1.
Introduction
Ammonia (NH3) is a noxious gas that can be lethal at high concentrations or long exposure times. It is both produced by natural processes such as decomposition of biological materials as well as by the manufacturing industry such as in e.g. industrial refrigeration[1]. Although the human nose can detect NH3 odor at a low non-hazardous level of 5–10 ppm[2], detectors are still needed for health and safety and also for medical diagnostics. Detectors in the industrial application domain do not need to be fast nor have ultrahigh sensitivity, the 8 h time-weighted average exposure limit is approximately 25 ppm. However, medical diagnostics such as the use of NH3 as a biomarker for liver and kidney disease[3] requires highly sensitive, selective and compact devices. Current research investigates non-invasive measurement techniques such as e.g. breath analysis for the detection of NH3. In this case, the sensor detection limit needs to go down to ~50 ppb. In all these cases interfering gases will be present including H2O, CO, NOx, etc. Sensors thus need selectivity in order to distinguish between the different gases within a mixture.
Different types of sensing methods exist, including electrochemical, electrical (e.g. resistive, capacitive, amperometric, etc), optical (e.g. surface plasma resonance) and mechanical (piezo-electric)[4, 5]. Solid-state electrical sensors come with the benefits of recovering to their original state due to the gas adsorption/desorption process; CMOS integration possibilities for readout and control; and compactness. However, their sensitivity to the background gases mentioned above is problematic. Approaches to improve the selectivity of the sensors are based on functionalization with selective layers, often consisting of nanoparticles[6]. Since the adsorption of gases on the sensing surface influences its character, another method to improve selectivity is to probe the electrical characteristics of the surface via low frequency noise measurements[7]. Since NH3 is an electron donor, its presence changes not only the electrical conductivity of the sensor but can also influence the low-frequency noise of the sensor via interaction with surface traps[8]. The combination of conductivity change and change in low-frequency noise characteristics can be exploited to increase the selectivity of the sensor and is also a tool to better understand the processes occurring at the surface of the sensors.
In order to increase the sensitivity of the resistive-based solid-state sensors, the effective surface area can be increased and/or the dimensions of the sensing channel can be reduced to increase the surface-to-volume ratio. One-dimensional structures are of particular interest for this purpose[9]. Their fabrication and operation are schematically illustrated in Table 1.
Nano-wires/-rods | Structure | Response time | Effective detection surface | Carrier transport mechanism | Fabrication |
Single | ![]() | Fast (~10 s) | Small | 1D | Complex |
Aligned array | ![]() | Slow (order of minutes) | Large | 1D | Complex/easy |
Random distribution | ![]() | Slow (order of minutes) | Large | Hopping | Easy |
Table1.
Different configurations of nanowire/rod based sensors with their particular characteristics[10].
Table options
-->
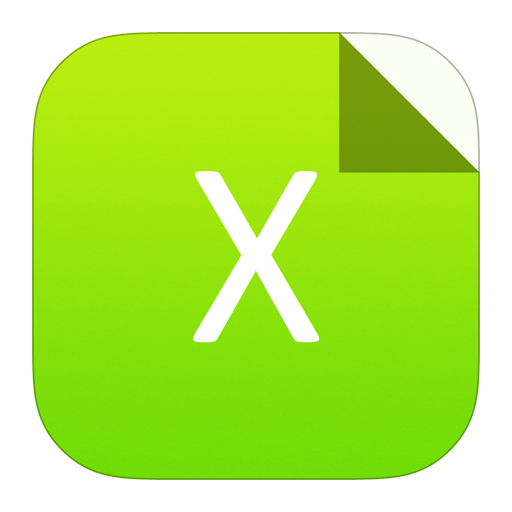
Download as CSV
Nano-wires/-rods | Structure | Response time | Effective detection surface | Carrier transport mechanism | Fabrication |
Single | ![]() | Fast (~10 s) | Small | 1D | Complex |
Aligned array | ![]() | Slow (order of minutes) | Large | 1D | Complex/easy |
Random distribution | ![]() | Slow (order of minutes) | Large | Hopping | Easy |
A wide range of top down and bottom up methods exist to fabricate 1D structures. Amongst the bottom up techniques low and high temperature CVD, MBE, laser ablation, and others can be found[11]. Characteristics of these approaches are versatility, good control over morphology, large material diversity, very small diameter, but also kinks in growth direction, difficulty to control doping and catalyst-related defects.
