 m/s for longitudinal phonons and 5230 m/s for transversal phonons. KB and

The impacts of temperature on electrostatic properties and carrier transport are well considered in our simulator, which can be seen in Fig. 3.

class="figure_img" id="Figure3"/>
Download
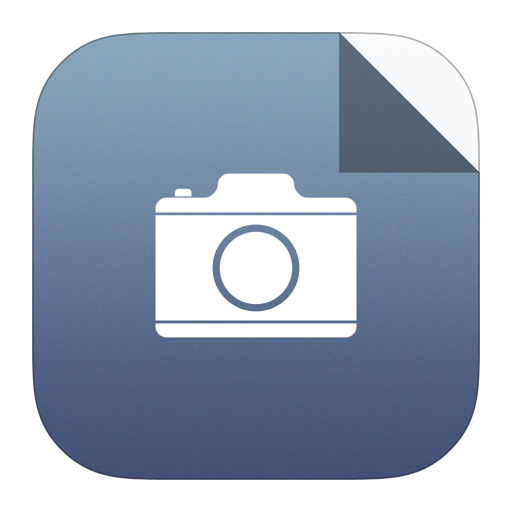
Larger image
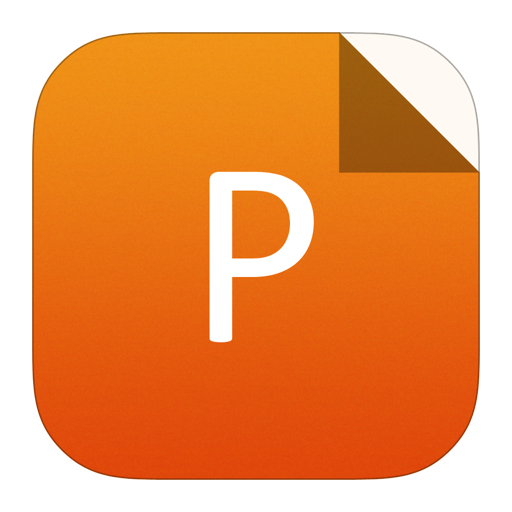
PowerPoint slide
Figure3.
(Color online) (a) Intrinsic carrier density versus temperature. Data in Ref. [17] is shown for comparison. (b) Electro-phonon scattering rate versus kinetic energy at different temperatures. Data at TA = 300 K in Ref. [18] is shown for comparison.
It is difficult to analyze thermal issues in modern packaged ICs due to the complicated interconnects and package structures[32]. To solve this problem, thermal analysis in components of modern ICs are widely advancing by abstracting the other components into proper boundary conditions, such as thermal analysis in interconnects[32, 33], package structures[34, 35] and devices[7, 22]. In the thermal analysis of our work, the impacts of interconnect, package structure and substrate on devices are abstracted as the impact of TA. In the simulated structure, the source (S), drain (D), gate (G) and substrate (B) are the four main heat dissipation paths while other boundaries are adiabatic. Si/Metal contact thermal resistance[36] is used at the S/D/B contact. The summation of Si/metal contact thermal resistance and Si/SiO2 contact thermal resistance[37] is used at the G contact. The essential thermal parameters are listed in Table 3[36–41]. The area of four contacts respectively are HsdWpitch for S/D, (2Hfin + Wfin + 4Tox)Lg for G and (2Lsd + 2Lext + Lg)Wpitch for B. The final heat flux percentages of the four heat dissipation paths are 61.23% (D), 21.85% (S), 8.63% (G) and 8.29% (S) when Vgs = Vds = 0.7 V, TA = 300 K, which meets the heat dissipation requirement of a normal working device[32, 42].
Silicon thermal conductivity (anisotropic) | |
ky in Region (1–6) | 15 Wm?1K?1 |
kx, kz in Region (1–6) | 10 Wm?1K?1 |
Silicon thermal conductivity (isotropic) | |
k in Region (7) | 148 Wm?1K?1 |
Oxide thermal conductivity (isotropic) | |
Trench oxide (SiO2) | 1.4 Wm?1K?1 |
Gate oxide (HfO2) | 1.1 Wm?1K?1 |
Contact thermal resistance | |
Gate | 2.5 × 10?4 cm2K/W |
Source/Drain/Substrate | 0.5 × 10?4 cm2K/W |
Table3.
Thermal parameters used in this work.
