1.
Introduction
Photonic integrated circuits (PICs) have been very promising for many applications, including optical interconnects in different scales from long-haul networks to on-chip systems[1, 2]. Various platforms for PICs have also developed successfully, like silica[3], polymer (e.g., SU-8)[4], silicon[5], etc. Among them, silicon photonics on a silicon-on-insulator (SOI) platform has been recognized as one of the most popular options in the past decade because of the compatibility with the complementary metal–oxide–semiconductor (CMOS) technology. The CMOS compatibility enables excellent process control, low cost and scalability to high-volume and high-yield manufacturing. Moreover, the ultra-high refractive index-contrast of SOI nanowires enables ultra-sharp bending (e.g.,R ≈ 1–2 μm[6]) and thus silicon PICs are usually several orders of magnitude smaller than silica PICs. Passive silicon photonic devices have shown excellent performances for different functional elements, including multi-channel mode (de) multiplexers[7], polarization beam splitters (PBSs)[8], polarization splitter-rotators (PSRs)[9], arrayed-waveguide gratings (AWGs)[10], optical micro-cavities[11, 12], and efficient optical mode transformers for efficient coupling to optical fibers[13, 14].
On the other hand, as a typical indirect-bandgap semiconductor, silicon is not a good option for active photonic integrated devices. Fortunately, hybrid silicon photonics has been proposed and demonstrated by combining silicon with other active materials, like germanium[15, 16], III–V semiconductor[17], metal[18, 19], etc. Various active silicon photonic integrated devices have been developed, including high-speed Ge/Si photodetecor[15], high-gain avalanche photodiode[16], and high-speed III–V/Si modulator[17]. More recently, graphene has also been introduced to the platform of silicon photonics as an attractive candidate to overcome the drawback of silicon for realizing active photonic devices. In particular, a monolayer graphene is ~0.34 nm thickness, which makes it straightforward to integrate with SOI nanowires flexibly without any complicated design and fabrication processes. A unique property of SOI nanowires with graphene is that the optical mode in an optical waveguide is very similar to that in the regular SOI nanowires without graphene, so that there is almost no mode mismatching when the passive SOI nanowire and the active SOI nanowire are connected. This makes silicon-graphene photonic devices very attractive.
As demonstrated recently, graphene has been utilized to realize photodetectors on silicon since it has very high carrier mobility (up to ~ 200 000 cm2V?1s?1)[20] and can also absorb light in a broad band ranging from ultraviolet to mid-infrared[21–27]. When utilizing silicon-graphene hybrid optical waveguides, light absorption can be accumulated very significantly by extending the waveguide length, which makes it possible to enhance the responsivity of the graphene photodetectors. It is also possible to combine graphene and silicon to form a Schottky diode, so that the light absorption in silicon can also be utilized, which helps enhance the responsivity greatly for short-wave light[21–24]. As is well known, the Fermi-level of graphene is adjustable by modifying the carrier density in graphene. Consequently, the silicon-graphene Schottky diode is also helpful to achieve efficient all-optical modulation by utilizing the carrier transport from the silicon region to the graphene sheet[28]. Furthermore, graphene has very strong optical nonlinear effects[29], and thus it could be used as saturable absorbers for pulse generation. Finally, regarding the transparency and electric-conduction, graphene can work very well as a promising transparent nano-heater, as demonstrated in recent years[30–34].
In this paper, we give a review on recent progress of silicon-graphene photonics devices, including high-responsivity silicon-graphene photodetectors, efficient all-optical modulator, as well as high-efficiency thermal-tuning devices.
2.
Silicon-graphene photodetectors from mid-infrared to ultraviolet
Since graphene has some unique optoelectronic properties, e.g., high carrier mobility (200 000 cm2V?1s?1 at room temperature)[20, 35], broadband absorption of πα = ~ 2.3% per layer for normal incidence illumination[36, 37], it is very attractive to introduce graphene as a novel material for realizing photodetectors that can detect light beyond the spectral absorption range of silicon. Instead of normal incidence light on a sheet of graphene, in-plane propagation of light in silicon-graphene hybrid optical waveguides has been widely explored in order to enhance interaction length of light with graphene by utilizing the interaction of the evanescent field from a silicon waveguide with graphene. In this case, the responsivity of the graphene photodetectors can be enhanced very greatly, as demonstrated in 2013[25–27] . The responsivity of the demonstrated photodetectors is 0.05–0.1 A/W and the bandwidth is 18–20 GHz[25, 26] for near-infrared light of 1.55 μm. This proved that the silicon-graphene platform has a great potential to achieve on-chip photodetectors with reasonable performances. It has also been demonstrated that silicon-graphene waveguide photodetector works for mid-infrared light[27]. Fig. 1(a) shows the silicon-graphene waveguide-type photodetector based on a silicon-graphene Schottky junction, where there are two gold electrodes fabricated to contact with the silicon waveguide and the graphene sheet, respectively. For this silicon-graphene photodetector, the responsivity for mid-infrared (~2.75 μm) is much higher than that for near-infrared (1.55 μm). This can be explained from the energy diagram of silicon-graphene Schottky junction. For the mid-infrared light excitation, the photon energy is less than twice the difference between the Fermi level and the Dirac point, and thus indirect transition processes may happen. This is different from the case with the near-infrared (1.55 μm) light excitation, in which case the direct transition from the valence band to the conduction band. On the other hand, there is a 0.31 eV barrier for the silicon-graphene junction, which makes it possible to achieve higher photocurrent when working with a reverse bias. In Ref. [27], the measured responsivity for the fabricated silicon-graphene photodetector at room temperature is as high as 0.13 A/W for incident light (and about 0.6 A/W for coupled light) when operating at ?1.5 V.

