1.
Introduction
Quantum cascade lasers (QCLs), as a unipolar semiconductor light source, were invented more than twenty years ago and are performing increasingly important roles in both middle-wave infrared (MWIR, λ = 3–5 μm) and long wave infrared (LWIR, λ = 8–12 μm)[1, 2]. Unlike traditional bipolar semiconductor laser devices, stimulated emission of QCLs is based on the intersubband transition of electrons in the conduction band[3, 4], and the internal quantum efficiency is furthermore increased by using a cascade effect. Due to their low electrical power consumption and miniature size, QCLs can be packaged into compact and portable systems, which have been widely applied[5]. The MWIR and LWIR QCLs are particularly attractive since a large number of molecular species have their “fingerprint” absorption in this spectrum range[6–8]. Watt-level of light power has been obtained in MWIR[9–11]. The development of LWIR QCLs has been lagging behind, however. The room temperature (RT) continuous wave (CW) operation of LWIR QCL can be obtained by few groups, especially when the wavelength is longer than 9 μm[12–14]. This is because there exists an intrinsic technological challenge in the LWIR, such as the increased absorption losses, the decreased optical confinement, the lower intersubband gain and so on[15–17]. All these factors contribute to gradually worsening device performances as the lasing wavelength moves toward the far-IR end of the LWIR window. One bit of good news from the QCLs in the LWIR is that the bandgap offset can be exploited for lattice-matched InGaAs/InAlAs/InP or a slight strain material system, which is propitious to the growth of ultrathin layer heterostructure material. In contrast, the QCLs in the MWIR need highly strained material growth to improve the electronic confinement.
In this letter, we present an LWIR QCL with a continuous wave (CW) operating temperature up to 303 K. The active region is designed with a double-phonon resonant structure and grown with strain-compensation technology. For a 2-mm-long and 10-μm-wide QCL with high-reflectivity (HR) coating on the rear facet, CW output power of 45 mW at 283 K and 9 mW at 303 K is obtained, at a lasing wavelength of ~ 9.4 μm.
2.
Wafer growth and devices fabrication
The QCL structure, designed according to double-phonon resonance theory, was grown on an n-doped (Si, 2 × 1017 cm?3) InP substrate wafer by solid-source molecular beam epitaxy (MBE). The active core structure presented in this paper is similar to Ref. [12], containing 30 periods of lattice-matched In0.6Ga0.4As/In0.44Al0.56As quantum wells and barriers. The specific layer sequence of one period, is as follows: (layer thickness in nanometers): 4.0/1.9/0.7/5.8/0.9/5.7/0.9/5.0/2.2/3.4/ 1.4/3.3/1.3/3.2/1.5/3.1/1.9/3.0/2.3/2.9/2.5/2.9, where InAlAs barrier layers are in bold, InGaAs well layers are in roman, and n-doped layers (2 × 1017 cm?3) are underlined. The complete structure of the QCL wafer layer sequence is: 4 μm lower InP cladding layer (Si, 3 × 1016 cm?3), 0.3-μm-thick n-In0.53Ga0.47As layer (Si, 4 × 1016 cm?3), 30 active/injector stages, 0.3-μm-thick n-In0.53Ga0.47As layer (Si, 4 × 1016 cm?3), 2.6 μm upper cladding layer (Si, 3 × 1016 cm?3), 0.15 μm gradually doped layer (changing from 1 × 1017 to 3 × 1017 cm?3) and 0.8 μm highly doped cladding layer (Si, 5 × 1018 cm?3).
The QCL wafer was then fabricated into a double channel ridge waveguide with an average active core width of 10 μm by conventional photolithography and a nonselective wet chemical etching. After etching, InP:Fe was grown by metal organic chemical vapor deposition (MOCVD) in the channel, which would decrease the waveguide loss and increase the heat dissipation of the devices. Then a layer of 450-nm-thick SiO2 was deposited as an electrical insulation by plasma enhanced chemical vapor deposition (PECVD). Then a Ti/Au layer as the top contact was deposited by e-beam evaporation, next a 5-μm-thick Au layer was electroplated to better conduct the current and heat on the laser surface. After the wafer was thinned down to 120 μm, the Ge/Au/Ni/Au metal contacts were formed as the substrate layer. Finally, the waveguide was then cleaved into 2-mm-long laser bars and the rear facets were coated with high reflection (HR) coating consisting of Al2O3/Ti/Au/Ti/Al2O3 (200/10/100/10/120 nm). The lasers were soldered epilayer side down onto diamond platelet, which was mounted on the copper heat sink with indium solder. Then the lasers were wire bonded and a thermistor was fixed on the heat sinks close to the laser chip to monitor the temperature.