Lithography and etching can be used as top down techniques. A top down approach that does not require lithography is based on metal-assisted chemical etching (MACE) which is a wet chemical process in “ambient” conditions in which noble metal particles (Ag, Au, Pt, etc) on the surface act as catalyst for H+ generation to locally oxidise the semiconductor. For Si-based materials HF then dissolves the SiO2, generating directional pits[12, 13]. This gives a random array of nanowires with a diameter range of 50 nm < d < 300 nm and a length determined by the etch times and ambient conditions. Template based MACE increases the number of required process steps but leads to better control and uniformity of the nanowire diameter [14]. The main characteristics of the MACE process are its simplicity, low cost, easy process control and doping determined by the substrate. However, the etch is strongly doping concentration dependant, the material choice is limited and the process is wasteful in material use.
In this manuscript MACE will be used to fabricate Si nanowire arrays (Si NWAs) for the sensors. A SEM micrograph of a typical Si NWA used in this work is given in Fig. 1, showing a parallel connection of well-aligned nanowires attached to the Si substrate. Evaporation of a metal contact under an angle with respect to the nanowires, leaves top access for gas ad-/desorption.
In addition to a wide range of semiconducting nanostructures used for gas sensing, graphene has also become a popular material for sensing purposes, driven by its 2D character, chemical stability and high electrical conductivity[15]. In particular, CVD graphene has been studied widely because of its potential for upscaling to commercial applications[16]. As with other solid-state sensors, graphene is sensitive to multiple gases. Selectivity can be obtained between gases donating a different carrier type such as NH3 (electron donor) and NO2 (electron acceptor). However in many practical sensing applications H2O (in the form of relative humidity) will be present and influences the response of the sensors. It has been demonstrated that low-frequency noise characterization of graphene and its response to different gases might help improve selectivity without the need for surface functionalization. In Ref. [17] selectivity was obtained between solvents via the different shift of the lifetime of the generation-recombination center determined in the low-frequency noise characteristics. Although, graphene offers a large surface-to-volume ratio, only half of the surface is available for easy molecule adsorption as the graphene layer needs to be supported onto a substrate. In this manuscript we report on the combination of graphene and Si NWAs. Graphene is transferred onto the NWA making the bottom area of the graphene available for molecule adsorption. The combination of Si NWAs and graphene leads to higher improvements in sensitivity of the graphene layers than when graphene resides on a solid surface. A SEM micrograph of a graphene layer on a Si NWA is given in Fig. 2. The Si NWs in this figure are bundled at the top due to capillary effects in the wet chemical process and van der Waals forces.

class="figure_img" id="Figure2"/>
Download
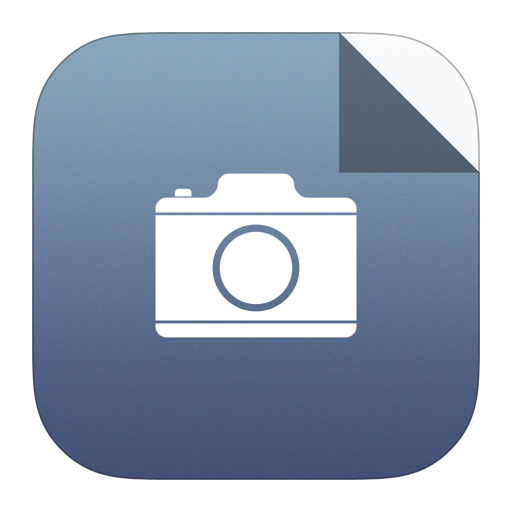
Larger image
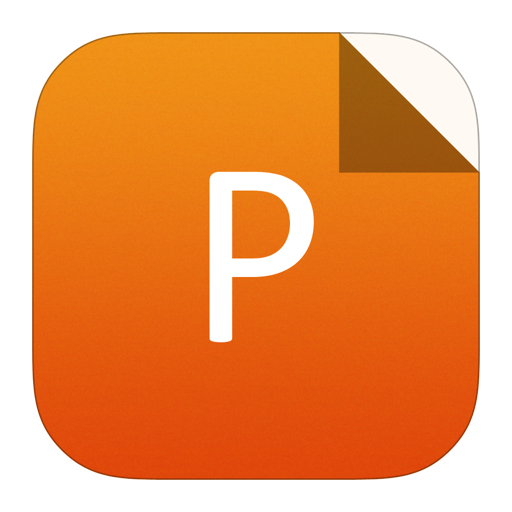
PowerPoint slide
Figure2.
SEM images. (a) Top view of a graphene layer covering 2/3 rd of the Si NWA in the image. Graphene can be seen as a milky shine on top of the Si NWA. (b) Side view of the suspended graphene layer that can be seen as a white sheet on top of the Si NWA.