class="figure_img" id="Figure1"/>
Download
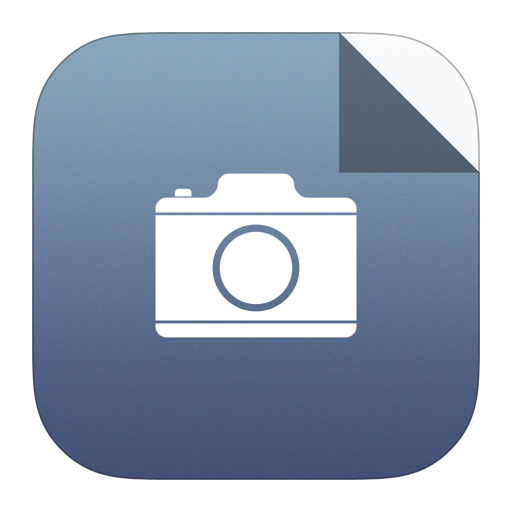
Larger image
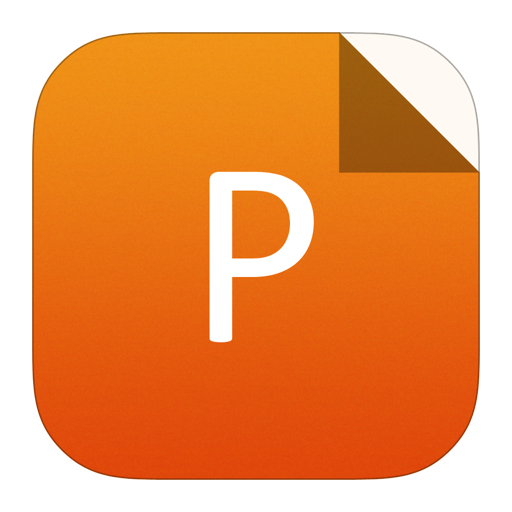
PowerPoint slide
Figure1.
(Color online) (a) Schematic of the silicon-graphene waveguide-type photodetector. (b) Measured photocurrent with different input powers (@2.75 μm)[27].
The structure with a silicon-graphene Schottky diode is also very useful for normal-incident photodetection when operating at the short-wave range. In this case, the light absorption in silicon can contribute to enhance the responsivity very greatly[22–24]. Recently, a silicon-based graphene conductive photodetector was demonstrated by putting a graphene sheet on an n-type silicon substrate with a doping level n < 10 16 cm?3. The response time was only ~1.48 ms, and the responsivity was ~106 A/W with an input optical power less than 10 pW[22]. A p-type silicon-graphene conductive photodetector was also demonstrated with further improved response time of ~3 μs and reduced responsivity of ~104 A/W under an input optical power of 0.112 μW[23].
According to the circuit model given in Ref. [24], the photo-current of the silicon-graphene conductive photodetector (see Fig. 2(a)) is given by the following simplified equation when the optical power is lower than tens microwatt,
$${I_{{ m{ph}}}} approx frac{{UDelta nemu }}{{{{left( {frac{{{R_{ m{C}}}nemu }}{{sqrt {L/W} }} + sqrt {L/W} } ight)}^2}}}, $$ ![]() |
where RC is the graphene-metal contact resistance, L and W are respectively the length and width of graphene sheet, n is the carrier concentration in graphene and Δn is the carrier concentration variation in graphene. It can be seen that the photocurrent and responsivity have strong dependence on the graphene sheet size (L and W), and the graphene-metal contact resistance RC. The strong size dependence of responsivity of the photodetector has been observed experimentally. Fig. 2(b) shows the measured responsivity of silicon-graphene photodetectors with different graphene sizes, which is consistent with the theoretical prediction from the circuit model. It can be seen that the responsivity can be improved by reducing the graphene area if the ratio L/W is fixed. When the graphene area is fixed, the responsivity is dependent on the graphene-metal contact resistance RC and graphene sheet resistance RG. One has a maximal responsivity when the resistances RC and RG are equal.