The one-dimensional mode characteristic is studied for the LWIR QCL device with comsol, as shown in Fig. 1. The optical confinement factor of Г = 47% is calculated, which is defined as the ratio of the light confined in the InGaAs layer and active region, and the light in all the waveguide. The calculated effective refractive index neff = 3.16 + 1.11 × 10?4i, thus the waveguide loss αw = (4π/λ) × 1.11 × 10?4i is 1.48 cm?1, which is higher than the MWIR devices[18]. The reflection coefficient R1 of the front facet is around 27%, which is estimated with Fresnel relation R = (neff ? 1)2/(neff + 1)2, and the rear facet R2 is around 97% with HR coating. The mirror loss αm = 2.23 cm?1 is calculated with the formula αm = (1/2L)ln (1/R1R2).
3.
Results and discussion
The heat sink was then fixed on a metal sheet of which the temperature was monitored and adjusted by a thermistor and a thermoelectric cooler (TEC). The temperature of the heat sink is controlled very close to the metal sheet under CW operation at 283–303 K. The optical power from the uncoated facet of the laser was measured with a calibrated thermopile detector placed directly in front of the laser facet. The laser spectra were measured with a Fourier transform infrared (FTIR) spectrometer in rapid scan mode with a resolution of 0.25 cm?1.
Fig. 2(a) shows the optical power–current–voltage (PIV) curve of a 10-μm-wide and 2-mm-long coated HR on the rear facet, operating at different heat sink temperatures from 283 to 303 K. The power is up to 45 mW at 283 K with a threshold current density of 4 kA/cm2 and decreases to 9 mW when the temperature increases to 303 K. Fig. 2(b) shows that the pulsed peak output power of 100 mW is obtained at 283 K corresponding to 70 mW at 303 K with a repetition frequency of 5 kHz and a duty circle of 1%. The slope efficiency of the device at pulsed mode is 260 mW/A at 293 K. In this view, the device with more stages exhibiting the lower threshold is suitable for high temperature CW operation. The slope efficiency of the device can be described as

class="figure_img" id="Figure2"/>
Download
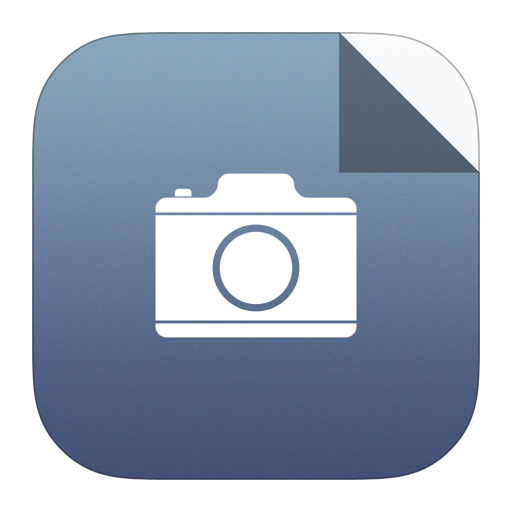
Larger image
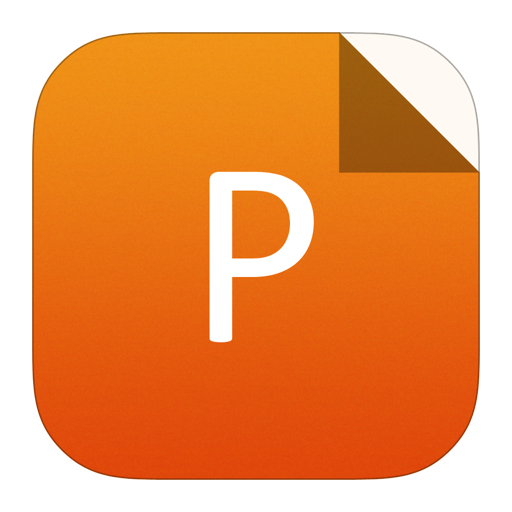
PowerPoint slide
Figure2.
