1.
Introduction
A surface electromyography (sEMG) electrode is a non-invasive technique for detecting electrical signals generated by the contraction of skeletal muscles[1, 2]. The sEMG electrode placed on the skin surface is exploited for many clinical and research purposes, ranging from diagnosing neuromuscular disorders[3, 4], studying muscle pain[5–7], and controlling prosthetic or orthotic devices[8–13]. Additionally, the sEMG electrodes should satisfy large deformation and conformally contact with the skin surface to be used as an HMI to control the motion of the external actuators.
Conventional surface electrodes affixed on the skin surface with adhesive tapes for biological signal recording limits mounting locations at large, relatively flat regions of the body, such as the wrist and chest[14]. Additionally, it also causes noise in the bio-potential measurement as motion artifacts due to the relative slippage between the electrode and skin[1, 15]. Recently, epidermal electronic systems with an ultrathin, low-modulus, lightweight and stretchable substrate that conformably laminates onto the skin surface have been designed to overcome such problems[15–18]. Tremendous efforts have been developed to achieve stretchability for biological monitoring via proper materials and structures[16, 19–22]. A stretchable sEMG electrode with self-similar serpentine structure is designed to satisfy the larger deformation of skin[23, 24]. A wireless sensor and data transmission system with physiological measurement techniques require an interface to the artificial skin, which is of interest to monitor healthy inform- ation[25, 26]. The HMI based on sEMG electrodes with serpentine structure is designed to control the motion of the quadrotor by different gestures[8]. A wearable interactive HMI has potential applications in personal mobile electronics and the Internet of Things[9]. The HMI with porous pressure sensitive rubber (PPSR) pressure sensors and strain gauges is designed to control the robot arm remotely[27]. However, beyond biological signal monitoring in the parts of the body with sharp curvature, the sEMG electrode should satisfy large deformation and conformal contact with the skin surface which is used as an HMI to control the motion of a mobile robot.
The stretchable surface electrode with self-similar serpentine structure is designed to monitor the biological signals, which could satisfy large deformability (>30%) and high areal coverage. The stretchable surface electrodes are used to record physiological signals from different parts of the human body with sharp curvature, such as the index finger and back of the neck. It is also used as an HMI to control the motion of the mobile robot via human gestures remotely. We here report an HMI based on a stretchable surface electrode that converts recorded data into signals for physiological measurement and is integrated with wireless ZigBee nodes. The electrodes laminated onto the two wrists would generate different control signals with different gestures. These control signals are transmitted to the mobile robot by wireless ZigBee transmitting nodes. Experiments have been demonstrated that the stretchable surface electrode is used to monitor biological signals and as an HMI for controlling the motion of the robot remotely.
2.
Principle and fabrication
Fig. 1(a) shows an optical image of the capacitive electrode conformally laminated onto the skin surface which is used to record EMG signals. Fig. 1(b) shows that the main fabrication process for the stretchable electrode includes four steps. (1) Fabricated by MEMS techniques on a carrier wafer. (2) Transferred to the PDMS substrate. (3) Delaminated from the glass substrate. (4) Directly printed onto the skin surface. Fig. 1(b(I)) depicts the sEMG electrode is fabricated by microelectromechanical system(MEMS) techniques on a carrier wafer with polymethyl methacrylate (PMMA) sacrificial layer. A precursor solution of PI is spin-coated on a carrier wafer with PMMA sacrificial layer. The PI layer is cured on the hotplate at 150 °C for 5 min and baked in inert atmosphere at 250 °C for 2 h. The Au (~300 nm) layer is deposited by sputtering process and patterned by the photolithography. For the encapsulation, another PI layer is spin-coated and baked in inert atmosphere using the same procedures. Then photoresist AZ4620 is spin-coated onto the PI layer and exposed to determine the PMMA/PI patterns. The photoresist AZ4620 is used as a mask to protect the PI and PMMA polymers that are not etched by O2 RIE (reactive ionic etching, Seni Lab, German) for 10 min. The PDMS substrate is used to pick up the sEMG electrode after the PMMA sacrificial layer is dissolved in atone bathing shown in Fig. 1(b(II)). The whole structure (PI/Au/PI) is patterned in stretchable form, released from the carrier Si wafer and transferred to the PDMS substrate. Fig. 1(b(III)) depicts the sEMG electrode is delaminated from the glass substrate. Fig. 1(b(IV)) shows the sEMG electrode is directly printed onto the skin surface. The electrode adheres onto the surface conformally after dissolving the polyvinyl alcohol (PVA) tape in water for sEMG signal recordings.