Table options
-->
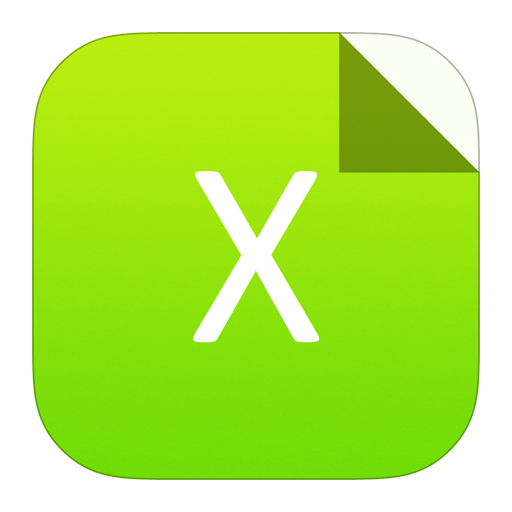
Download as CSV
Silicon thermal conductivity (anisotropic) | |
ky in Region (1–6) | 15 Wm?1K?1 |
kx, kz in Region (1–6) | 10 Wm?1K?1 |
Silicon thermal conductivity (isotropic) | |
k in Region (7) | 148 Wm?1K?1 |
Oxide thermal conductivity (isotropic) | |
Trench oxide (SiO2) | 1.4 Wm?1K?1 |
Gate oxide (HfO2) | 1.1 Wm?1K?1 |
Contact thermal resistance | |
Gate | 2.5 × 10?4 cm2K/W |
Source/Drain/Substrate | 0.5 × 10?4 cm2K/W |
Fig. 4 shows the simulated and experimental[25] Ids–Vds curves of the 14 nm-node FinFET, which indicates that the MC results can well reproduce the experimental data.

class="figure_img" id="Figure4"/>
Download
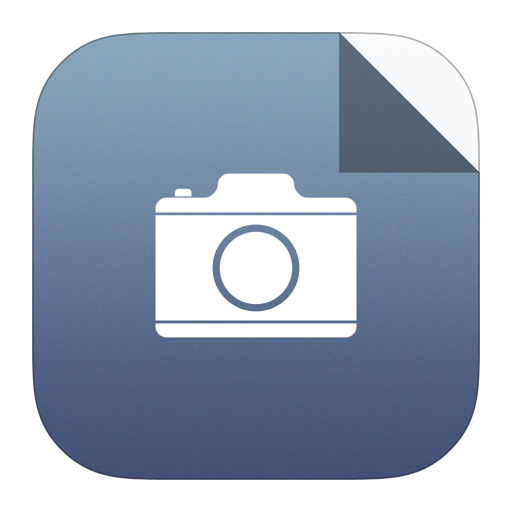
Larger image
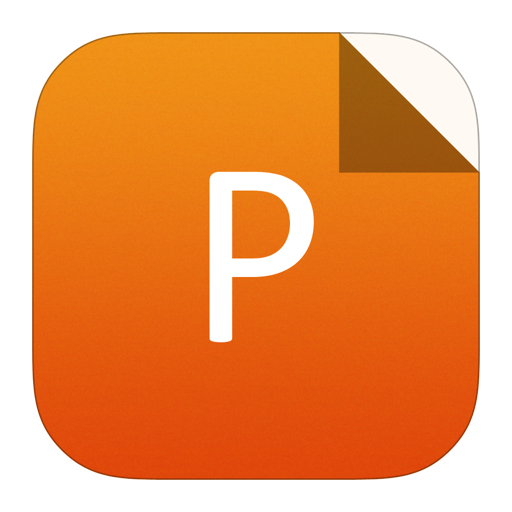
PowerPoint slide
Figure4.
(Color online) Calibration of 14 nm-node Si nFinFET simulation results with DC experimental data.
3.
Results and discussions
3.1
Heat generation
Fig. 5 shows the average electron energy distribution along the transport direction at Vgs = 0.7 V, Vds = 1 V and TA = 300 K. It clearly demonstrates the existence of thermal non-equilibrium carriers at the drain end. A part of input power can be carried out by the thermal non-equilibrium carriers, which can eventually heat up MOL and BEOL.

class="figure_img" id="Figure5"/>
Download
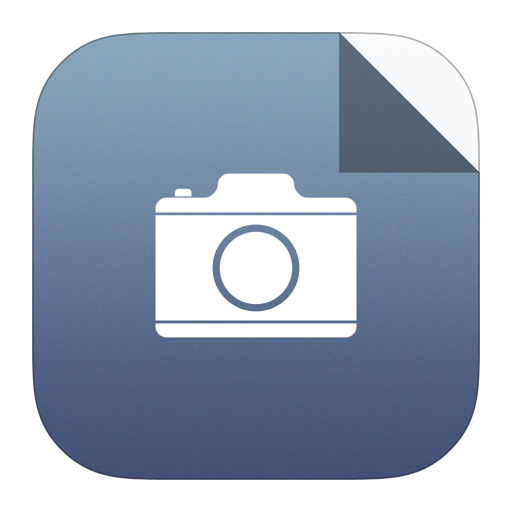
Larger image
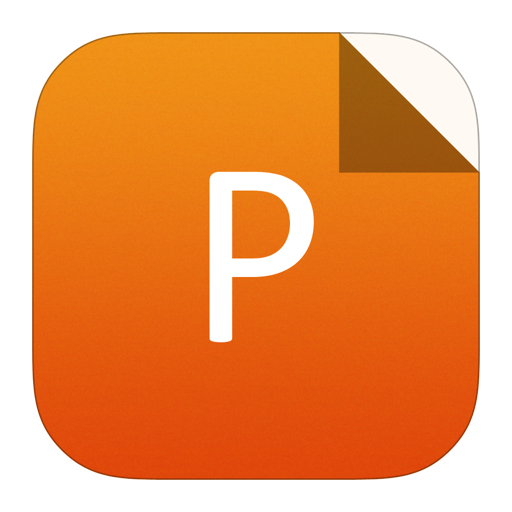
PowerPoint slide
Figure5.
