1.
Introduction
Body surface temperature can reflect the health status of the human body[1]. There are many devices for measuring human body temperature: e.g. the mercury thermometer, thermocouple thermometer, and infrared thermal imager. The first two devices yield more accurate temperature measurements within 0.05 °C[2], but their measurement efficiency is far too low. The latter device has high measurement efficiency, but the measurement accuracy cannot meet the requirements of practical applications. Studies have shown that measurements by an infrared thermal imager from the inner canthus of the eye correlates highly with axillary thermometry[3] and can be considered as an estimator of body core temperature[4]. If the measurement accuracy could be sufficiently improved, the infrared thermal imager would have very broad application prospects in many fields, such as inspection and quarantine in airports, population thermometry, and the real-time monitoring of the body temperatures of farm animals. Toward this goal, this paper aims to find a successful approach for reducing the error in measuring the temperature of human eyes with infrared thermal imaging, focusing on the influence of environment temperature and measurement distance.
In recent decades, most researchers have studied the uncooled infrared thermal imager[5, 6]. Owing to its high performance, contact-free testing, nondestructive examination, wide measurement range, low power consumption, high resolution, quick response, and low-cost[7], it has been widely used in military[8, 9] and civil applications[10], including electrical engineering[11], medicine[12, 13], construction[14], manufacturing[15], and road traffic flow monitoring[16]. The uncooled infrared thermal imager has progressed to a point where its sensitivity and resolution are sufficient for it to replace the cooled infrared thermal imager in civil applications. However, its measurement accuracy is affected by many factors, such as the emissivity of the tested object, the temperature of the environment, the distance between the tested object and the imager, the temperature of the atmosphere, and the transmissivity of the atmosphere. Therefore, the measured temperature tends to deviate from the actual temperature. This deviation is most sensitive to measurement distance and environment temperature.
Various methods have been developed to improve the accuracy of the uncooled infrared thermal imager. Zhang et al.[17] analyzed the effect of the internal temperature of the thermal imager on the measurement accuracy of the uncooled infrared thermal imager. They proposed a compensation method based on voltage compensation to acquire the actual surface temperature of objects. Their experiment showed that this method can effectively reduce the relative error of the infrared thermal imager temperature measurement in the laboratory environment. Chrzanowski[18] analyzed the effect of the difference between the object-to-imager distances under calibration and operating conditions on the accuracy of the temperature measurement with the infrared system. He developed several formulas for determining the temperature measurement errors due to this difference for any measurement conditions and system parameters. However, these papers are limited in scope to laboratory conditions, not in situ applications, particularly not the field of human body thermometry. In this paper, eye temperatures are measured to represent human body temperature practically with the goal of improving the measurement accuracy. Empirical error correction formulas are derived and verified experimentally.
2.
Theoretical principles
This section describes how measurement distance and environment temperature affect the measurement accuracy of the uncooled infrared thermal imager.
2.1
Influence of measurement distance on measurement accuracy
Fig. 1 contains a schematic representation of the tested object and imager (detector and optical system). The detector output voltage V(TO) can be expressed as[19]
$$Vleft( {{T_{ m O}}} ight){ m{ = }}, varepsilon {R_{max }}{A_{ m d}}{c_1}{sin ^2}{u_{ m m}}int_{{lambda _1}}^{{lambda _2}} {frac{{{tau _lambda }{s_lambda }}}{{{lambda ^5}left( {{{ m e}^{{{{c_2}} / {lambda {T_{ m o}}}}}} - 1} ight)}}} ,$$ ![]() | (1) |
where ε is the object's emissivity, Rmax is the maximum sensitivity of the uncooled infrared thermal imager, Ad is the area of the detector, um is the aperture angle of the image, λ1 and λ2 are the working band limits of the thermal imager, c1 is the first radiation constant, c2 is the second radiation constant, τλ is the optical system’s transmittance, sλ is the relative sensitivity of the detector, and TO is the absolute temperature of the test object's surface.