The electrical performance of the sEMG electrode mainly depends on material selection and geometry layout. The second order serpentine structure is adopted to improve the areal coverage and stretchability compared to the first order serpentine structure. The sEMG electrode with self-similar configuration is designed to monitor the electrophysi ological (EP) signals for satisfying larger stretchability (>30%). The sEMG electrode with ultrathin construction and neutral mechanical plane configuration improves the stretchability and contact behavior.Fig. 1(c) depicts an optical image of the sEMG designed to quantify biological signal measurement with reference, ground, and measurement electrodes with self-similar serpentine structure. Fig. 1(c) depicts the layout of the wavy network with the 2nd order self-similar serpentine electrode consisting of horseshoe building blocks. The node connection of the unit cells in the network electrode forms triangular lattices. The stretchability and areal coverage with the second order self-similar serpentine structure are larger than the first order structure. The line width of electrode is 50 μm. The middle frame of Fig. 1(c) depicts an optical image of the second order self-similar serpentine structure. The right frame of Fig. 1(c) depicts the SEM image of a cross section of the sEMG electrode layers with PMMA/PI (~1.8 μm), Au (~0.3 μm), and PI (~1.2 μm). A capacitive sEMG electrode is designed to measure the EP signals at the electrode/skin interfaces with a PI polymer dielectric layer.

class="figure_img" id="Figure1"/>
Download
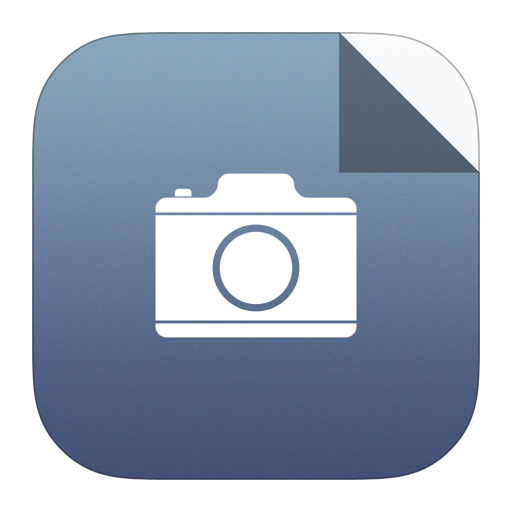
Larger image
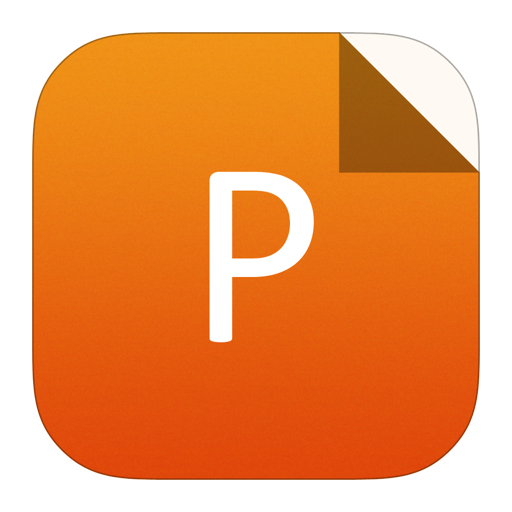
PowerPoint slide
Figure1.
(Color online) Principle and fabrication process of the sEMG electrode with the second order serpentine structure. (a) Photograph of the EMG electrode for signal recordings. (b) Main fabrication process of the EMG electrodes. (c) Optical image of the capacitive EMG electrode with self-similar serpentine structure.
3.