In this manuscript we will qualitatively compare the resistive and electrical low-frequency noise response of Si NWAs and graphene supported by Si NWAs.
2.
Experiment
2.1
Sample preparation
The Si NWAs are fabricated using MACE on (100) substrates. For the Si NWA sensors, Si samples of ~1 × 1 cm2 with a resistivity, ρ = 1–10 Ω cm were used. Work is carried out on p- (acceptor doped using B atoms) as well as n-type (donor doped using As atoms) Si. One surface of the sample is protected with a poly methyl methacrylate (PMMA) layer during etching. After cleaning, the sample is immersed in a solution of 0.03 M AgNO3 : 5.6 M HF for 3 h. This one-step MACE process creates vertically aligned Si NWs attached to the remaining Si substrate (see Fig. 1). The residual Ag particles, left during the MACE process, are removed using a concentrated (5 M) solution of HNO3. Finally, the PMMA layer is removed in acetone. Ohmic contacts are defined on p-type Si NWAs using a sputtered ~50/500 nm Cr/Au, and on n-type using ~500 nm Al. Different metals are used to ensure ohmic contact behavior for the two doping types. The native oxide is removed prior to metallization in a 4% HF solution for 2 min. Rapid thermal annealing in ambient Ar at 450 °C is used to improve the contact characteristics.

class="figure_img" id="Figure1"/>
Download
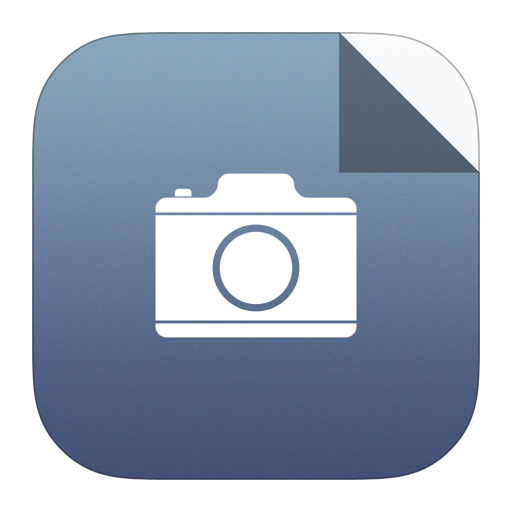
Larger image
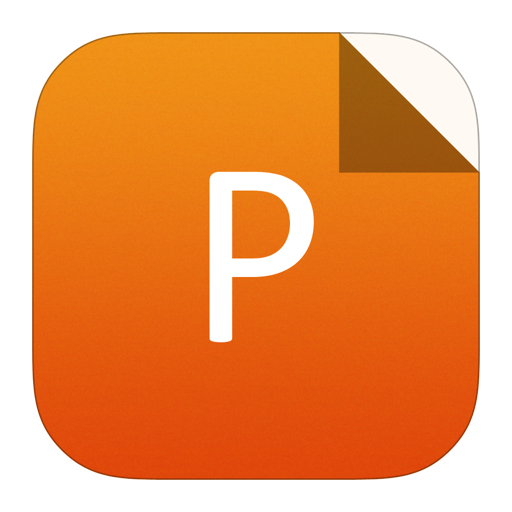
PowerPoint slide
Figure1.
SEM of (a) side view of a MACE etched Si nanowire array and (b) top view after metallisation.
For the suspended graphene layer, the Si NWA was etched in a two-step MACE process. A clean ~1 × 1 cm2 (100) p-Si sample was used with ρ = 1–5 Ω cm. In the first step the sample was immersed in 4 mL AgNO3 : 4 mL of 50% HF : 12 mL H2O for 10 min at room temperature. This nucleates Ag nanoparticles (NPs) on the surface. After rinsing the sample is then immersed in 10 mL H2O2 : 4 mL 50% HF : 6 ml H2O for 10 min. The Ag NPs act as catalyst for the oxidation and etch process, leaving behind vertically aligned NWs (see Fig. 2). The relatively high volume of 4 mL AgNO3 was used for the graphene experiments to deliver sharp unbundled NWs[18] (see Fig. 3) unlike in Fig. 2 where the AgNO3 volume was half, 2 mL. Using a higher concentration of AgNO3 in the mixture results in shorter NWs, however the unbundled NWs allows more access to the graphene back surface[19]. The difference in the Si NWA geometry caused by one-step and two-step MACE[20] has no impact on the results as the role of the NWA is as passive support only in the graphene sensor. Multilayer (3–8 layers) CVD graphene on Ni was used for the suspended graphene experiments. The recipe for the graphene transfer process uses a PMMA supporting layer as reported in Refs. [21, 22]. Ni is etched in 3 HPO4 : 3 HNO3 : 1 CH3COOH : 1 H2O. The detached graphene is then transferred onto the Si NWA. Finally, the PMMA layer is dissolved in acetone vapor at 86 °C for 1 h, resulting in a very strong bond between the graphene and the Si NWA. The same process was applied to transfer graphene onto a clean SiO2 control sample.