When the angle field of the detector is ω, the output voltage V′(TO) becomes
$$V'!!left( {{T_{ m O}}} ight){ m{ = }},,varepsilon {R_{max }}{A_{ m d}}{c_1}cos^4omega {sin ^2}{u_{ m m}}int_{{lambda _1}}^{{lambda _2}} {frac{{{tau _lambda }{s_lambda }}}{{{lambda ^5}left( {{{ m e}^{{{{c_2}} / {lambda {T_{ m o}}}}}} - 1} ight)}}}.$$ ![]() | (2) |
The aperture angle of the image can be defined by the geometry of the imager:
$${sin ^2}{u_{ m m}} = frac{{{{left( {{D / 2}} ight)}^2}}}{{{{left( {{D / 2}} ight)}^2} + {{d'}^2}}} = frac{{{D^2}}}{{{D^2} + 4{{d'}^2}}}.$$ ![]() | (3) |
According to Fig. 1, the relationship between d and d′ is

class="figure_img" id="Figure1"/>
Download
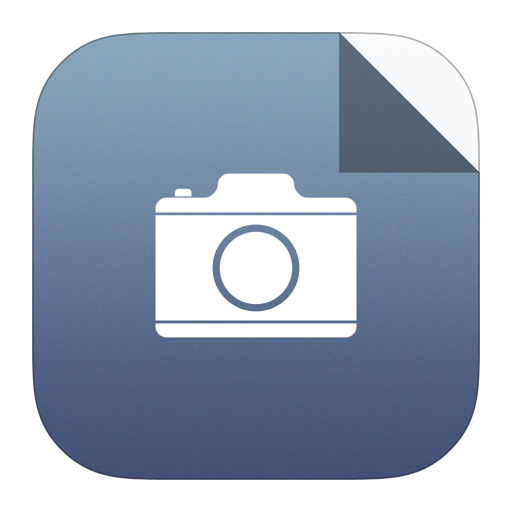
Larger image
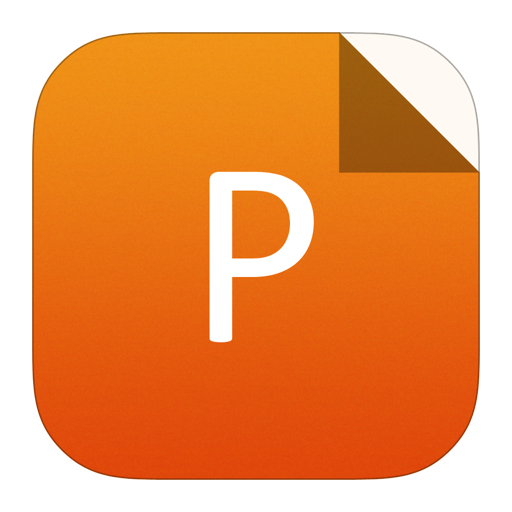
PowerPoint slide
Figure1.
Tested object imager in optical system.
$$d' = f' + frac{{{{f'}^2}}}{{f + d}},$$ ![]() | (4) |
where D is the pupil diameter of the optical system, d' is the imaging distance, d is the distance between the object and the infrared thermal imager, f is the object focus, and f' is the image focus. Thus, changing the measurement distance, d, will change the angle field. The output voltage will also change under the same conditions. Therefore, the measurement distance can affect the accuracy of the temperature measurement.
2.2
Influence of environment temperature on measurement accuracy
The absolute temperature of the tested object is measured by the radiation incident on the infrared detector. In general, the infrared thermal imager receives radiation from the tested object, reflected background, and atmosphere. The infrared detector transforms the radiation power into corresponding voltage signals[14]:
$${V_{ m t}} = {tau _alpha }left[ {varepsilon Vleft( {{T_{ m O}}} ight) + (1 - varepsilon )Vleft( {{T_{ m U}}} ight)} ight] + left( {1 - {tau _alpha }} ight)Vleft( {{T_{ m a}}} ight),$$ ![]() | (5) |
where τα is the transmissivity of the atmosphere, ε is the emissivity of the object, TO is the absolute temperature of the tested objected, TU is the absolute environment temperature, and Ta is the atmospheric temperature. According to Planck’s law of radiation, Eq. (5) can be rewritten as
$${T^n} = {tau _alpha }left[ {varepsilon T_{ m O}^n + left( {1 - varepsilon } ight)T_{ m U}^n} ight] + left( {1 - {tau _alpha }} ight)T_{ m a}^n.$$ ![]() | (6) |
Thus, the absolute surface temperature of the tested objected is
$${T_{ m O}} = {left{ {frac{1}{varepsilon }left[ {frac{1}{{{tau _alpha }}}{T^n} - left( {1 - varepsilon } ight)T_{ m U}^n - left( {frac{1}{{{tau _alpha }}} - 1} ight)T_{ m a}^n} ight]} ight}^{frac{1}{n}}}.$$ ![]() | (7) |
Eq. (7) demonstrates that environment temperature and atmospheric temperature can affect the measured temperature of the tested object.
3.
Experiments
A FLIR A300 (shown in Fig. 2) was used as the experiment instrument. Its features and technical specifications are as follows:

class="figure_img" id="Figure2"/>
Download
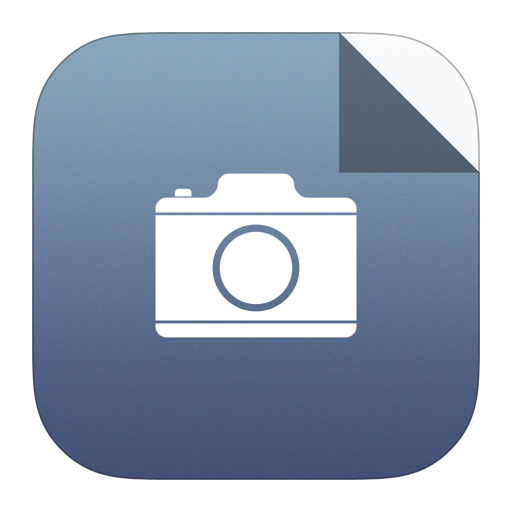
Larger image
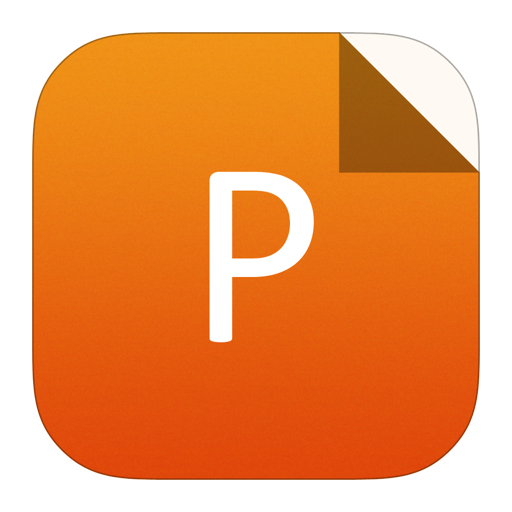
PowerPoint slide
Figure2.