More importantly, the temperature dependence of the responsivity for the silicon-graphene photodetector was studied for the first time in Ref. [24], as shown in Figs. 2(c) and 2(d). It can be seen that the responsivity increases greatly as the temperature decreases. When operating at low temperature, the responsivity can be enhanced greatly. For example, when the temperature T = ?25 °C, the responsivity increases to as high as 107 A/W and it is able to detect an optical power as low as ~6 pW. This is attributed to the smaller contact resistance and graphene resistance. The ability for the detection of weak visible light can be even improved by lowering the temperature further.

class="figure_img" id="Figure2"/>
Download
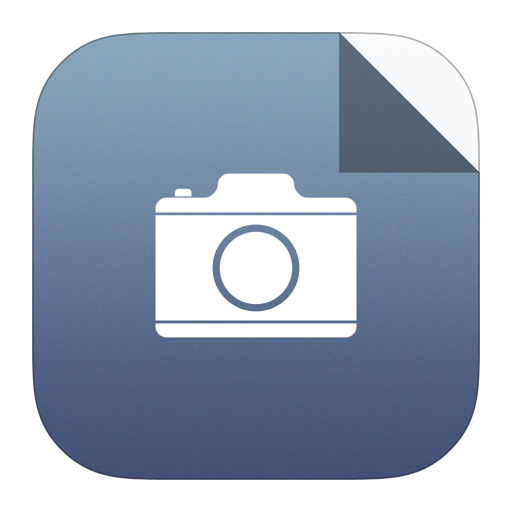
Larger image
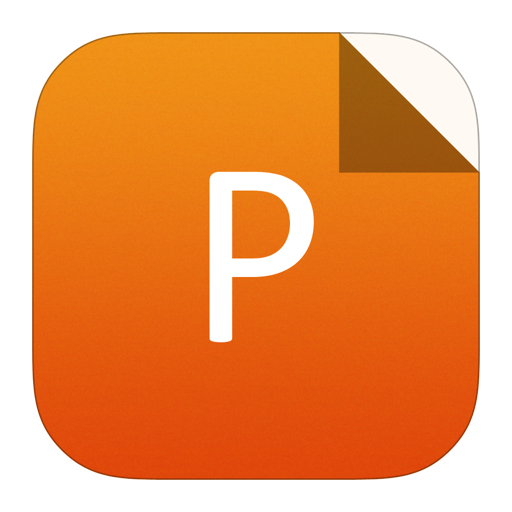
PowerPoint slide
Figure2.
(Color online) Silicon-graphene conductive photodetector. (a) Schematic configuration. (b) Measured responsivity when designed with different graphene sheet dimensions. (c) Measured responsivity with L/W = 20/100 μm when operating at 27, 40, 60, and 80 °C, respectively. (d) Measured responsivity when operating at ? 25 °C[24].
More recently, a self-powered graphene?silicon ultraviolet photodetector with high performances was also demonstrated[21], as shown in Fig. 3(a). For traditional pure silicon ultraviolet (UV) photodetectors, the performance is mainly limited by the ultra-high light absorption of silicon. For example, the penetration depth of UV light in silicon is less than 20 nm for the wavelength λ < 370 nm [38], which results in very high carrier recombination during the diffusing process (at the scale of ~100 nm). In contrast, the special ultra-shallow graphene-silicon Schottky junction is beneficial to separation of the photo-generated electron?hole (e?h) pairs without any external bias, owing to most hot carriers induced in the depletion region. In order to minimize the dark current and not to degrade the photocurrent, a thin (~2 nm) silica layer was introduced intentionally between graphene and silicon. This UV photodetector exhibits a > 100% internal quantum efficiency, a high responsivity of ~0.2 A/W, and a fast temporal response of ~5 ns, as shown in Figs. 3(b) and 3(c). With further spin-coating silicon quantum dot on the graphene-silicon Schottky junction, the responsivity can be enhanced (0.495 A/W) without sacrificing the device operation speed (< 25 ns)[39]. On the other hand, the metal–semiconductor–metal (MSM) lateral structure is a better choice for high-speed photodetectors. Recently, a lateral interdigitated MSM graphene-silicon Schottky photodetector has been demonstrated with a response time of < 2 ns, showing the potential of GHz frequency range operation [40]. In order to further extend the detection spectrum of silicon-graphene photodetector to cover the 1.55 μm region for optical communication, plasmonic effect was utilized for light trapping to enhance the light absorption of graphene[41]. In this structure, the silicon-graphene Schottky diode works for separating the hot carriers generated in the graphene layer. In this case, the measured responsivity of the fabricated silicon-graphene photodetector with Au-nanoparticles is up to 83 A/W and the rising time is less than 600 ns[41].