class="figure_img" id="Figure3"/>
Download
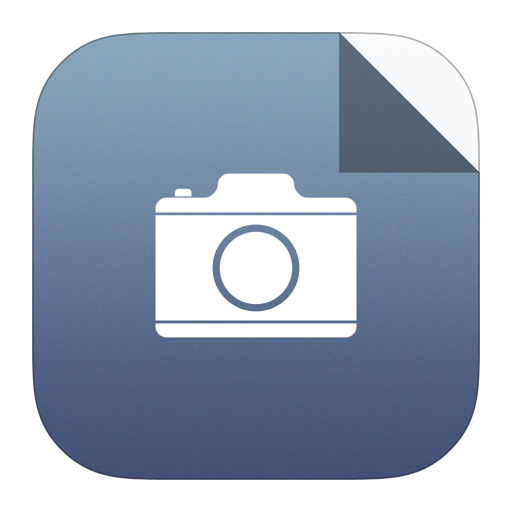
Larger image
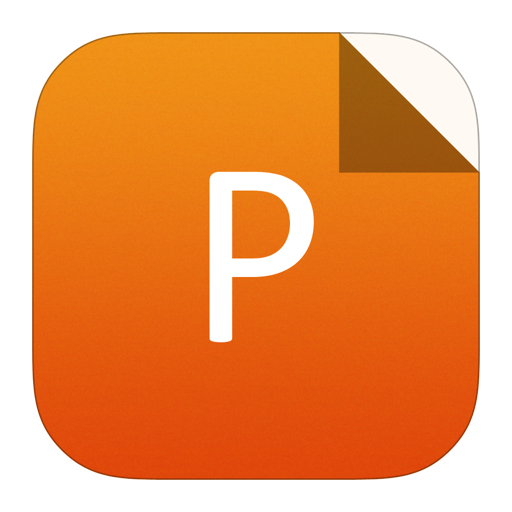
PowerPoint slide
Figure3.
SEM of the nanowire array used for suspended graphene sensing using 4 ml AgNO3.
2.2
Measurement set-up
Electrical measurements are performed using an Agilent 4155B or Keysight B1500A semiconductor device analyzer. The Si NWA sensors are biased at constant voltage and the current is measured as a function of time in steps of 4 s. For the graphene-based sensors, a constant current of 50 μA was supplied and the voltage variation was measured as a function of time. The schematic of the experimental set-up can be found in Fig. 4(a). NH4OH (28 wt% NH3 in H2O) is introduced into the measuring chamber using a 3.5 cm high cylindrical beaker with a diameter of 3.5 cm and positioned ~1 cm from the sensor. Due to the low concentration of NH4OH, the dissociation process follows mainly NH4OH ? NH3 + H2O. The mass transfer factor of NH3 is, k ≈ 0.32 × 10?2 m/s for very low wind speed, thus NH3 evaporates readily from the NH4OH pool and will arrive within ~6 s at the surface of the sensor[23, 24]. It was found that a 0.5 mL pool of NH4OH was sufficient to saturate the Si NWA samples. The electrical measurements on the Si NWAs are carried out by contacting the NWs with a flat spring-loaded probe tip of 3 mm diameter and a back contact via the Cu back plate. For the graphene measurements, two of the four 3 mm diameter spring-loaded probes are used in a horizontal configuration, separated by ~3 mm and aligned vertically. All probes are Au coated to avoid corrosion.