(Color online) Picture of the infrared thermal imager.
● Detector type: uncooled infrared microbolometer FPA detector
● Resolution: 320 × 240
● Spectral range: 7.5 to 13 μm.
● Temperature measurement ranges: ?20 to 120 °C or 0 to 350°C
● Thermal sensitivity: < 0.05 °C @ + 30 °C
Fig. 3 shows that that the eyes have the highest temperature of the human face and can be considered as an estimator of body core temperature[4]. Therefore, we chose the eyes as the measurement point. We conducted numerous experiments to analyze the effects of the measurement distance and the environment temperature on measurement accuracy.

class="figure_img" id="Figure3"/>
Download
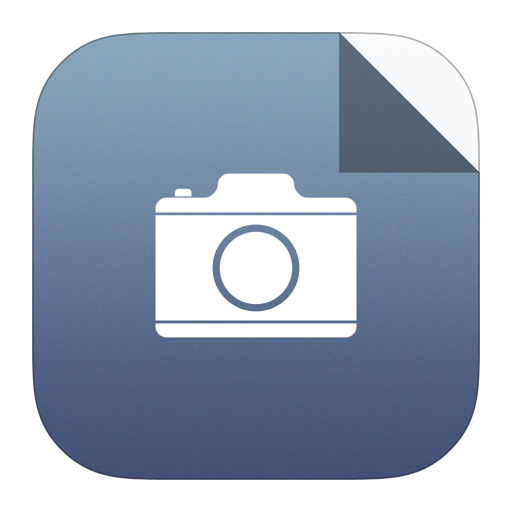
Larger image
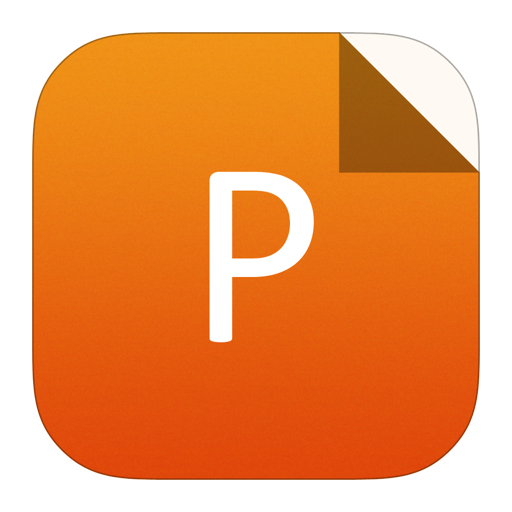
PowerPoint slide
Figure3.
Temperature distribution of the human face.
3.1
Relationship between human eye temperature and environment temperature
Owing to the inability to measure human eye temperature with a thermocouple, we measured the temperature of the forehead as an intermediate variable and eventually correlated this with eye temperature. In order to find the true temperature of the forehead in real working conditions, experiments were conducted in different environment temperatures. First, the thermocouple was used to measure forehead temperature in the test room with indoor environments of 19, 20, 21, 22, 23, 24, and 25 °C. Before measuring the forehead temperature, the human participant remained in the test room for five to ten minutes to reduce the effect of the outdoor environment and metabolic rate changes from movement. The relationship between forehead temperature and environment temperature is shown in Fig. 4.

class="figure_img" id="Figure4"/>
Download
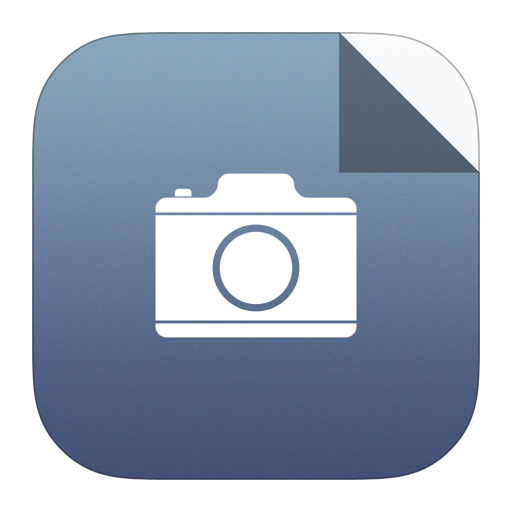
Larger image
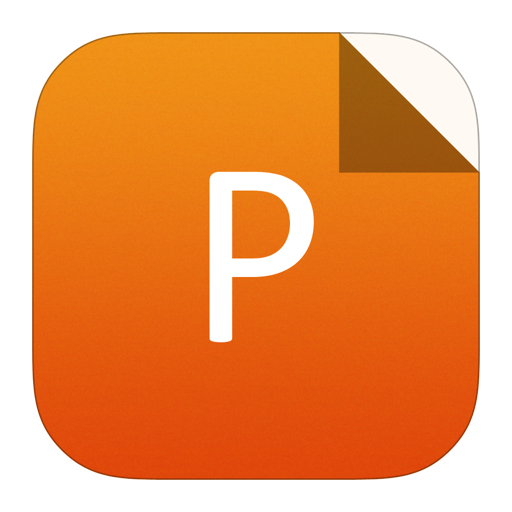
PowerPoint slide
Figure4.