class="figure_img" id="Figure3"/>
Download
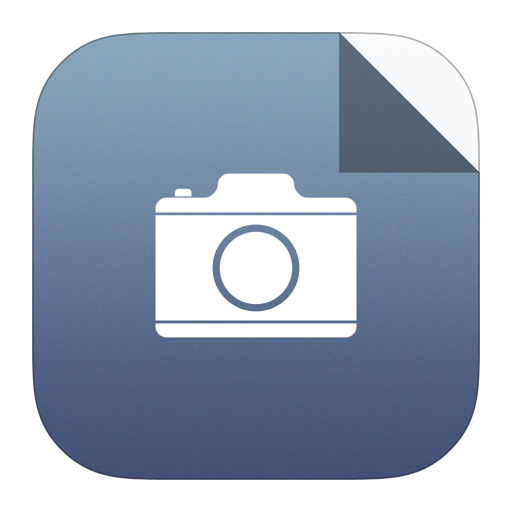
Larger image
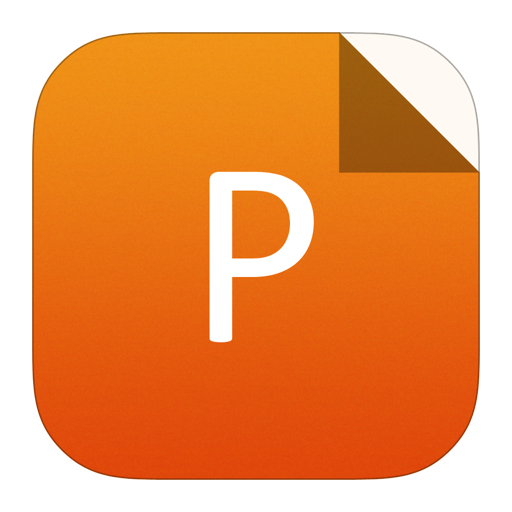
PowerPoint slide
Figure3.
(Color online) (a) Schematic view of the silicon-graphene UV photodetector. (b) Measured UV spectral responsivity for the photodetectors (D1?D3) and the theoretical responsivities with and without reflection. (c) Temporal response of the photodetector for λ = 375 nm when the bias Vb = 0[21].
3.
Silicon-graphene photonic devices for all-optical modulation
Fig. 4(a) shows the three dynamic processes for electrons and holes in graphene when it is excited optically with high power[42]. These three processes have different temporal scales. When excited by a strong laser pulse, the electrons jump from the valance band to the conduction band in the monolayer, and would be thermalized and cooled down to form the hot Fermi-level distribution within 10?150 fs. The intraband photon scattering further cools the thermalized carriers within 150?1000 fs. The electron?hole recombination is the dominant process in the temporal scale of > 1 ps. Before the recombination process, the conduction band would be filled with the hot carriers and thus light absorption becomes saturable because of Pauli blocking effect. Compared to traditional semiconductor materials for saturable absorption, graphene has some unique advantages, e.g., low saturation power density, broad band, ultrafast response [43]. In Ref. [43], a mode-locked fiber laser was demonstrated with graphene saturable absorber transferred to the fiber facet and ultrashort soliton pulses (~756 fs) at the telecommunication band were generated, as shown in Fig. 4(b).
The modulation depth of graphene absorption can be tuned in a wide range from 66.5% to 6.2% by varying the graphene thickness, as shown in Fig. 4(c). The optical intensity for saturable absorption and the saturation carrier density are IS = 0.71 MW/cm2 and NS = 5.84 × 1013 cm?2 for the 2?4 layers graphene. For the 9–11 layers of graphene, one has IS = 0.61 MW/cm2, and NS = 8.16 × 1014 cm?2. In comparison with the single-walled carbon nanotubes (SWNTs)[44–46] and semiconductor saturable absorber mirrors (SESAMs)[47], the atomic-layer graphene has much smaller non-saturable loss, one order of magnitude lower saturation intensity, and 2?3 times larger modulation depth. Furthermore, since graphene saturable absorber works well in a broad band, a Q-switched laser at 1.06 μm[48] and a mode-locked laser at 2 μm[49] have also been demonstrated recently. Sub-100 fs laser pulses have been achieved because of the ultrafast electron–holes recovery time in graphene[50].