Mechanical and electrical performances
Conformal contact at the electrode/skin interface can improve the accuracy of EP signal measurement. It reduces the motion artifact due to the movements of the skin compared to the conventional biological electrodes. The sEMG electrode with self-similar structure adheres onto the skin surface only by van der Waals interactions[28]. The sEMG electrode mounted in this way exhibits excellent compliance and ability to follow the motion of the skin without constraint or delamination, which satisfies the large deformation of the skin. The total energy of the surface electrode adhered onto the skin consists of bending energy and membrane energy of the sEMG electrode, elastic energy of the skin, and the device-skin interfacial adhesion energy[29, 30]. Figs. 2(a) and 2(b) show the device adhered onto the skin surface in the compressed and stretched form respectively. Fig. 2(c) depicts the sEMG electrode is delaminated from the skin surface for recycling the electrodes. The sEMG electrode is in the twisted form shown in Fig. 2(d). It shows that the sEMG electrode with low bending stiffness (thin, soft electrode/backing layer), smooth and soft skin, and strong adhesion all promote conformal contact[31].

class="figure_img" id="Figure2"/>
Download
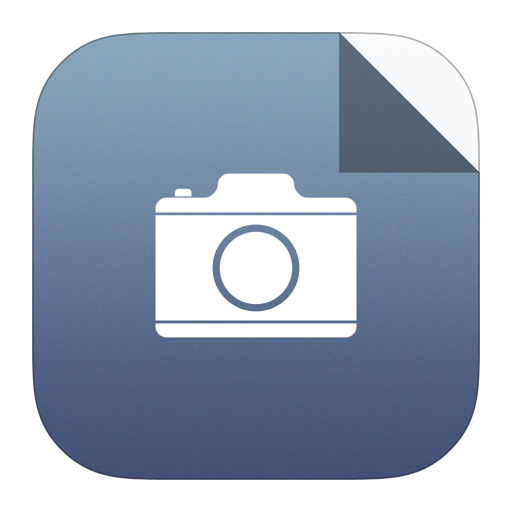
Larger image
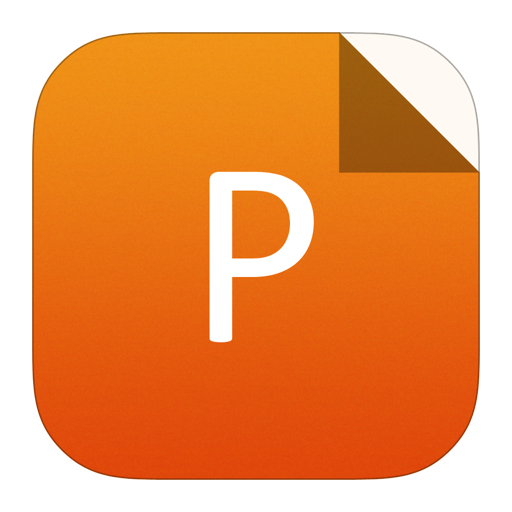
PowerPoint slide
Figure2.
(Color online) Contact behavior at the sEMG electrode-skin interface in: (a) stretched, (b) compressed, (c) delaminated, and (d) twisted forms. The scale bar is 1 cm.
The sEMG electrode with self-similar configuration satisfies the large deformation of the skin surface[32, 33]. The stretchability of the sEMG electrode is evaluated quantitatively by FEM using the commercial package Abaqus 6.10. Fig. 3(a) shows the 40% stretched state of the sEMG electrode by FEM simulation. The optical images of the electrode in the stretched format are shown in Fig. 3(b). Comparing the node parts in Fig. 3(a1) and Fig. 3(b1), the deformation format of the optical image of the electrode is similar to the FEM simulation results. Figs. 3(a2) and 3(b2) show the distribution of maximum principal strain appear at the largest curvature of the microstructure. It is seen that the experimental results agree well with the FEM simulation results, which shows the patterned electrode structure satisfies 40% stretched deformation.