(Color online) Relationship between human forehead temper-ature and environment temperature.
This result shows that the relationship between forehead temperature and environment temperature is approximately linear. To verify the assumption, a linear fit of the data was made. The regression equation is given by the following formula:
$${T_{ m F}} = 32.5164 + 0.1275{T_{ m U}},$$ ![]() | (8) |
where TF is forehead temperature and TU is environment temperature; the coefficient of determination, R2, is 0.97995. Fig. 5 compares the forehead and eye temperatures measured by the uncooled infrared thermal imager. These results indicate that eye temperature is averagely 1 °C higher than forehead temperature. Thus, eye temperature can be expressed approximately as

class="figure_img" id="Figure5"/>
Download
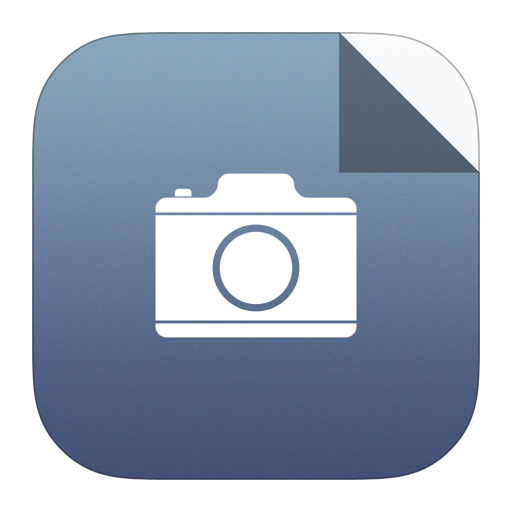
Larger image
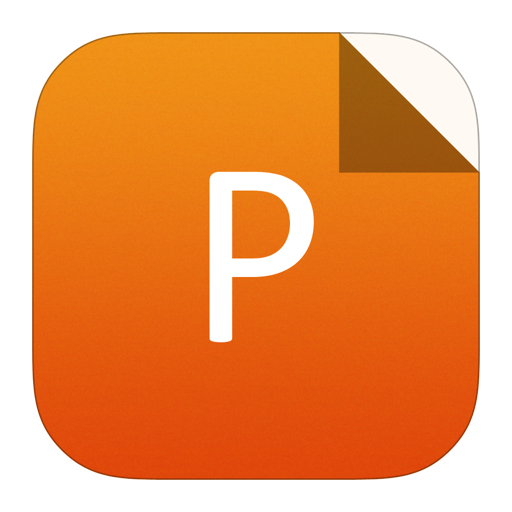
PowerPoint slide
Figure5.
(Color online) Forehead and eye temperatures measured by the uncooled infrared thermal imager.
$${T_{ m E}} = {T_{ m F}} + 1,$$ ![]() | (9) |
where TE is the actual temperature of the eyes. Combining Eq. (8) with Eq. (9) yields a correlation between the measured eye temperature and the temperature of the environment:
$${T_{ m E}} = 33.5164 + 0.1275{T_{ m U}}.$$ ![]() | (10) |
3.2
Relationship between measured temperature and measurement distance
We measured human eye temperature at different measurement distances. The results prove that the relationship between measured temperature and measurement distance is approximately linear. Under the conditions of constant environment temperature and humidity and 100% atmospheric transmittance in the laboratory, several groups of infrared image data in different environment temperatures were obtained. The test matrix comprised environment temperatures of 22, 23, 24, 25, and 26 °C and measurement distances of 0.6, 1.2, 1.8, 2.4, 3.0, 3.6, 4.2, 4.8, 5.4, and 6.0 m. The data are shown in Table 1. Each data point was measured three times, and its average value is given. According to Table 1 and Fig. 6, the measured temperature decreases as the distance increases, and there exists a linear relationship between them at distances of 0–6 m.
T (°C) | d (m) | |||||||||
0.6 | 1.2 | 1.8 | 2.4 | 3.0 | 3.6 | 4.2 | 4.8 | 5.4 | 6.0 | |
22 | 34.95 | 34.89 | 34.75 | 34.71 | 34.56 | 34.36 | 34.27 | 34.17 | 34.15 | 33.94 |
23 | 35.35 | 35.23 | 35.00 | 34.85 | 34.72 | 34.66 | 34.56 | 34.46 | 34.30 | 34.16 |
24 | 35.64 | 35.56 | 35.41 | 35.21 | 35.11 | 34.97 | 34.86 | 34.74 | 34.60 | 34.63 |
25 | 35.86 | 35.79 | 35.69 | 35.54 | 35.46 | 35.21 | 35.05 | 35.05 | 34.84 | 34.74 |
26 | 36.27 | 36.27 | 35.99 | 35.93 | 35.92 | 35.74 | 35.60 | 35.61 | 35.52 | 35.44 |
Table1.