class="figure_img" id="Figure4"/>
Download
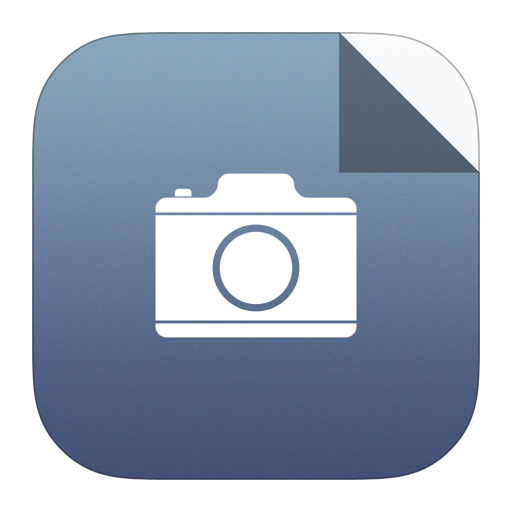
Larger image
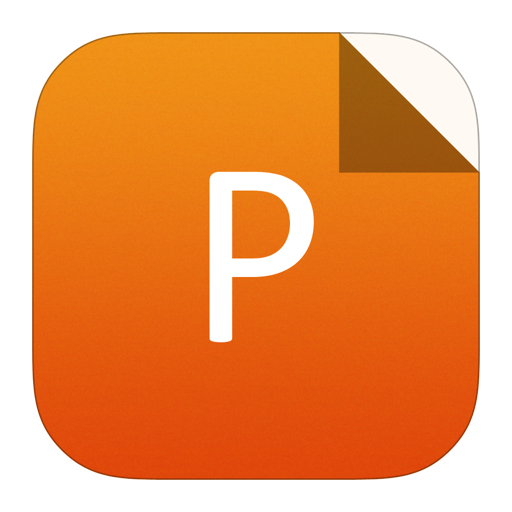
PowerPoint slide
Figure4.
(Color?online)?(a) The measurement set-up for resistance measurements. 1: z-axis translation, 2: flat spring loaded probe tip for Si NWA measurements, 3: back plate for back contact, 4: 4 parallel, equidistant (3 mm) probe tips for graphene measurements. Probe configuration 2 and 4 can be interchanged. All probe tips are Au coated and have a diameter of 3 mm. (b1) Closed container and schematic set-up ((b2) container, (b3) conical flask attached to container) used for low frequency noise measurements on Si NWA.
The low-frequency noise measurements use a dedicated closed cylindrical container with a volume of V ≈ 1.6 cm3 (see Fig. 4(b)). The sample was saturated with evaporating NH3 before measurements. The container was placed in an E-M shield and the devices biased using a battery. Contacts were made with a 3 mm diameter Au top probe under controlled pressure onto the NWA and a Cu back plate. Noise data was obtained in a frequency range from 1 to 1 kHz at 300 K for the NWA under constant bias. Background noise was measured using the zero-biased set-up and subtracted from the total device noise. The voltage fluctuations SV from the load resistor RL connected in series with the NWA were analyzed using a SR770 FFT Spectrum Analyzer. The spectral noise density of the short circuit current fluctuations, SI, was calculated using the expression:
$${S_{ m I}} = {S_{ m V}}{left[ {frac{{{R_{ m L}} + {R_{ m d}}}}{{{R_{ m L}} {R_{ m d}}}}} ight]^2},$$ ![]() |
where Rd is the sensor’s differential resistance.
3.
Results and discussion
3.1
Measurements on Si NWAs
To measure the response to evaporating NH3, the beaker with 0.5 mL NH4OH was placed in the container and the lid was closed[25–28]. For the desorption process the lid and the NH4OH recipient were removed from the container. Since the different sensors have different initial resistance values, the resistance change is normalized to the resistance value in air. Fig. 5(a) shows the measured adsorption/desorption characteristics on the p-Si NWA. The adsorption characteristic of NH3 is repeated in Fig. 6(a) (dashed line).

class="figure_img" id="Figure5"/>
Download
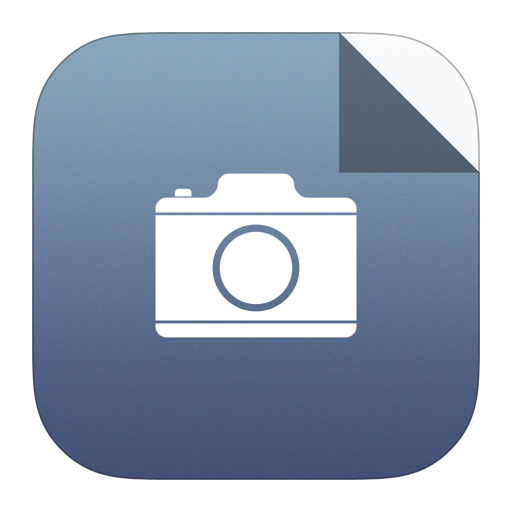
Larger image
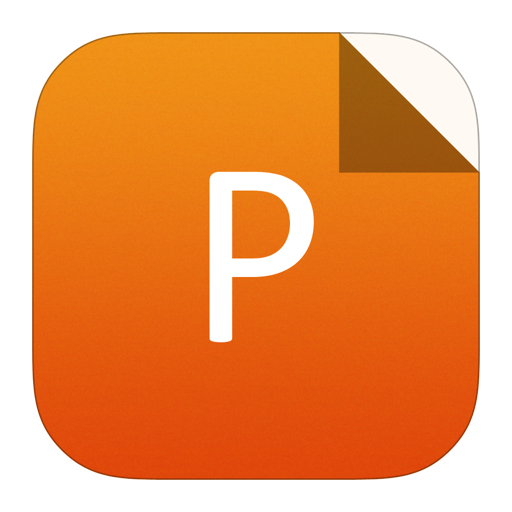
PowerPoint slide
Figure5.