(Color online) The average electron energy distribution along the transport direction at Vgs = 0.7 V, Vds = 1 V and TA = 300 K. The thermal equilibrium carrier energy is 1.5 KBT (0.0389 eV).
Fig. 6(a) shows the input power Qinput and the heat generation Qheat under different Vds at TA = 220, 300, and 400 K. Here Vgs keeps 0.7 V. Qinput is calculated as IdsVds and Qheat is the total heat generation in the device region by counting the energy exchanged in electron-phonon interactions as in the phonon’s perspective. The figure shows that TA has little impact on the Qinput in this situation, which is the result of the compensation of the increased carrier density and the degenerated carrier transport[43]. However, the Qheat is larger at a higher TA, which is due to more serious electron–phonon interactions at a higherTA. Fig. 6(b) shows the corresponding ratios of Qheat in Qinput. The ratio decreases with the increasing Vds. Under the same Vds, a higher TA leads to a larger ratio of input power turning into heat generation, which can lead to more serious SHE. With Vgs = 0.7 V, Vds = 0.7 V at TA = 300 K, the ratio is 67.7% and it implies that 32.3% of Qinput is carried out of the device region by thermal non-equilibrium carriers. When Vgs = 0.7 V and Vds = 1 V at TA = 220 K, the energy carried out by thermal non-equilibrium carriers can rise up to 40.9% of Qinput due to less electro–phonon interactions.
Fig. 7(a) shows the Qinput and Qheat under different Vgs at TA = 220, 300, and 400 K. Here Vds keeps 0.7 V. The impact of TA on Qheat becomes smaller with the increase of Vgs. Fig. 7(b) shows the corresponding ratios of Qheat in Qinput. The ratio decreases with the increasing Vgs. When Vgs = 1 V and Vds = 0.7 V at TA = 220 K, the ratio is 47.9% and 52.1% of Qinput is carried out of the device region by thermal non-equilibrium carriers. Both Figs. 6 and 7 indicate the existence of serious non-equilibrium transport in 14 nm-node FinFETs that should be necessarily concerned.

class="figure_img" id="Figure6"/>
Download
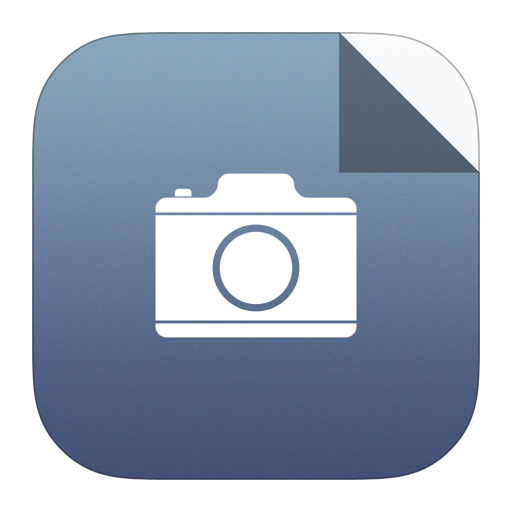
Larger image
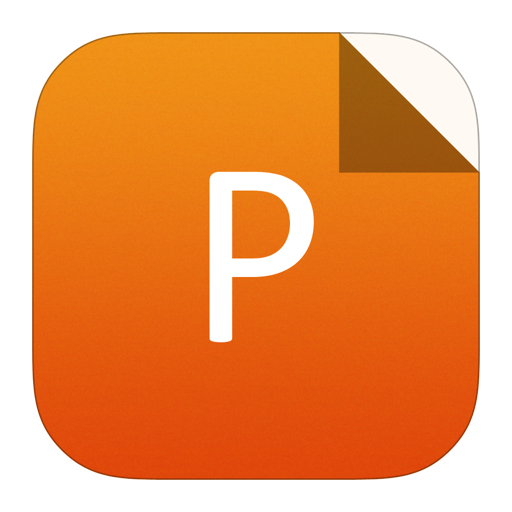
PowerPoint slide
Figure6.