Measured eye temperature (°C) for various distances and environment temperatures.
Table options
-->
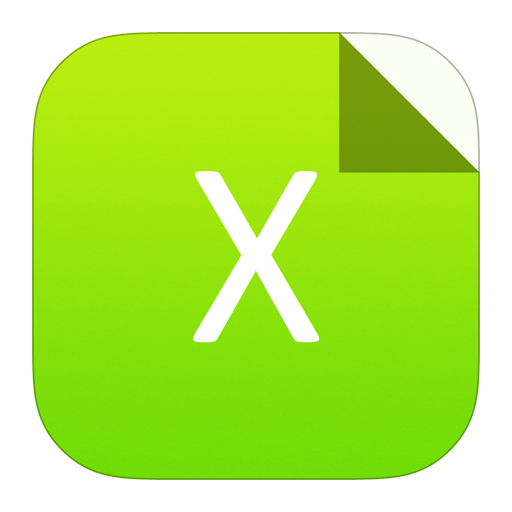
Download as CSV
T (°C) | d (m) | |||||||||
0.6 | 1.2 | 1.8 | 2.4 | 3.0 | 3.6 | 4.2 | 4.8 | 5.4 | 6.0 | |
22 | 34.95 | 34.89 | 34.75 | 34.71 | 34.56 | 34.36 | 34.27 | 34.17 | 34.15 | 33.94 |
23 | 35.35 | 35.23 | 35.00 | 34.85 | 34.72 | 34.66 | 34.56 | 34.46 | 34.30 | 34.16 |
24 | 35.64 | 35.56 | 35.41 | 35.21 | 35.11 | 34.97 | 34.86 | 34.74 | 34.60 | 34.63 |
25 | 35.86 | 35.79 | 35.69 | 35.54 | 35.46 | 35.21 | 35.05 | 35.05 | 34.84 | 34.74 |
26 | 36.27 | 36.27 | 35.99 | 35.93 | 35.92 | 35.74 | 35.60 | 35.61 | 35.52 | 35.44 |

class="figure_img" id="Figure6"/>
Download
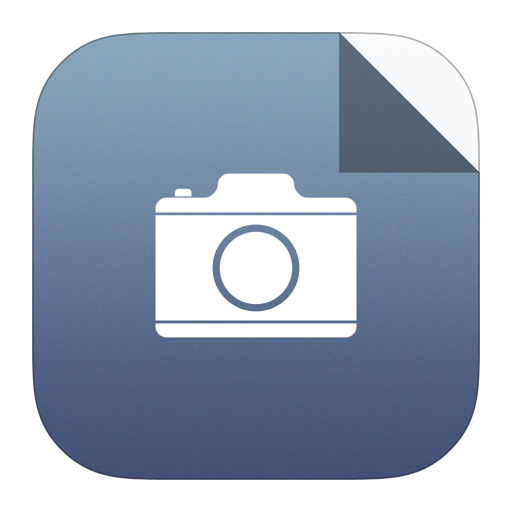
Larger image
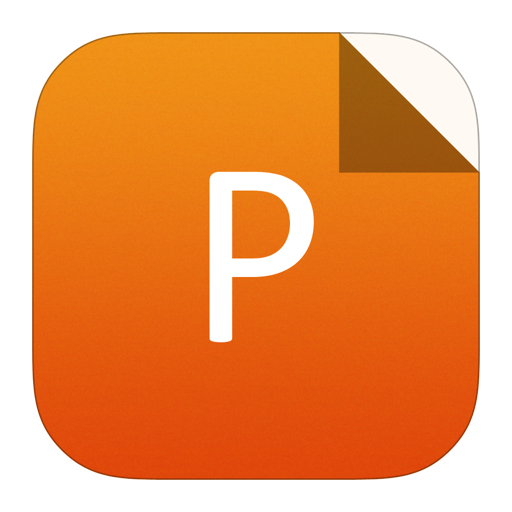
PowerPoint slide
Figure6.
(Color online) Measured temperature for various distances and environment temperatures.
According to Table 2, all the coefficients of determination are greater than 0.95, which shows a high degree of fit to the regression equation. Therefore, the model relationship between the temperature measured by an uncooled infrared thermal imager and the measurement distance can be written as
Temperature (°C) | Regression equation* | R2 |
22 | TM = ?0.188dd + 35.0928 | 0.98393 |
23 | TM = ?0.2092d + 35.4214 | 0.98364 |
24 | TM = ?0.2064d + 35.7544 | 0.98129 |
25 | TM = ?0.2191d + 36.0451 | 0.98489 |
26 | TM = ?0.1597d + 36.3556 | 0.95921 |
*: TM is the measured temperature and d is the measurement distance. |
Table2.
Regression equations of measured eye temperature with measurement distance for various environment temperatures.