(Color online) (a) The measured normalized resistance variation as a function of time for the adsorption/desorption process on p-Si NWA. (b) The normalized low frequency current noise spectra with and without NH3 (both axes are on log scale).
NH3 is introduced at t = 0.12 h, the resistance changes immediately and full saturation of the characteristics is reached within 0.08 h (4.8 min). The normalized resistance changes by a factor of 2. The increase in resistance confirms the electron donor characteristics of NH3. The same measurement on n-Si NWA is shown in Fig. 6(a) (full line). The normalized resistance change is a factor of ~10, whilst the time to full saturation is approximately half of that of the p-type sample. The improved response of the n-type wires is due to longer NWs in the array for n-type wires compared to p-type wires for the same etch recipe as described in Ref. [12]. A second factor that plays a role in the response is the thickness of the native oxide surrounding the NWs and the diameter of the wires. These parameters are strongly influenced by the doping type and density of the original Si wafer. The response of the n-Si NWA is opposite to that of the p-Si NWA, as expected. The electron donating character of NH3 depletes the p-Si surface whilst it accumulates electrons at the n-Si surface. The desorption shows a “fast” and slow response time. Initially, desorption and adsorption are at approximately the same rate. However, in order to fully recover, all NH3 molecules need to be removed from the NWA which takes ~2 h. The NWA traps the NH3 molecules between the NWs slowing the desorption process. Heating of the NWA can improve the desorption rate. The low frequency noise measurements for p and n-Si NWA are given in Figs. 5(b) and 6(b), respectively[29–31]. Interestingly, the variation of the low frequency noise for both samples are in the same direction because NH3 passivates the electron traps in the oxide that surrounds the NWs, resulting in a decrease in carrier number fluctuations in the Si NW conducting channel and thus decreases the low-frequency noise. The relevance of this is for selectivity as other gases with a different influence on the oxide traps related to the MACE process might cause similar resistance changes but different variations of the electrical noise[8, 10]. In Fig. 5(b) a generation–recombination (GR) shoulder appears in the low-frequency noise plot at ~1 kHz. This specific GR trap is not influenced by the gas adsorption because the trap does not occur in the oxide layer but is associated to a bulk-related trap in the NWs.

class="figure_img" id="Figure6"/>
Download
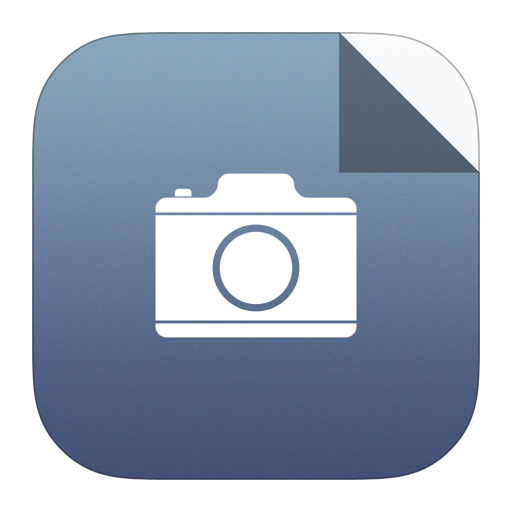
Larger image
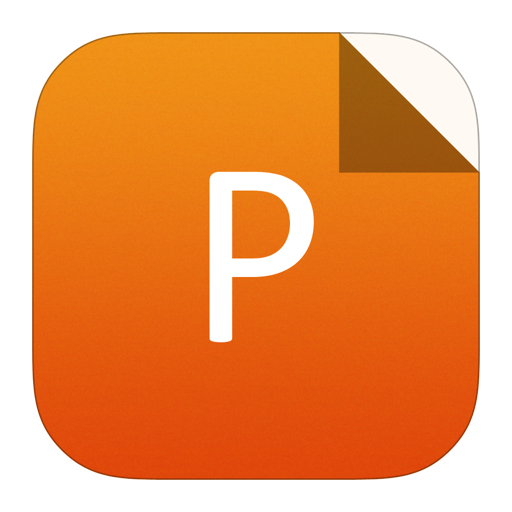
PowerPoint slide
Figure6.