(Color online) (a) Output light power versus injection current of a 2-mm-long and 10-μm-wide QCL in CW operation mode at different heat sink temperatures between 283 and 303 K along with V–I curves at 293 K. (b) Peak power of the same device changes with the injection current at the repetition frequency of 5 kHz and a duty circle of 1%.
$$frac{{{ m{d}}P}}{{{ m d}I}} = frac{{hnu }}{e}{N_{ m p}}frac{{{alpha _{ m m}}}}{{{alpha _{ m w}} + {alpha _{ m m}}}}{eta _{ m i}},$$ ![]() |
where hν is the photon energy, e is the elemental electronic charge, Np is the cascade period number, and ηi is the internal quantum efficiency of each period. Using the parameters obtained from Fig. 1, the internal quantum efficiency is around 11% per cascade period at 293 K. The internal quantum efficiency is lower than the MWIR for the reasons mentioned in the introduction[18].

class="figure_img" id="Figure1"/>
Download
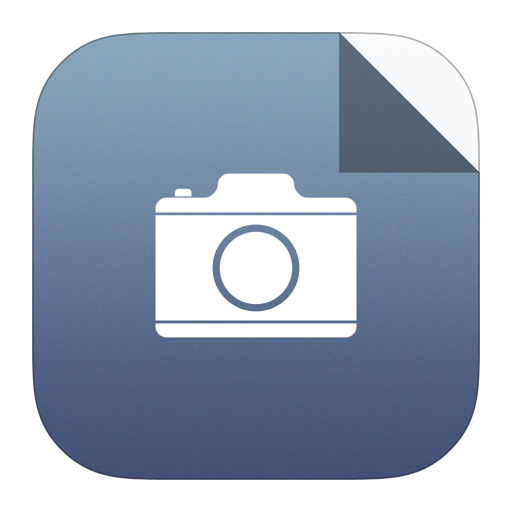
Larger image
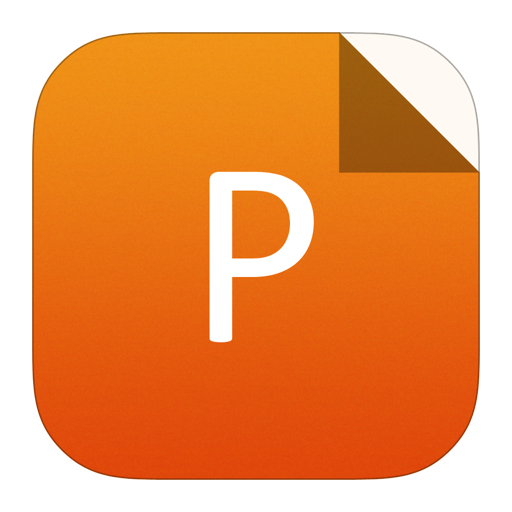
PowerPoint slide
Figure1.
(Color online) Mode characteristic according to the real part of the refractive index along the growth direction of the device structure with a Drude–Lorentz solver.
The spectrum characteristic of LWIR QCL is shown in Fig. 3. The emission frequency ν of a QCL can be tuned over a small range by changing the current and temperature. Fig. 3(a) shows the normalized CW lasing spectra at a current of 1.02 Ith with temperature ranging from 283 to 303 K with a step of 5 K of the device. The single lasing mode frequency changes from 1070.9 cm?1 at 283 K to 1058.7 cm?1 at 303 K. Fig. 3(b) demonstrates the CW lasing spectra of the same device at different injection currents, from 0.85 to 1 A, with a step of 0.03 A at 288 K, the single mode frequency changes from 1066.3 to 1065.0 cm?1 corresponding to the electrical power tuning coefficient ?ν/?P of ?0.537 cm?1W?1. The characteristic of a single mode is obvious in spite of no grating modulating the optical mode. This can be explained by the narrow electrical luminescence spectrum shown in the insert of Fig. 3(a). Besides, we have tried the external-cavity experiment with the device, which only has a narrow tunable range around 40 cm?1.