Table options
-->
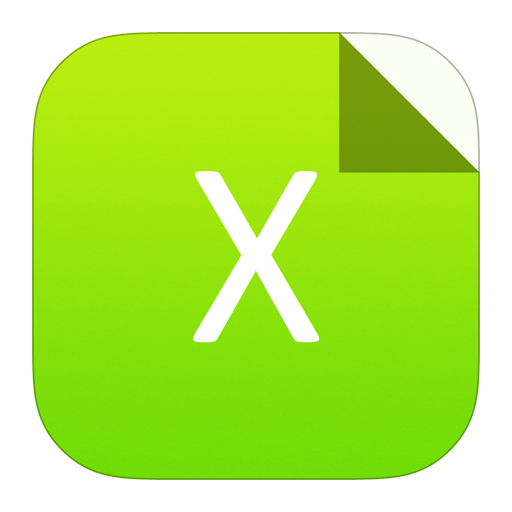
Download as CSV
Temperature (°C) | Regression equation* | R2 |
22 | TM = ?0.188dd + 35.0928 | 0.98393 |
23 | TM = ?0.2092d + 35.4214 | 0.98364 |
24 | TM = ?0.2064d + 35.7544 | 0.98129 |
25 | TM = ?0.2191d + 36.0451 | 0.98489 |
26 | TM = ?0.1597d + 36.3556 | 0.95921 |
*: TM is the measured temperature and d is the measurement distance. |
$${T_{ m M}} = {C_1} + {C_2}d,$$ ![]() | (11) |
where C1 and C2 are fitting coefficients.
3.3
Relationship between measured temperature and environment temperature
The relationship between measured temperature and environment temperature was analyzed using the methodology used in the prior section. Using the data from Table 1, the regression equations of measured temperature and environment temperature for various distances can be obtained. The results indicate that there is a linear relationship between measured temperature and environment temperature. The relationships are shown in Fig. 7.

class="figure_img" id="Figure7"/>
Download
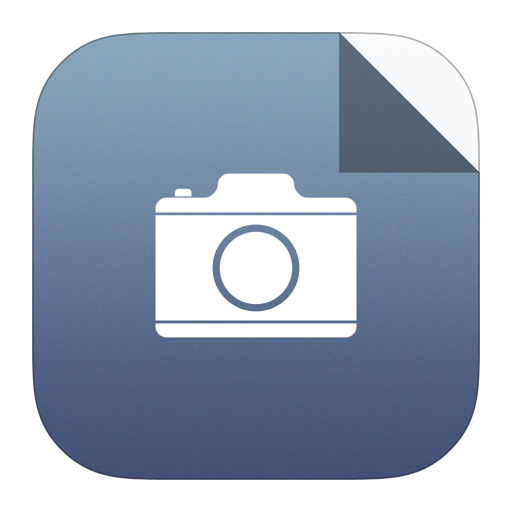
Larger image
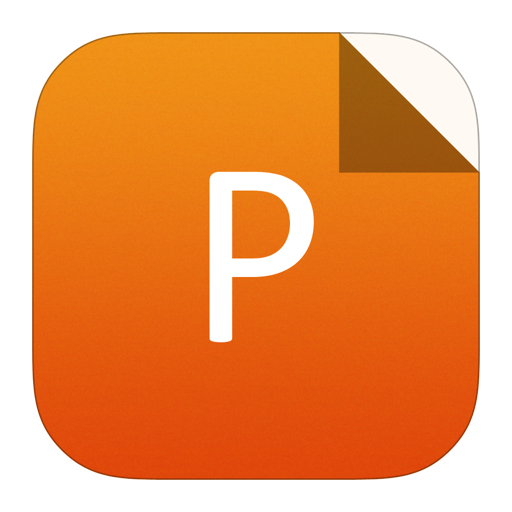
PowerPoint slide
Figure7.
(Color online) Fitting curves of measured temperature with environment temperature for various measurement distances.
The linear regressions in Table 3 have high coefficients of determination, indicating a high degree of fit. The relationship between measured temperature and environment temperature is linear, so the relationship between measured temperature and environment temperature can be established and written as:
Distance (m) | Regression equation* | R2 |
0.6 | TM = 0.315TU + 28.054 | 0.99015 |
1.2 | TM = 0.332TU + 27.58 | 0.98937 |
1.8 | TM = 0.317TU + 27.76 | 0.99526 |
2.4 | TM = 0.313TU + 27.736 | 0.98 |
3.0 | TM = 0.346TU + 26.85 | 0.97846 |
3.6 | TM = 0.331TU + 27.044 | 0.98078 |
4.2 | TM = 0.315TU + 27.308 | 0.97138 |
4.8 | TM = 0.347TU + 26.478 | 0.97535 |
5.4 | TM = 0.328TU + 26.81 | 0.92515 |
6.0 | TM = 0.358TU + 25.99 | 0.94678 |
*: TM is the measured temperature and TU is the environment temperature. |
Table3.
Regression equations of measured eye temperature with environment temperature for various measurement distances.