(Color online) (a) The measured normalized resistance variation as a function of time for the adsorption process on n-Si NWA (full line) and p-Si NWA (dashed line). (b) The normalized low frequency current noise spectra with and without NH3 (both axes are on log scale).
3.2
Measurements on suspended graphene
The measurements on suspended graphene on a Si NWA and graphene-on-oxide were carried out in a 2-horizontal probe configuration. The graphene surface area is approximately 1 cm2, similar to the NWA area in the previous measurements. In Fig. 7 the normalized resistance is plotted as a function of time for both suspended graphene on a Si NWA and graphene on SiO2.

class="figure_img" id="Figure7"/>
Download
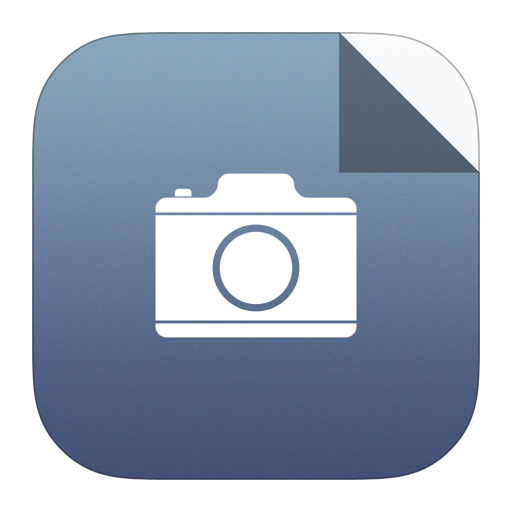
Larger image
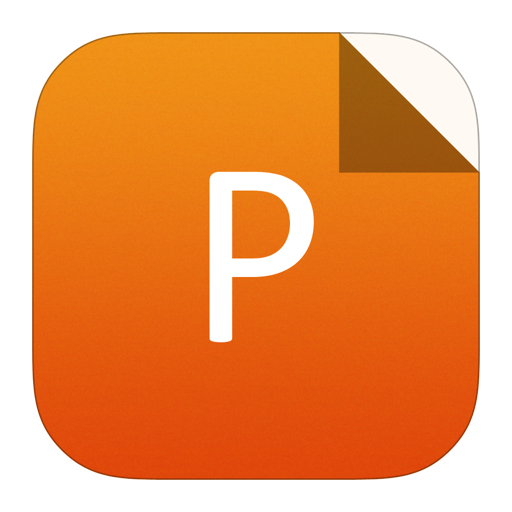
PowerPoint slide
Figure7.
The measured normalized resistance variation as a function of time for the adsorption process on suspended graphene on an NWA (full line) and graphene on SiO2 (dashed line).
The relative resistance increases in both systems, similar to the response of the p-Si NWA, implying that the graphene layer is p-type. Comparing the response of suspended graphene to graphene on SiO2 shows some interesting features: the initial response rate (variation of 10% from the initial value) of suspended graphene is ~10 times faster than graphene on SiO2. Similarly the desorption rate of suspended graphene is ~20 times higher than that of graphene on SiO2. Although none of the sensors have saturated within 1 h 45 min, the amplitude of the normalized resistance of suspended graphene is 1.2 × higher within the same timeframe. The difference in behavior is caused by both the increase in effective surface area of suspended graphene compared to graphene on SiO2―since the bottom graphene surface has become available for adsorption―as well as the different diffusion dynamics of the NH3 molecules in the two systems. When NH3 molecules reach the sensors, some will adsorb on the top surface whilst others will diffuse through the system before adsorption. Since the suspended graphene allows more room for this diffusion process through the gaps between the nanowires its rate is higher, both in the adsorption as well as the desorption process.
Comparing the time to saturation of the suspended graphene system to the nanowire-only system shows that the response rate of the NWA-only system is at least 5× higher than the graphene system, indicating slower adsorption dynamics on graphene. Similarly, the amplitude of the resistance variation with the same pool of NH4OH is much higher for the NWA-only sample. This feature is related to the number of available adsorption sites in the system influenced by the effective surface area but also by the uncompleted bond density at the surface that allows adsorption of NH3. Since the density of NWs is approx. 109 cm2 and taking an average NW diameter of 250 nm, the effective surface area of the 60 μm long Si NWA is ANWA ≈ 3 × 103 mm2. This is much larger than that of suspended graphene with AsusGr ≈ 20 mm2 (determined approximately by the distance between the probes and the probe diameter).