class="figure_img" id="Figure3"/>
Download
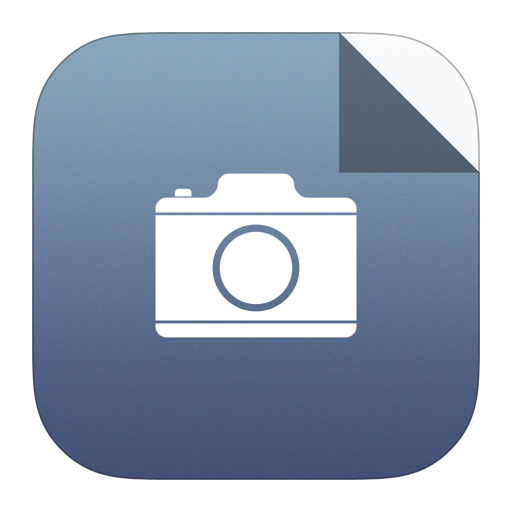
Larger image
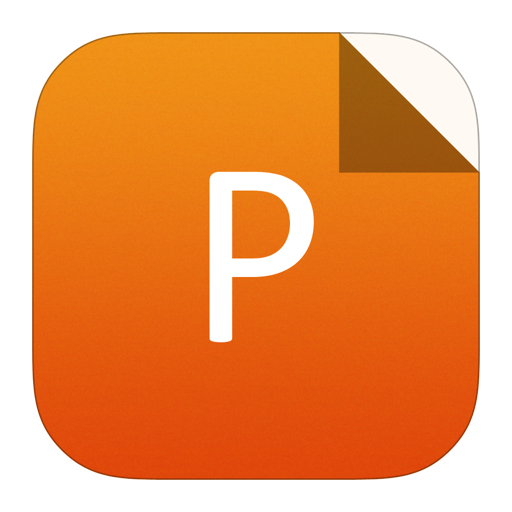
PowerPoint slide
Figure3.
(Color online) (a) Stretchability analysis of the capacitive electrode with triangular lattice up to 40% by FEM, and local graph analysis. (b) Experiment comparison analysis and validated stretchability of the capacitive electrode.
The sEMG electrode with self-similar structure laminated onto the skin surface forms a capacitance with the PI dielectric layer[34]. The stretchable surface electrode reduces the contact impedance as it increases the contact area due to the conformal contact with the skin surface. Capacitive electrodes with PI insulating layers exhibit higher stable gain over the frequency range 20–10 000 Hz, due primarily to their relatively highCE. Fig. 4(a) depicts the skin-electrode interface contact impedance decreases with the increasing scanning frequency with the area 40 mm2. Fig. 4(b) depicts the leakage current from the capacitive electrodes is rather small compared to the conventional medical biological electrodes. It shows that the current is below 0.2 mA when 10 mA passes through the electrodes, which is helpful to protect the soft biological tissues during biological signal monitoring.

class="figure_img" id="Figure4"/>
Download
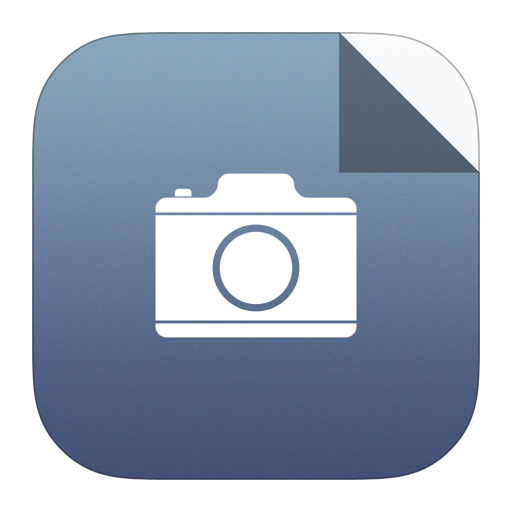
Larger image
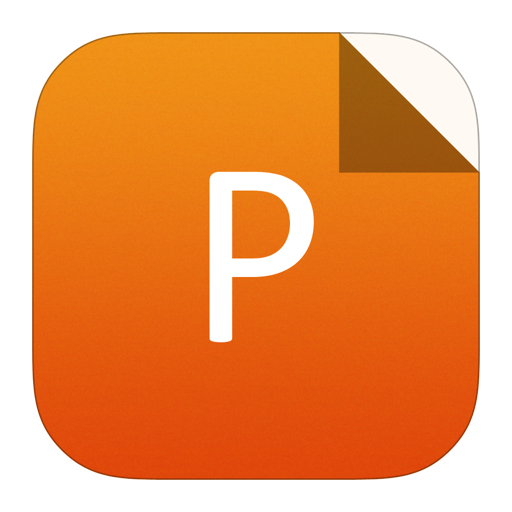
PowerPoint slide
Figure4.