Table options
-->
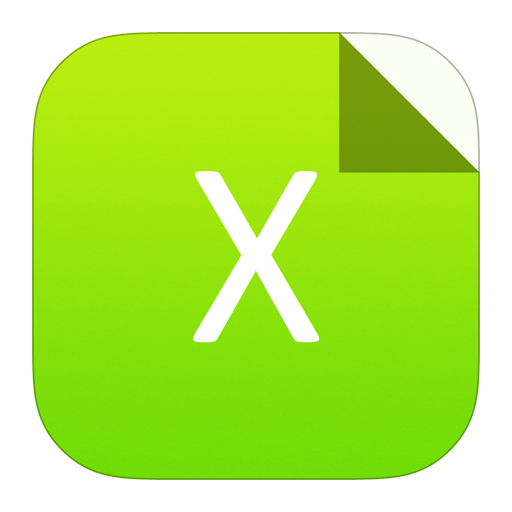
Download as CSV
Distance (m) | Regression equation* | R2 |
0.6 | TM = 0.315TU + 28.054 | 0.99015 |
1.2 | TM = 0.332TU + 27.58 | 0.98937 |
1.8 | TM = 0.317TU + 27.76 | 0.99526 |
2.4 | TM = 0.313TU + 27.736 | 0.98 |
3.0 | TM = 0.346TU + 26.85 | 0.97846 |
3.6 | TM = 0.331TU + 27.044 | 0.98078 |
4.2 | TM = 0.315TU + 27.308 | 0.97138 |
4.8 | TM = 0.347TU + 26.478 | 0.97535 |
5.4 | TM = 0.328TU + 26.81 | 0.92515 |
6.0 | TM = 0.358TU + 25.99 | 0.94678 |
*: TM is the measured temperature and TU is the environment temperature. |
$${T_{ m M}} = {C_2} + {C_3}{T_{ m U}},$$ ![]() | (12) |
where C2 and C3 are fitting coefficients.
3.4
Model of measurement error according to measurement distance and environment temperature
Sections 3.2 and 3.3 separately analyzed the effects of measurement distance and environment temperature on the measured temperature. Because both sets of relationships were linear, the combined effect of these factors can be formed with a bi-linear model:
$${T_{ m M}} = A + Bd + C{T_{ m U}},$$ ![]() | (13) |
where TM is the measured temperature, A, B, and C are fitting coefficients, d is the measurement distance, and TU is the environment temperature. The errors between the measurement temperature and the actual temperature can be written as:
$${T_{ m e}} = T - {T_{ m M}},$$ ![]() | (14) |
where Te is the temperature measurement error and T is the actual temperature. Combining Eq. (13) with Eq. (14), Te can also be written as:
$${T_{ m e}} = A + Bd + C{T_{ m U}},$$ ![]() | (15) |
but with different fitting coefficients.
The actual eye temperature can be obtained using Eq. (10). From the data of Table 1, the fitting coefficients can be obtained:
$${T_{ m e}} = 5.694 + 0.1966d - 0.2022{T_{ m U}}.$$ ![]() | (16) |
The calibrated formula for temperature is
$${T_{ m C}} = {T_{ m M}} + {T_{ m e}}.$$ ![]() | (17) |
Namely,
$${T_{ m C}} = {T_{ m M}} + 5.694 + 0.1966{d_{}} - 0.2022{T_{ m U}},$$ ![]() | (18) |
where TC is the temperature after error correction.
In general, there is no need to use an uncooled infrared thermal imager to measure human skin temperature at great distances, so, the atmospheric transmittance is considered to be 100% and other factors can be ignored. The most significant factors are measurement distance and environment temperature. By adjusting the measured temperature according to the measurement distance and environment temperature, we can obtain the actual temperature of human eyes.
3.5
Verification experiment
In order to verify the accuracy of the formula, an additional experiment was performed. Three groups of infrared image data were obtained at environment temperatures of 10, 18, and 25 °C. The curves of the measured temperature and corrected temperature are shown in Fig. 8.

class="figure_img" id="Figure8"/>
Download
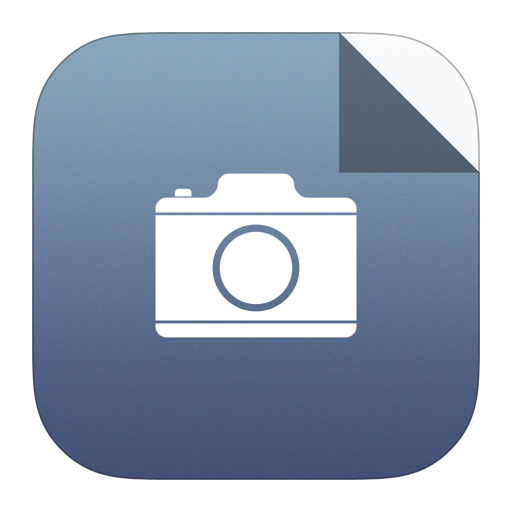
Larger image
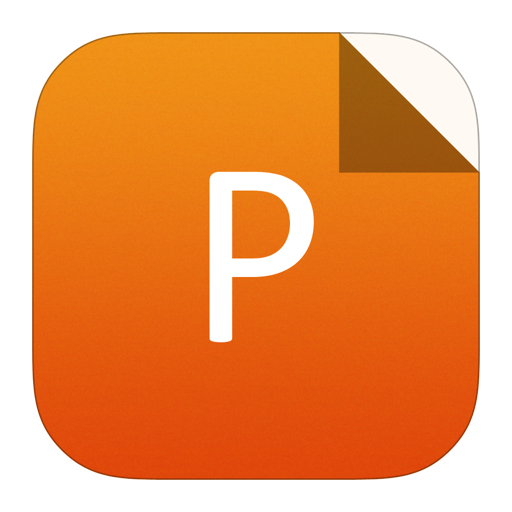
PowerPoint slide
Figure8.