(Color online) (a) The input power Qinput and heat generation Qheat under different Vds and (b) the corresponding ratios of Qheat in Qinput at TA = 220, 300, 400 K and Vgs = 0.7 V.

class="figure_img" id="Figure7"/>
Download
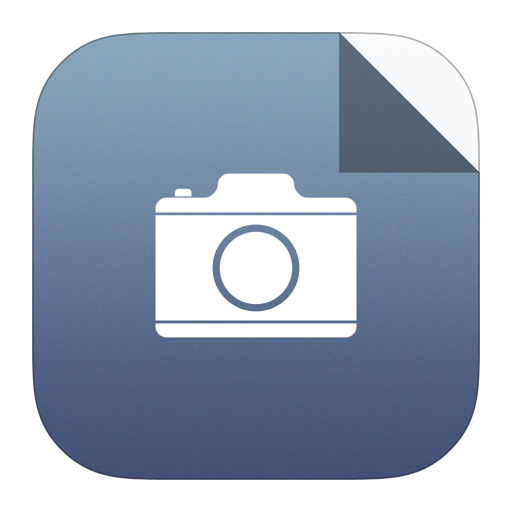
Larger image
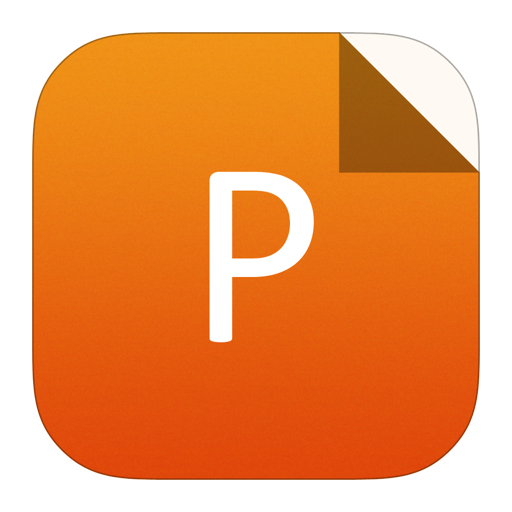
PowerPoint slide
Figure7.
(Color online) (a) The input power Qinput and heat generation Qheat under different Vgs and (b) the corresponding ratios of Qheat in Qinput at TA = 220, 300, 400 K and Vds = 0.7 V.
3.2
Impact of ambient temperature on SHE
The SHE of the 14 nm-node FinFET at different TA is evaluated. The temperature distributions with the corresponding power densities along the transport direction at TA = 220, 300, and 400 K are investigated. Comparisons of results at Vds = 0.7 V with Vds = 1 V under the same Vgs are shown in Figs. 8(a) and 8(b), and the comparisons of results at Vgs = 0.7 V with Vgs = 1 V under the same Vds are shown in Fig. 8(c) and 8(d). From Figs. 8(a) and 8(b), we can see that the change of TA leads to a larger difference in power density and temperature distribution at a larger Vds, which reveals a more serious non-equilibrium transport exists at a larger Vds. On the contrary, the change of TA leads to a smaller difference at a larger Vgs, which is shown in Figs. 8(c) and 8(d). It indicates that there is a smaller difference in the non-equilibrium transport at a larger Vgs under different TA. The increase of Vgs primarily increases the carrier density in the inversion layer.

class="figure_img" id="Figure8"/>
Download
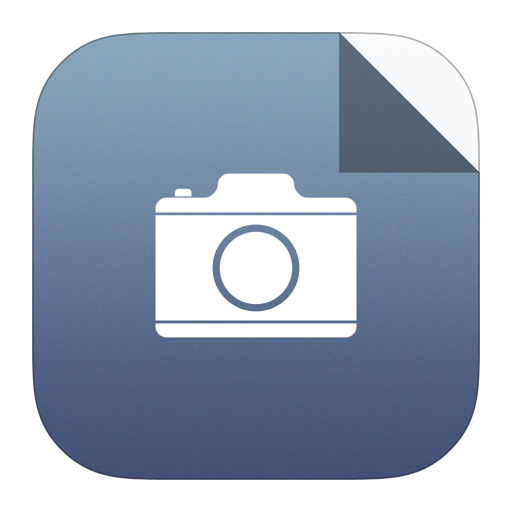
Larger image
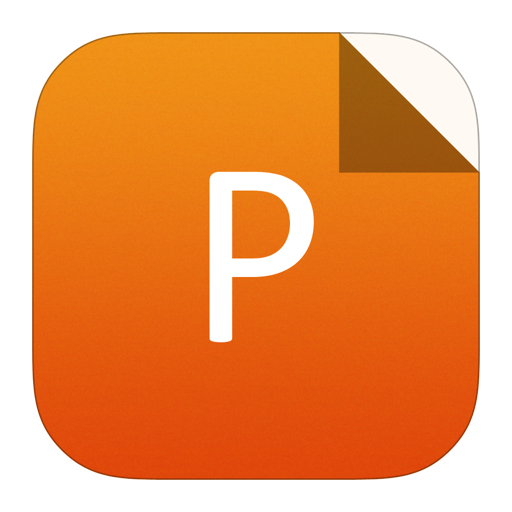
PowerPoint slide
Figure8.