Enhanced all-optical processing has also been demonstrated by combining graphene with silicon photonic waveguides. A passive mode-locked laser at 1.56 μm was demonstrated with a 7 nm spectral bandwidth and a 1.4 ps pulse width[51], as shown in Fig. 4(d). In this configuration, an SOI nanowire covered with graphene was inserted to the laser cavity to serve as an on-chip saturable absorber.

class="figure_img" id="Figure4"/>
Download
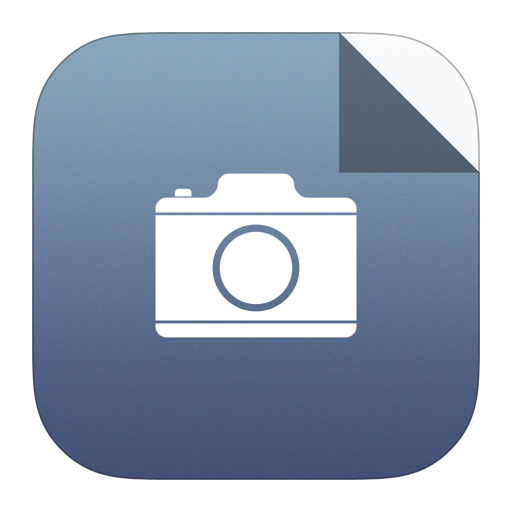
Larger image
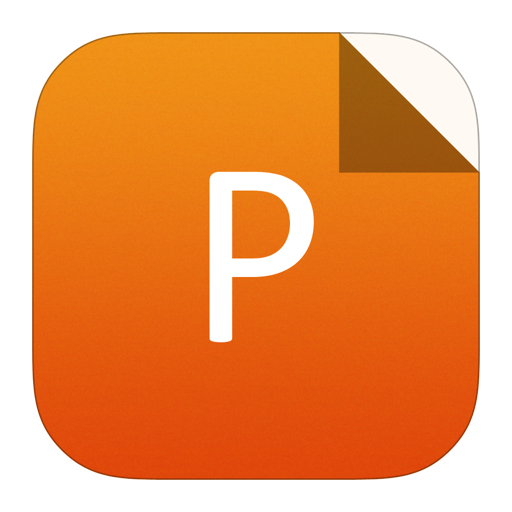
PowerPoint slide
Figure4.
(Color online) (a) Three dynamic processes for electrons and holes in graphene when it is excited optically with high power[42]. (b) Schematic of graphene mode-locked fiber laser[43]. (c) Nonlinear absorption of graphene films with different number of layers[43]. (d) Schematic of a mode-locked laser with graphene saturable absorber on an SOI nanowire[51].
As discussed above, the power density for saturable absorption in graphene is up to MW/cm2[43]. In Ref. [28], an optical-induced transparency (OIT) effect for all-optical modulation in silicon-graphene hybrid nanophotonic waveguide was observed with ultra-low threshold of power density for the first time. The experimental result shows that the pump power density required is as low as ~ 2 W/cm2. Fig. 5(a) shows the silicon-graphene hybrid nanophotonic waveguide with the OIT effect. In this configuration, graphene is contacted with silicon directly and a Schottky junction is formed. Fig. 5(b) shows the mode profiles of TE- and TM-polarizations for the hybrid nanophotonic waveguide, respectively. With this structure, the signal light at 1550 nm propagating along the hybrid nanophotonic waveguide can be modulated locally and non-locally by the normal-incident pump light at 635 nm, as shown in Fig. 5(c). It can be seen that the output power from the waveguide is improved when the pump laser is on. It means that the graphene absorption is reduced. Figs. 5(d) and 5(e) show the measured modulation depths for TE- and TM-polarization mode as the length of the waveguide covered with a graphene sheet increases. It can be seen that TM polarization mode has higher modulation depth than TE polarization. This is attributed to the enhanced evanescent field for TM polarization mode. The non-local OIT effect is also characterized as shown in Fig. 5(f). From this figure, it can be seen that the OIT effect still occurs even when the pump light position is ~ 4 mm far away from the silicon core region. The OIT effect happens due to the photo-generated carriers transport from the silicon region to the graphene sheet, which can be explained from the energy diagram given by the inset in Fig. 5(f). More importantly, the threshold power density for the OIT effect is only about 2 W/cm2, which is 6 orders lower than the conventional saturable absorption effect in graphene. This makes the OIT effect in the silicon-graphene hybrid nanophotonic waveguide very attractive for low-power all-optical modulation in the future.