(Color online) Electrical parameters analysis of the capacitive EMG electrode. (a) Contact impedance versus with the scanning frequency. (b) The leakage current at the skin/electrode interface.
4.
Human-machine interaction
4.1
Signal recordings and classification
The electromyography signals are recorded by the stretchable surface electrode adhered onto the skin surface. To minimize the motion artifact during movement, the electrodes should maintain conformal contact with the skin in case an external load is applied to the surface electrode. The electrodes yield analog data, which is converted to digital signals, and are connected to the collecting board card PXI 6289 (National Instruments, USA) with conductive wire. The signal is transmitted to Matlab (The Mathworks Inc., Natick, MA) software on a PC. The classification signal is transmitted to the mobile robot integrated with a wireless ZigBee receiving node via a wireless ZigBee transmitting node, which is connected to the PC via serial port communication.
Fig. 5 shows the physiological signal measurement of the surface electrode from different parts of the human body, such as the wrist, index finger and face with different motion of the body. A capacitive electrode is even wrapped onto the finger, where bending creates high-quality sEMG signals, in spite of the relatively small area of the corresponding muscle. Fig. 5(a) illustrates the stretchable capacitive electrodes for measuring sEMG signals on the forehead. The corresponding sEMG signals from the stretchable capacitive electrode are shown in Fig. 5(b) with frown and blink actions. Fig. 5(c) shows the electrode laminated onto the index finger, as an example of a location where a conventional gel-based electrode might not be acceptable. The corresponding sEMG signals when bending and unbending the finger are shown in Fig. 5(d). The capacitive sEMG electrode laminated onto the surface of the face is shown in Fig. 5(e). Fig. 5(f) depicts clenching the jaw, smiling, and moving the mouth create different types of sEMG signals, each of which is clearly distinguishable from the baseline noise. Fig. 5(g) shows the capacitive electrode placed onto the back of the neck. The corresponding sEMG signals are shown in Fig. 5(h) with nodding and moving to the left actions. The biological signals from these parts of the body are clearly distinguishable from the baseline noise, which as demonstrated in these experiments suggest many possibilities in health monitoring, as well as in HMI technologies based on the sEMG electrode.

class="figure_img" id="Figure5"/>
Download
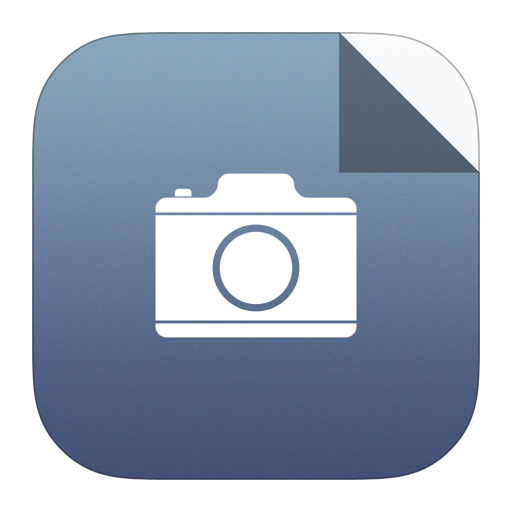
Larger image
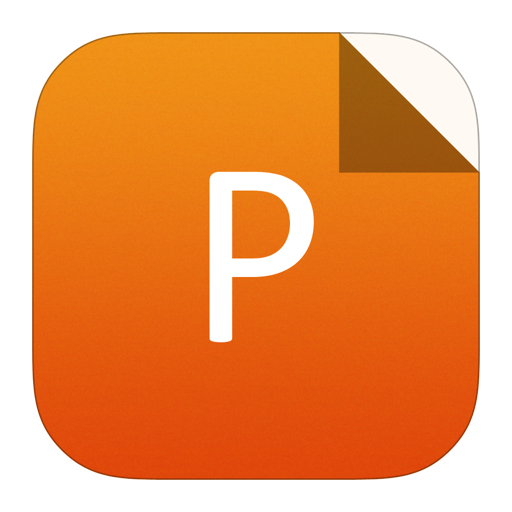
PowerPoint slide
Figure5.