(Color online) (a) The average augments of lattice temperature and (b) the power densities along the transport direction comparing Vds = 0.7 V and Vds = 1 V at Vgs = 0.7 V, (c) the average augments of lattice temperature and (d) the power densities along the transport direction comparing Vgs = 0.7 V and Vgs = 1 V at Vds = 0.7 V. TA = 220, 300 and 400 K.
Fig. 9(a) shows the current densities with and without SHE with different Vds at Vgs = 0.7 V, TA = 220, 300, and 400 K and the corresponding current degradation percentages are shown in Fig. 9(b). The current degradation increases with the increase of Vds. We can see that the current degradation is larger at a higher TA, which indicates a more serious SHE. When Vgs = 0.7 V, Vds = 0.7 V and TA = 300 K, the current degradation percentage is 2.5% and it can be 3.5% when TA = 400 K. Once Vgs = 0.7 V, Vds = 1 V and TA = 400 K, the current degradation percentage can be 4%.

class="figure_img" id="Figure9"/>
Download
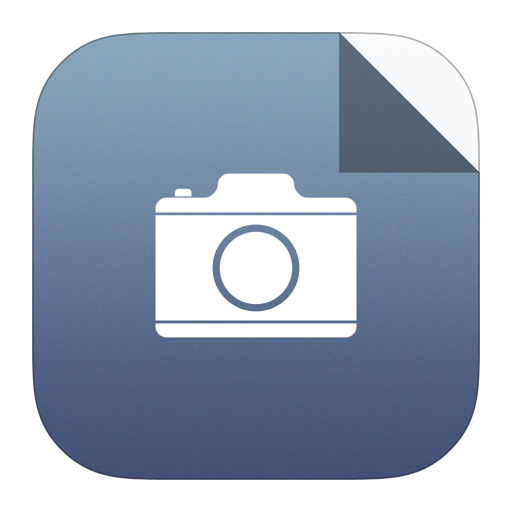
Larger image
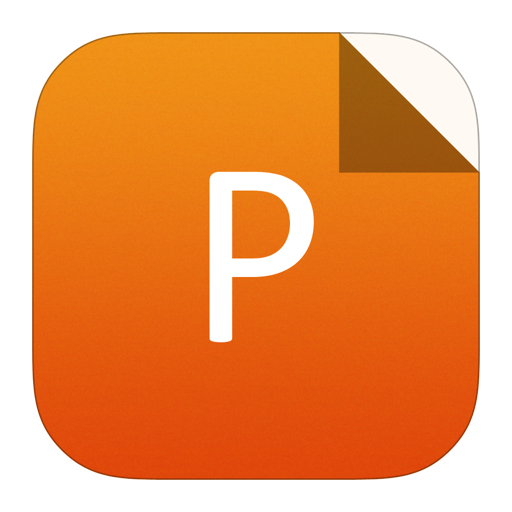
PowerPoint slide
Figure9.
(Color online) (a) Comparison of current densities considering SHE with those not considering SHE under different Vds and (b) the corresponding current degradation percentages caused by SHE at TA = 220, 300, 400 K and Vgs = 0.7 V.
Fig. 10(a) shows the current densities with and without SHE under different Vgs at Vds = 0.7 V, TA = 220, 300, and 400 K. The corresponding current degradation by SHE is shown in Fig. 10(b). The current degradation increases with the increase of Vgs. When Vgs = 1 V, Vds = 0.7 V and TA = 400 K, the current degradation can reach to 8.9%, which implies serious SHE.
Due to its more serious SHE, we then choose the situation with fixed Vds and different Vgs to continue our investigation.
The definition of ballistic factor is as follows[44]:
$${B_{ m {sat}}} = frac{{{I_{ m {ON}}}}}{{{I_{ m {BL}}}}},$$ ![]() | (3) |
where IBL is the ballistic current with no scattering events in the channel and ION is the normal device current with scattering events in the channel.