class="figure_img" id="Figure5"/>
Download
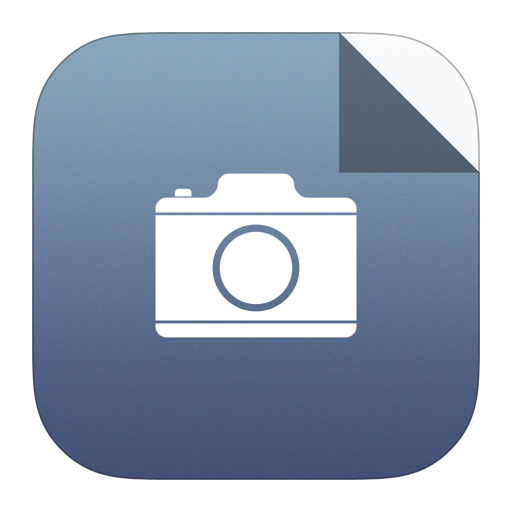
Larger image
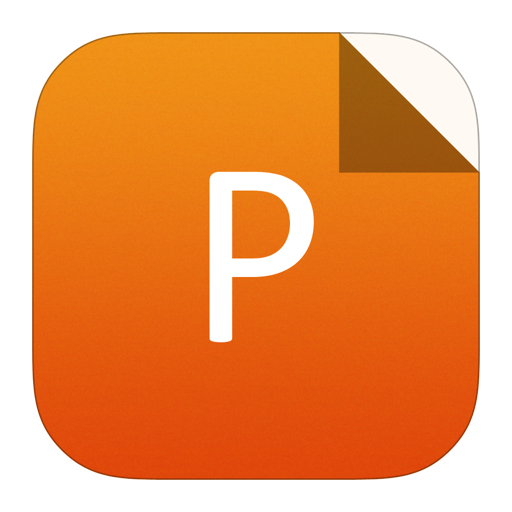
PowerPoint slide
Figure5.
(Color online) (a) Schematic of silicon-graphene hybrid nanophotonic wire. (b) Crossing section of hybrid nanophotonic wires and optical mode profile for TE- and TM-polarization modes. (c) Schematic configuration for local and nonlocal OIT effects in a silicon-graphene hybrid nanophotonic wire. (d) Temporal dynamics of the OIT effect for TE- and TM-polarization modes. (e) Measured modulation depths of the OIT effect in the hybrid nanophotonic wires. (f) Measured modulation depths for the non-local OIT effect (Inset: energy diagram of silicon-graphene junction)[28].
4.
Silicon-graphene photonic devices for thermal-tuning/switching
Switchable/tunable photonic devices are playing very important roles for many applications. Among various mechanisms for realizing tunable/switchable photonic devices[52, 53], utilization of the thermal-optic effect is one of the most popular ways because there is no special requirement for the waveguide materials[54]. Furthermore, the structural design and the fabrication process are very simple and convenient. In particular, it is even more popular for silicon photonics because of the high tuning/switching efficiency resulting from the high thermo-optic coefficient (~1.8 × 10?4 K?1 @ λ = 1.55 μm) and the high heat conductivity (~149 W/(m·K)) of silicon[53]. There is a considerable amount of literature on thermal-tunable/switchable silicon photonic devices, including thermo-optic switches based on Mach-Zehnder interferometers (MZIs) or micro-ring resonators (MRRs)[55–60].
As is well known, metal heaters are usually used to realize thermally-tunable silicon photonic devices. In this case, the SiO2 upper-cladding layer should be thick enough to minimize the metal absorption. Such a thick SiO2 upper-cladding layer usually introduces some drawbacks for thermal tuning/switching due to the poor heat conductivity of SiO2. For example, the thermal-optical response speed decreases and the heating efficiency is lowered. Furthermore, the temperature of the silicon core region is much lower than that of the metal heater while there is a limitation for the temperature allowed for the metal heater itself. Therefore, the temperature dynamics of the silicon core is limited and the thermal-tuning range becomes limited.
As an alternative of micro-/nano-heaters, graphene is also very attractive because of the high thermal conductivity (up to 5300 W/(m·K)) at room temperature[36, 61] and high optical damage threshold. More importantly, graphene is ultra-thin to be transparent almost and introduces very little influence on the optical mode propagating in an optical waveguide[30–33]. In 2014, a transparent graphene nano-heater for SOI nanowires was proposed and demonstrated for the first time[30] . This design consists of a graphene nano-heater contacting with the silicon core directly, which helps the realization of fast and efficient thermal conduction. In comparison with the traditional metal micro-heater for silicon photonics, the graphene nano-heater has improved heating efficiency, response speed as well as larger thermal dynamic range. With such a transparent graphene nano-heater, a thermally-tunable micro-disk resonator was demonstrated[32]. A tunable silicon-based micro-ring resonator was demonstrated with a wide graphene heater covering the whole structure[31], which also shows the potential of graphene heaters for high-efficiency thermal-tuning. More recently, the transparent graphene nano-heater on silicon was also used successfully to realize thermo-optic Mach-Zehnder optical switches with slow-light nano-structures[33].