(Color online) Curves of measured temperature, actual temperature, and corrected temperature in environment temperatures of (a) 10 °C, (b) 18 °C, and (c) 25 °C.
According to Fig. 8, there is a considerable error between the measured temperature and the actual temperature. The measurement error before correction is shown in Table 4. The measurement error after correction is shown in Table 5.
Temperature (°C) | 10 | 18 | 25 |
Max error (°C) | 5.59 | 3.21 | 1.90 |
Average error (°C) | 4.25 | 2.58 | 1.37 |
Max relative error (%) | 16 | 9 | 5.2 |
Table4.
Measurement error before correction.
Table options
-->
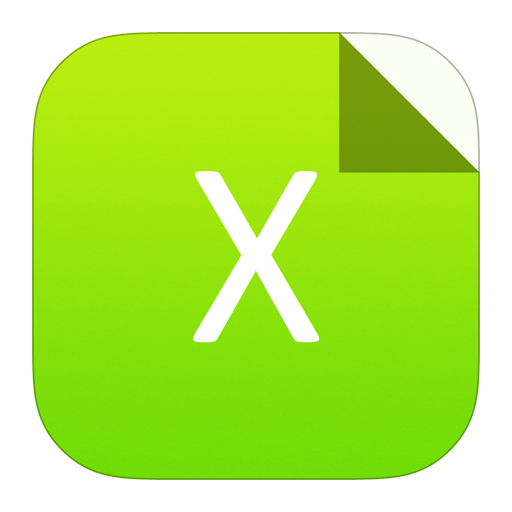
Download as CSV
Temperature (°C) | 10 | 18 | 25 |
Max error (°C) | 5.59 | 3.21 | 1.90 |
Average error (°C) | 4.25 | 2.58 | 1.37 |
Max relative error (%) | 16 | 9 | 5.2 |
Temperature (°C) | 10 | 18 | 25 |
Max error (°C) | 1.13 | 0.31 | 0.23 |
Average error (°C) | 0.6 | 0.14 | 0.09 |
Max relative error (%) | 3.2 | 0.8 | 0.62 |
Table5.
Measurement error after correction.
Table options
-->
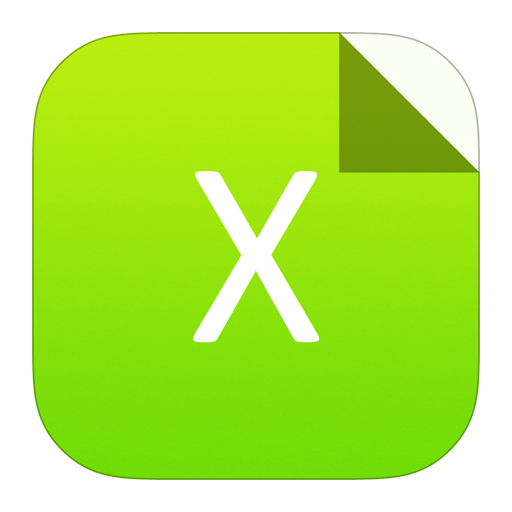
Download as CSV
Temperature (°C) | 10 | 18 | 25 |
Max error (°C) | 1.13 | 0.31 | 0.23 |
Average error (°C) | 0.6 | 0.14 | 0.09 |
Max relative error (%) | 3.2 | 0.8 | 0.62 |
Comparing Table 4 with Table 5 reveals that the maximum error decreased by 4.46 °C, the average error decreased by 3.65 °C, and the maximum relative error decreased by 12.8 percentage points at an environment temperature of 10 °C. At an environment temperature of 18 °C, the maximum error decreased by 2.9 °C, the average error decreased by 2.44 °C, and the maximum relative error decreased by 8.2 percentage points. Finally, at an environment temperature of 25 °C, the maximum error decreased by 1.67 °C, the average error decreased by 1.28 °C, and the maximum relative error decreased by 4.58 percentage points.
4.
Conclusion
In order to determine the effect of measurement distance and environment temperature on the temperature measured by an uncooled infrared thermal imager, several experiments were conducted. These experiments show that the measurement distance and environment temperature have strong linear effects on the measurement accuracy. We established a model based on the measured temperature, measurement distance, and environment temperature. The established model is an empirical model. We determine the effects of environment temperature and measurement distance on the measurement temperature of the human eye by experimental data. Finally, we obtained an empirical formula for calibrating the measured temperature to the actual temperature of the human eye. This formula was verified to be effective by experiment. Generally, this model can greatly improve the measurement accuracy under the conditions of known measurement distance and environment temperature.