(Color online) Biological signal measurement from different parts of the body with the capacitive EMG electrodes on (a) the forehead; (c) the face; (e) the index finger; (g) the back of the neck; (b), (d), (f) and (h) the EMG signals of corresponding parts of the body. The scale bar is 2 cm.
The versatility in location demonstrated in these experiments suggests many possibilities in health monitoring, as well as HMI technologies based on the sEMG electrode to control the motion of a mobile robot. It would generate different signals with different gestures by the surface electrode placed onto the two wrists. Root mean square (RMS) is used to evaluate the characteristic signal in the time span T, and it is shown as
$${ m RMS} = sqrt {frac{1}{N}sumnolimits_{k = 1}^N {{{left[ {{x_k}} ight]}^2}} } ,$$ ![]() | (1) |
where N means the number of samples, and xk means the k-th signal data in the time span.
The stretchable surface electrode is used to collect the biological signals. The two electrodes are laminated onto the two wrists, respectively. Subjects perform four gestures: 1) Both flip inward, 2) Left wrist flips outward and right flips inward, 3) Left wrist flips inward and right flips outward, and 4) Both flip outward. It generates four signal modes as the four manual gestures. For training purposes, the participants perform each bimanual gesture ten times. A baseline trial corresponds to the participant at rest for four seconds. Extraction and real-time classification uses a T sliding window with ΔT overlap with minimum RMS method. Fig. 6 shows the classification of the sEMG signals collected by the stretchable surface electrode under different combinations of gestures. Fig. 6(a) depicts optical images of four different gestures according to the wrists flipping inward and outward. The corresponding electrophysiological signals are recorded from the four bimanual gestures in Fig. 6(b). The two wrists flip inward and outward generate four gesture combinations, that is, four kinds of electrophysiological signal combinations, expressed as a set of Ω = {I, II, III, IV}. “I” represents both the left and right wrists flipping inward at the same time; “II” means left wrist outward, right inward; “III” means left wrist inward, right wrist outward; “IV” means two wrists flip outward simultaneously.
The characteristic signals of the different gestures are computed by Eq. (1) in the collecting time period T. The average RMS value
m{RMSI}}} $

m{RMSI}}} $

m{RMSO}}} $

m{RMSO}}} $

4.2
Motion control of the robot via HMI
Fig. 7 shows the human machine interaction algorithm for the motion control of the mobile robot via the surface electrode laminated onto the wrists based on the classification results (Fig. 6). The main steps are as follows:

class="figure_img" id="Figure6"/>
Download
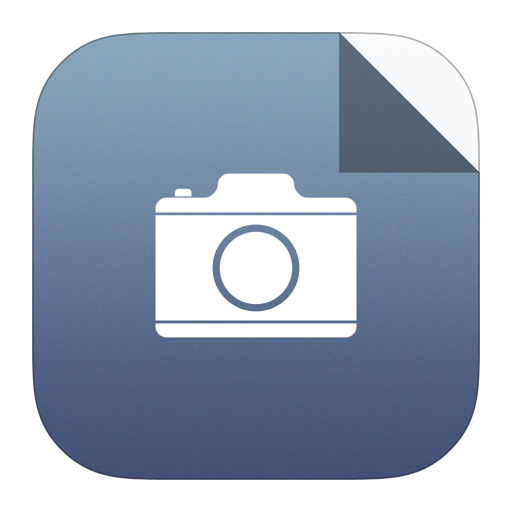
Larger image
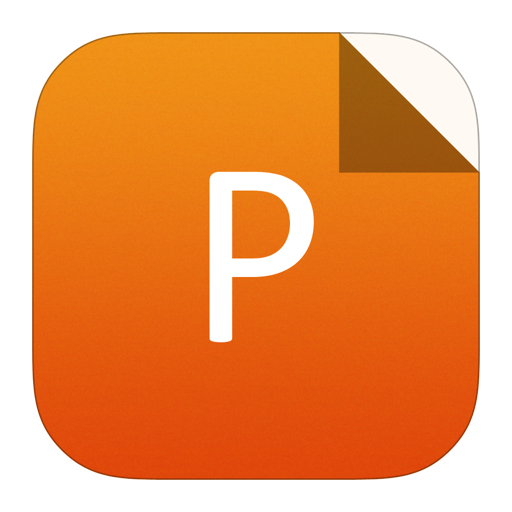
PowerPoint slide
Figure6.