Fig. 6(a) shows the fabricated thermally tunable silicon-based micro-disk resonator with transparent graphene nano-heaters[32]. Fig. 6(b) shows the measured spectral responses as the electrical power Pheating increases. It can be seen that the resonant wavelength λres of the micro-disk resonator has a red-shift of Δλ = ~5 nm when the heating power Pheating varying from 0 to 10.5 mW. The heating efficiency ηd (= Δλ/Pheating) is estimated to be ~0.48 nm/mW, as shown in Fig. 6(c). The heating efficiency for the fabricated micro-disk resonators with different sizes is shown in Fig. 7. Here the bending radii for the micro-disks are chosen as rd = 2, 3, and 5 μm. From Fig. 7, it can be seen that the achieved heating efficiency is as high as ~1.67 nm/mW when the radius rd is reduced to 2 μm. It is possible to improve the heating efficiency further when using a micro-ring instead of a micro-disk because of the reduced heating volume. As a conclusion, this transparent graphene nanoheater provides a promising option for realizing high-efficiency, thermally tunable silicon photonic devices.

class="figure_img" id="Figure7"/>
Download
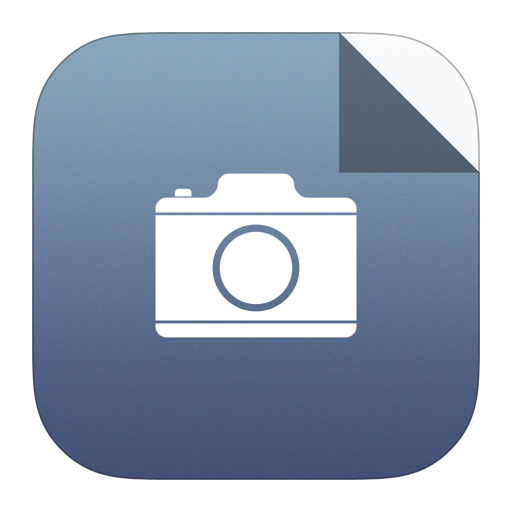
Larger image
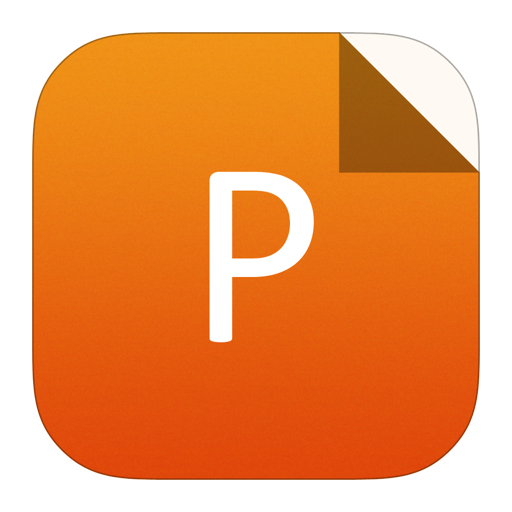
PowerPoint slide
Figure7.
(Color online) Measured heating efficiency for the micro-disk resonators (rd = 2, 3, 5 μm) using transparent graphene nanoheaters[32].

class="figure_img" id="Figure6"/>
Download
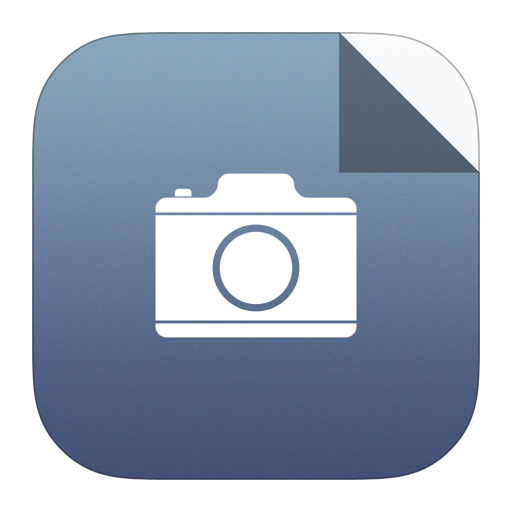
Larger image
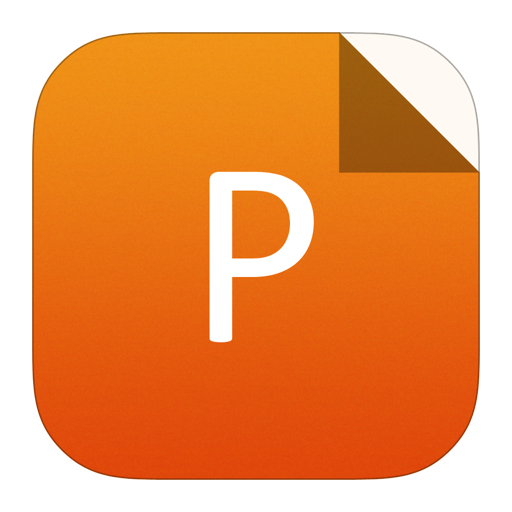
PowerPoint slide
Figure6.