The influence of the surface states can be analyzed via low-frequency noise measurements. The normalized low-frequency current noise power spectral density for the graphene systems is given in Fig. 8. The low-frequency noise spectral density of the graphene system is proportional to I2. This implies that the electrical current I does not drive the fluctuations but merely makes the fluctuations in the sample visible if Ohm’s law is used[32]. This is what is usually observed for graphene where noise is related to carrier number and/or mobility variations, similar to the Si NWA system. The amplitude of the normalised noise spectral density measured in our system is also within that reported for graphene: 10?7–10?9 Hz?1 at 10 Hz[24]. In our system, no specific G-R centers are observed in the samples in contrast to Ref. [17]. In consequence, the noise measurements in this case cannot identify directly the molecule type adsorbed. From Fig. 8 we can observe that the noise spectra follow a 1/f characteristic and that in the presence of NH3 molecules, the noise is reduced. This behaviour is identical to that on Si NWA proving that NH3 passivates the surface traps in the graphene system. Another observation is the higher amplitude of the normalized noise in the suspended graphene layer. This is consistent with the hypothesis that the amplitude of the response to NH3 is driven by the surface condition of the sensor. The amplitude response of the suspended graphene is indeed larger than that of graphene on oxide.

class="figure_img" id="Figure8"/>
Download
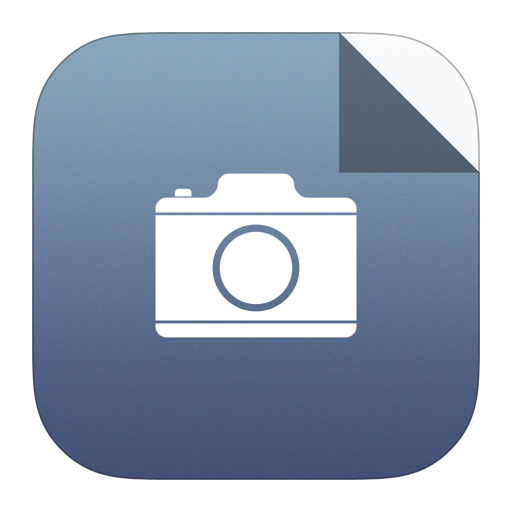
Larger image
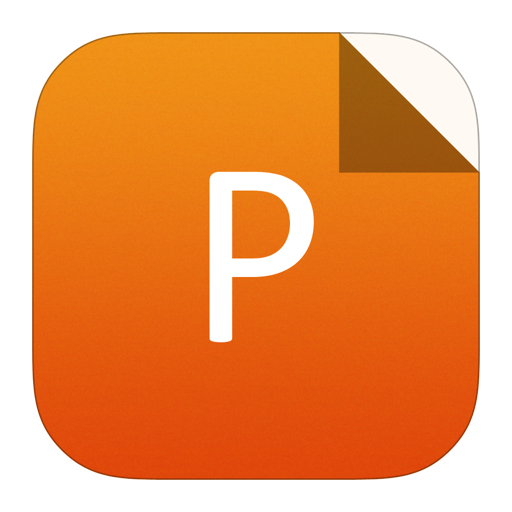
PowerPoint slide
Figure8.
?(Color?online)? The normalized low frequency current noise spectra with and without NH3. (a) Suspended graphene on Si NWA. (b) Graphene on SiO2 (both axes are on log scale).
4.
Conclusion
The response of an n- and p-type Si NWA to NH3 evaporating from a pool of 0.5 ml NH4OH at room temperature is compared to the response of graphene suspended on a Si NWA and graphene on SiO2. The response is given in terms of variations of the normalized resistance as a function of time and the normalized low-frequency current noise spectral density with and without the presence of NH3. It is found that the response is dependent on both the effective surface area as well as on the density of surface traps. The Si NWA systems offer an easy route towards increasing the effective surface area and as a consequence the variation of the normalized resistance upon admission of NH3 is larger than for the graphene system with a similar top surface area. The response rate is strongly dependent on the diffusion of NH3 through the sensor system. It is observed that the response rate on Si NWAs is faster than suspended graphene that in turn is faster than graphene on SiO2. This is because NH3 can more easily diffuse through a NW system. The noise spectra show the relationship between the density of surface traps and the response of the sensor. Although the density of surface traps is larger in suspended graphene than in the Si NWA, the much larger effective surface area of the Si NWA causes a larger amplitude response. However, comparing suspended graphene to graphene on SiO2 it is observed that due to the larger trap density in suspended graphene and larger effective surface area its amplitude response is larger than that of graphene on SiO2.
Acknowledgment
CP acknowledges financial support of EPSRC via the EEE department. C.L. and K.F. acknowledge support from e-on for the Si NWA sensing work.