class="figure_img" id="Figure3"/>
Download
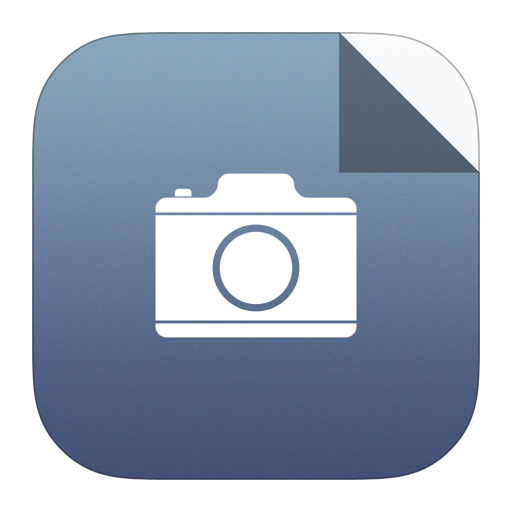
Larger image
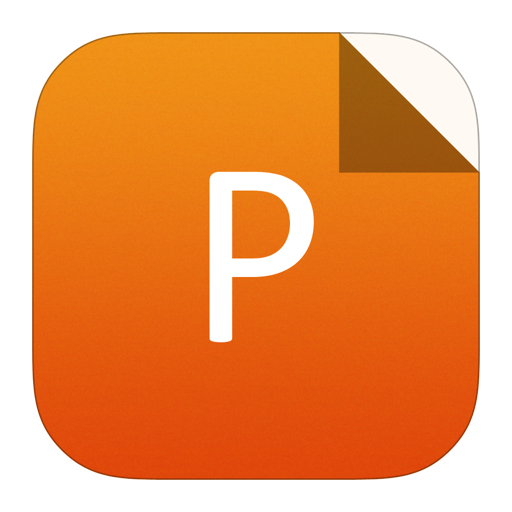
PowerPoint slide
Figure3.
(Color online) (a) CW emitting spectrum of QCL at different heat sink temperature between 283 and 303 K. The insert shows the electrical luminescence spectrum. (b) CW lasing spectra at a different injection current from 0.85 to 1.0 A with a step of 0.03 A. The inset shows the linear-fit tuning characteristics of the lasing frequency with electrical power for the same device.
In order to investigate the thermal behavior, we measured the threshold current characteristic at the different heatsink temperatures in CW and pulsed mode shown in Fig. 4. The red line fits with the exponential function Jth = J0exp(T/T0), where Jth is the threshold current density, J0 is the constant and T0 is the characteristic temperature[18]. The T0 is 238 K for the pulsed mode. Generally, due to the low heat conductivity of InGaAs/InAlAs ultrathin layer, the heat dissipation for the active region in CW operation mode is poor, thus the core temperature Tact of the QCL is much higher than the heatsink temperature Tsink. As a result, the threshold current increases more rapidly with a higher value in CW mode than in pulsed mode as the temperature is increased. From 283 to 303 K, T0 decreases to 128 K for CW mode. For high temperature and high power CW operation, the lower threshold power density and weaker temperature dependence are required.

class="figure_img" id="Figure4"/>
Download
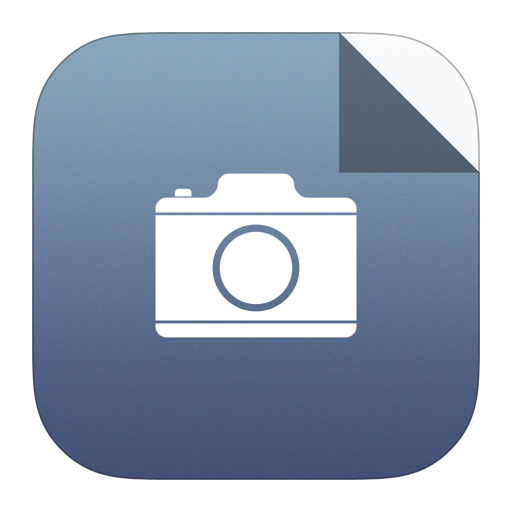
Larger image
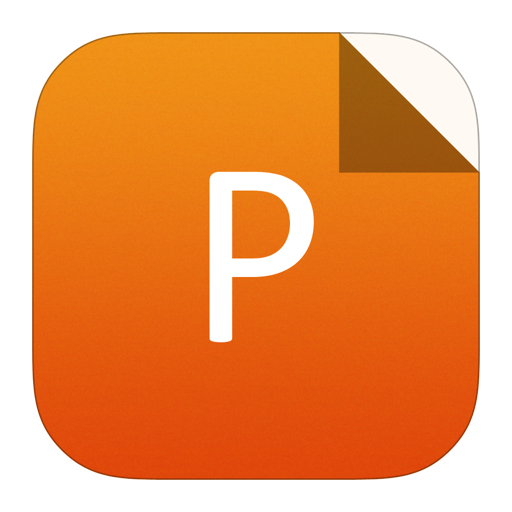
PowerPoint slide
Figure4.