(Color online) (a) Silicon micro-resonator with a graphene nano-heater. (b) Measured spectral responses as the heating power Pheating increases. (b) The measured resonant-wavelength shift Δλ[32].
5.
Discussion and conclusion
In this paper, we reviewed recent progress in silicon-graphene photonic devices for photodetection, all-optical modulation, and thermal-tuning. It has been shown that graphene has high compatibility with silicon photonics. As graphene does not introduce any significant modification of optical mode profile in the guided mode of a SOI nanowire, it is very helpful for the monolithic integration between the silicon-graphene photonic devices and offers useful properties for enhancing the capability of silicon photonic devices. As one of the most important applications of graphene, silicon-graphene photodetectors with high responsivity have been developed to cover the wavelength range from mid-infrared to ultraviolet. It is possible to realize silicon-graphene photodetector array to satisfy the demands for multi-channel optical interconnects and large-area optical imaging/sensing. Silicon-graphene structures are also very promising for all-optical modulation. By utilizing the saturable absorption of graphene, Q-switched lasers or mode-locked lasers have been developed for different wavelength ranges from 1.06 to 2 μm. An OIT effect with an ultra-low threshold of power-density has also been observed in silicon-graphene hybrid nanophotonic waveguides, which provides a promising approach for local and non-local all-optical modulation. The modulation depth of the OIT effect relies greatly on the silicon-graphene Schottky junction. It can be enhanced possibly by optimizing the Schottky junction carefully. Finally, graphene has also been used as a transparent nano-heater to work together with silicon photonic devices to achieve thermal tunability. The transparent graphene nano-heater can be placed to contact with the silicon core directly, in which way the heating efficiency can be improved greatly. In conclusion, the combination of graphene with silicon has extended the capability of silicon photonics. The key is to enhance the light–matter interaction. A possible approach is to introducing the mode-division-multiplexing technology, as shown in Fig. 8, so that light can propagate along a multimode silicon photonic waveguide forward and backward. In this way, the light–matter interaction can be enhanced by several folds, as invented by Dai[62]. More efforts are expected in the development of silicon-graphene photonic devices for realizing large-scale photonic integrated circuits.

class="figure_img" id="Figure8"/>
Download
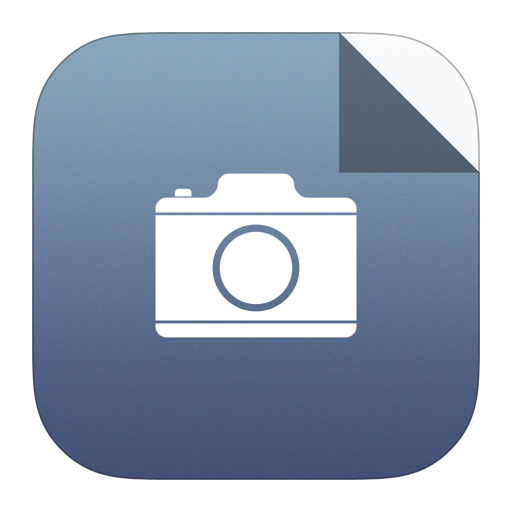
Larger image
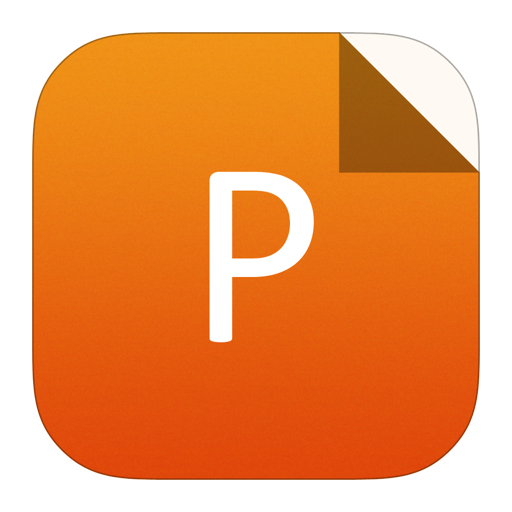
PowerPoint slide
Figure8.
(Color online) Schematic configuration of a novel optical modulator based on a multimode optical waveguide, for which the mode-division-multiplexing technology is introduced[62].