Bsat = 1 represents the full ballistic transport and smaller Bsat means more scattering events happening in the channel. Fig. 11(a) shows Bsat with and without SHE under different Vgs at TA = 220, 300, 400 K and Vds = 0.7 V. SHE leads to the increase of the local lattice temperature, which can lead to more scattering events and a smaller Bsat. This is clearly shown in Fig. 11(a). SHE can weaken the non-equilibrium transport to be more diffusive. Fig. 11(b) shows the degradation percentage of Bsat. The absolute value of Bsat degradation percentage increases with the increase of Vgs and it is larger at a larger TA when considering the same Vgs. It also indicates that SHE is more serious at a larger TA.

class="figure_img" id="Figure11"/>
Download
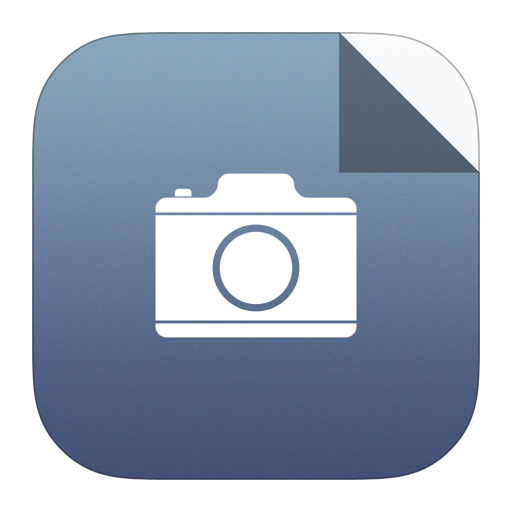
Larger image
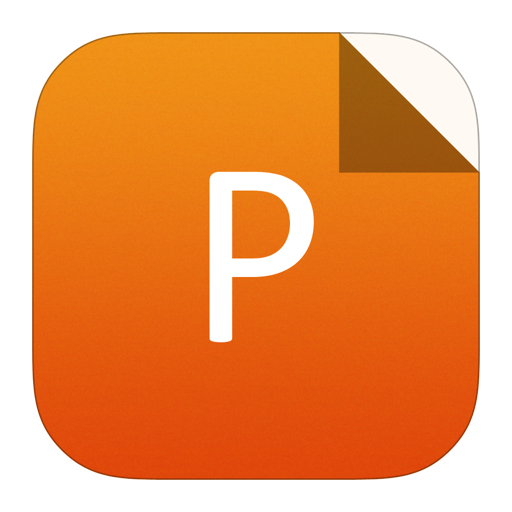
PowerPoint slide
Figure11.
(Color online) (a) Comparison of ballistic factor Bsat considering SHE with that not considering SHE under different Vgs and (b) the corresponding degradation percentages of Bsat by SHE at TA = 220, 300, 400 K and Vds = 0.7 V.
Fig. 12 compares the υinj and Qtop at ToB with different Vgs with and without SHE at TA = 220, 300, 400 K and Vds = 0.7 V. It shows that comparing with υinj, Qtop is less affected by TA and the difference becomes smaller with the increasing Vgs. SHE can lead to a prominent degradation in υinj and the degradation is much larger at a larger TA at the same Vgs. Therefore, the degradation in current is mainly caused by the degradation in υinj, which can explain the results in Fig. 10(b).

class="figure_img" id="Figure10"/>
Download
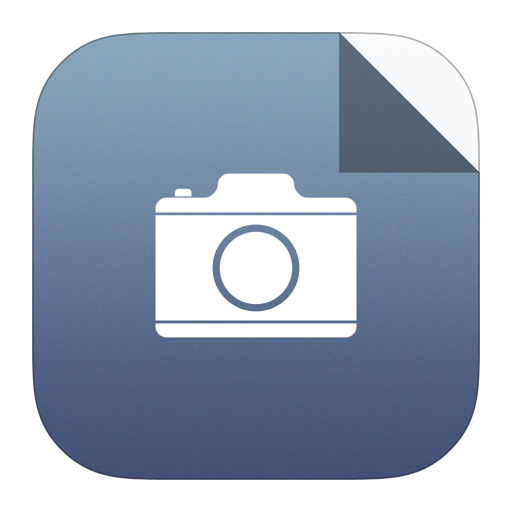
Larger image
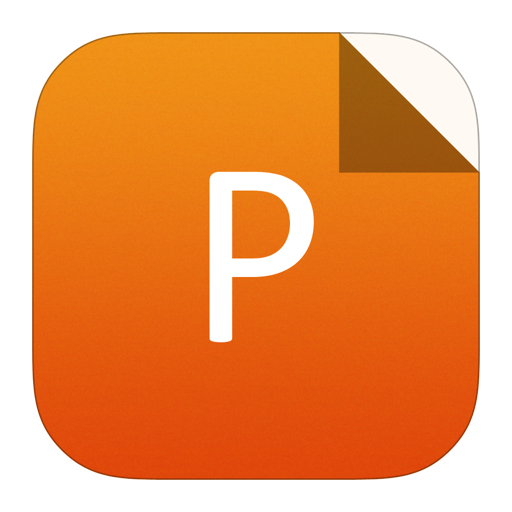
PowerPoint slide
Figure10.