(Color online) Threshold current density as a function of heat sink temperature in CW and pulsed mode of a 2-mm-long and 10-μm-wide LWIR QCL. The red line is fitted with the exponential function Jth = J0exp(T/T0).
The far-field measurement was done by mounting the laser on a computer controlled rotational stage with a step resolution of 0.05°. A room temperature operation HgCdTe detector was located 35 cm away from the QCL to collect the lasing light. Fig. 5 shows the measured lateral far-field radiation patterns of the LWIR QCL with the black dash and the fitted result of Gauss function with the red line. The measured full width at half maximum (FWHM) of the far-field pattern is 30°, which can be explained by the diffraction limit formula sinθ = 1.22λ/D, where θ is the diffraction angle, λ is the lasing wavelength and D is the width of the waveguide. The insert shows a spot of a laser beam emitted from the device collimated by an aspheric lens. The image was taken by a calibrated thermopile Infrared camera (Pyrocam III), indicating that the lasing mode occurs in fundamental mode both at the fast axis and slow axis direction.

class="figure_img" id="Figure5"/>
Download
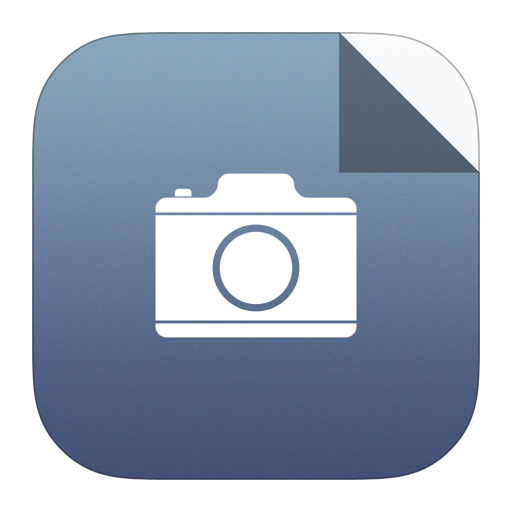
Larger image
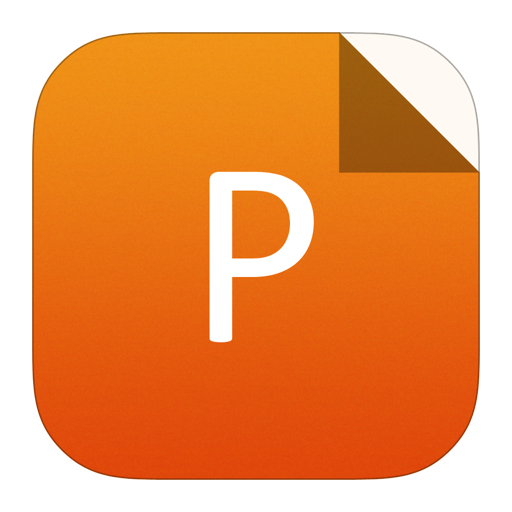
PowerPoint slide
Figure5.
(Color online) Measured lateral far-field radiation patterns for the devices at pulsed driving currents of 0.85 A under 50 kHz repetition frequency and 1% duty circle, the dash is the measurement and the red line is the result fitted with the Gauss function. The inset shows the spot of a laser beam emitted from the device collimated by the aspheric lens.
4.
Conclusion
In conclusion, we have demonstrated the CW operation of LWIR QCLs with a wavelength around 9.4 μm. The devices were fabricated as buried heterostructure lasers with semi-insulated InP:Fe to improve the heat dissipation capacity and decrease waveguide loss. The CW operating temperature is up to 303 K, which shows a good potential for practical applications.
Acknowledgements
The authors would like to acknowledge Ping Liang and Ying Hu for their help with device fabrication.