(Color online) (a) Comparison of current densities considering SHE with those not considering SHE under different Vgs and (b) the corresponding current degradation percentage by SHE at TA = 220, 300, 400 K and Vds = 0.7 V.

class="figure_img" id="Figure12"/>
Download
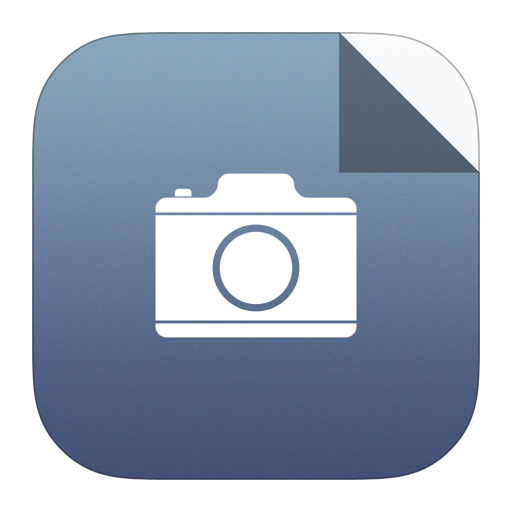
Larger image
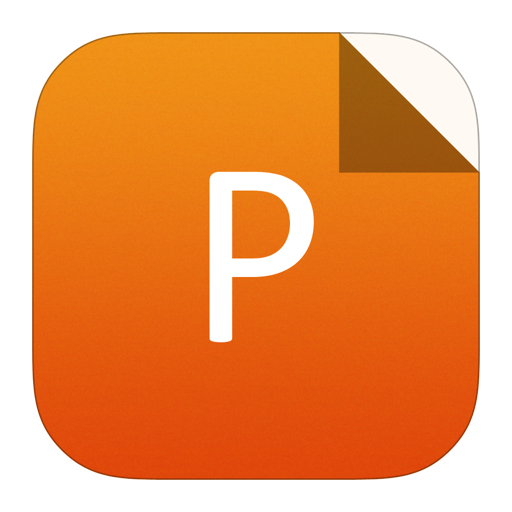
PowerPoint slide
Figure12.
(Color online) (a) Injection velocity υinj and (b) sheet density Qtop at the top of barrier ToB[45] under different Vgs with and without SHE at TA = 220, 300, 400 K and Vds = 0.7 V.
The relationship of T–TA with Qinput is shown in Fig 13(a). Results at TA = 220, 300, and 400 K when Vds = 0.7 V are presented. Tpeak–TA and Taverage–TA increase with increasing of Qinput. At the same Qinput, we can see that Tpeak–TA and Taverage–TA increase with the increase of TA. When Qinput = 90 μW at TA = 300 K, Tpeak–TA is 100 K and Taverage–TA is 75 K. The relationship of the device thermal resistance (Rth) with Qinput is shown in Fig 13(b). We can see that Rth decreases with the growth of Qinput. Besides, a larger Rth is found at a larger TA when considering the same Qinput. The probability of electron-phonon scattering is much larger at a larger TA. Electron-phonon scattering are an inelastic scattering and by experiencing this scattering, particles will exchange their energy to the lattice. A larger TA will lead to a larger part of Qinput turning into Qheat and an increasing Tpeak–TA. Therefore, the Rth increases with TA increases. The difference in Rth at different TA becomes smaller with the growth of Qinput, which is the result of the undistinguished heat density distribution shown in Fig. 5(a). To characterize SHE in nanoscale devices, the modeling on Rth at different TA is necessary in the device SHE investigation.

class="figure_img" id="Figure13"/>
Download
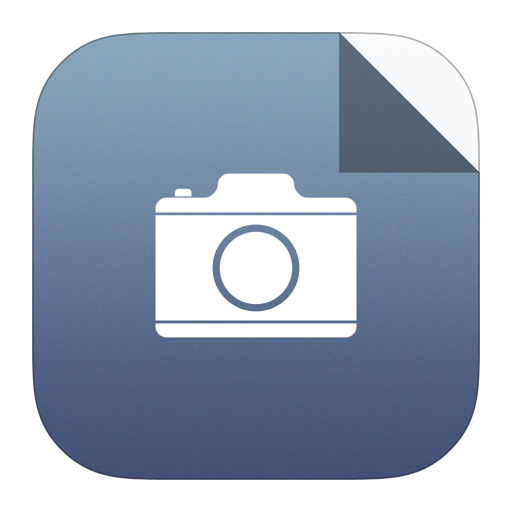
Larger image
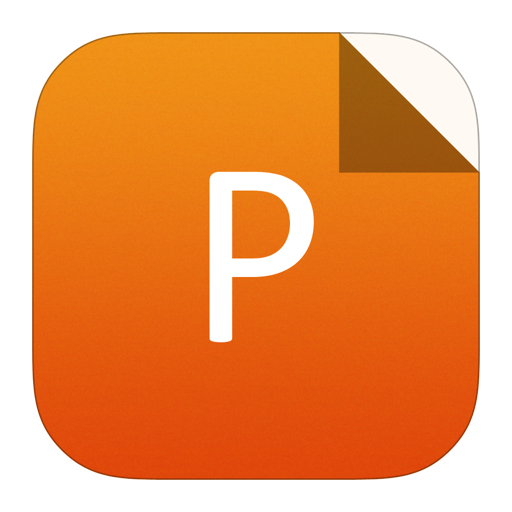
PowerPoint slide
Figure13.
(Color online) The relationship of (a) T–TA and (b) thermal resistance Rth with Qinput at TA = 220, 300, 400 K when Vds = 0.7 V. Tpeak and Taverage respectively refer to the peak lattice temperature and the average lattice temperature.
Fig. 14 shows the average augment of lattice temperature at the gate oxide region (Tgate–TA). Tpeak–TA is plotted for comparison. Results with TA = 220, 300, and 400 K under different Vgs at Vds = 0.7 V and different Vds at Vgs = 0.7 V are presented. In actual application, a temperature sensor is often placed at the gate top to achieve device temperature[46], which actually is Tgate. It shows that Tgate–TA increases with the increasing of TA. We can see that TA will have a serious impact on the results achieved by the temperature sensor. Therefore, the impact of TA should not be ignored.

class="figure_img" id="Figure14"/>
Download
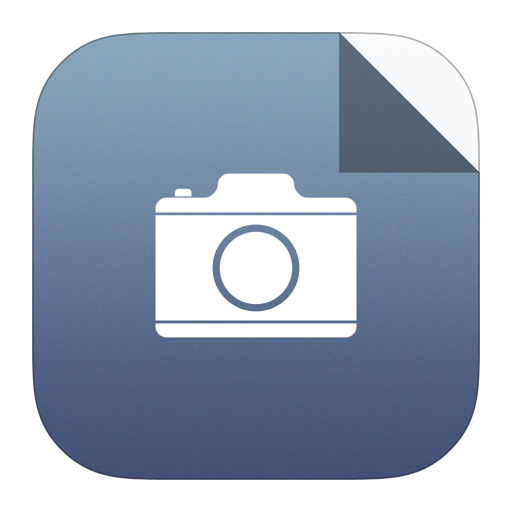
Larger image
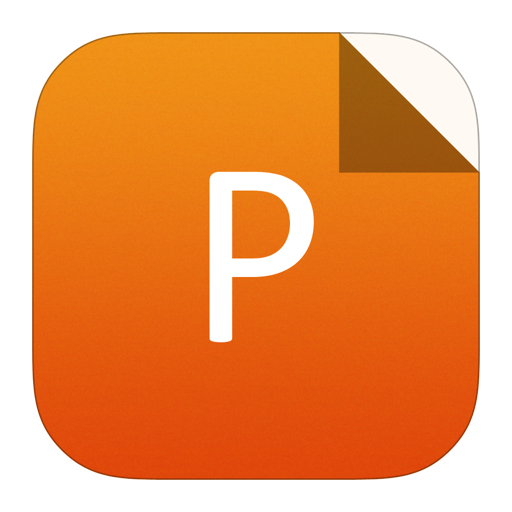
PowerPoint slide
Figure14.
(Color online) The average augment of lattice temperature at the gate oxide region (Tgate–TA) with TA = 220, 300 and 400 K (a) under different Vgs at Vds = 0.7 V and (b) under different Vds at Vgs = 0.7 V. Tpeak–TA is plotted for comparison.
4.
Conclusion
The impacts of TA on SHE in 14 nm-node nFinFETs are investigated in this work. Our electro-thermal coupled Monte Carlo simulation framework is useful to achieve the impact of the non-equilibrium transport and the non-local heat generation, which is important in analyzing SHE in nanoscale devices. The thermal non-equilibrium carriers carry out a part of Qinput, which eventually heats up the MOL and BEOL. An increase in Vgs can prominently increase heat generation in the channel and the drain side. However, an increase in Vds only results in an obvious heat generation increment in the drain side. The increase in TA can lead to more serious SHE, including a higher device temperature and larger degradation in current, ballistic factor and injection velocity, which are results of a larger heat generation in the device region. The increase in TA can lead to an increment of the device thermal resistance. The impact of TA should be carefully considered when investigating SHE in nanoscale devices.