(Color online) Classification of the biological signals with different actions.

class="figure_img" id="Figure7"/>
Download
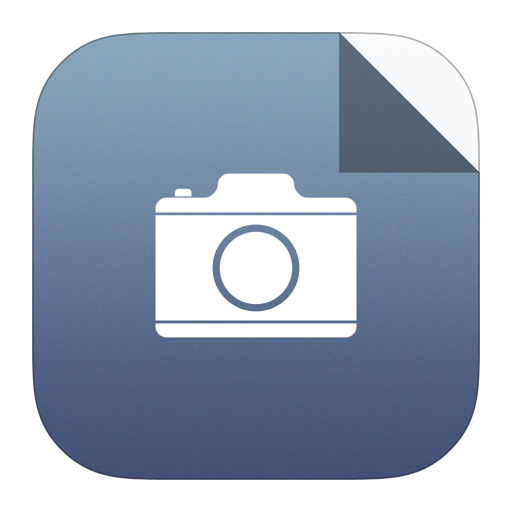
Larger image
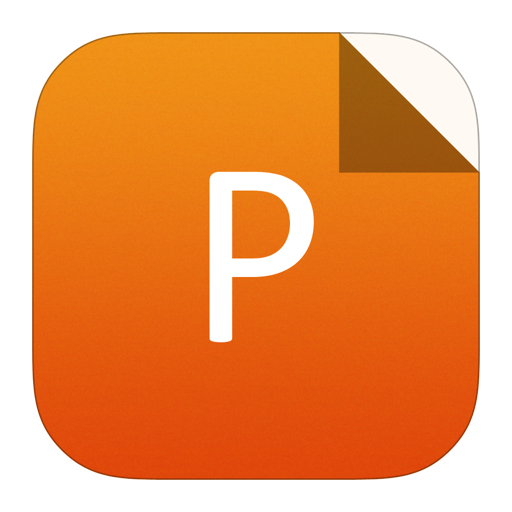
PowerPoint slide
Figure7.
(Color online) Flow chart of HMI to control the motion of the mobile robot.
Step 1: The sEMG signals are recorded as X(t), Y(t) from the stretchable surface electrode laminated onto the wrists.
Step 2: At time point ti, sliding time window method (the length of the window is T) is used to extract the EMG signal, and it is represented as the time series x(ti)~ x(ti+T) and y(ti)~y(ti+T) from the two wrists.
Step 3: At the time interval ti~ti+T, the RSM value is computed using Eq.(1) as the characteristic signal RMSX(ti) and RMSY(ti) for the left and right wrists.
Step 4: Comparing the characteristic signal RMSX(ti) and RMSY(ti) with the interval Ξ1 and Ξ2, if RMSX(ti) and RMSY(ti) belong to the intervals Ξ1 or Ξ2, the identifier FlagX and FlagY would assign to 0 or 1. If they do not belong to the intervals, FlagX and FlagY would assign to NULL.
Step 5: According to the EMG signals and identifiers (FlagX, FlagY) combination (11, 10, 01, 00), the control commands are generated to control the motion of the mobile robot (move forward, turn left, turn right and stop).
Step 6: The time moves forward ΔT, and goes to the next loop. Until ti + T > Ttotal, the algorithm ends.
The experiment platform for HMI to control the mobile robot includes two electrodes laminated onto the wrist, AD collecting unit, data process unit, wireless data transmitting unit and the mobile robot integrated with wireless ZigBee node. The mobile robot is controlled by receiving the signals from the stretchable electrodes. Fig. 8 shows experiment results of the motion control of the mobile robot by the sEMG signals from the wrists. Insert graphs show the control gestures. The mobile robot is successfully controlled by these gestures. Fig. 8(a) shows the mobile robot moving forward when receiving control command “I” with the two wrists flipping inward (the inset graph). Figs. 8(b)–8(f) show that the mobile robot can execute the actions (turn left, forward, turn right, forward and stop) with the corresponding control commands by the control gestures.

class="figure_img" id="Figure8"/>
Download
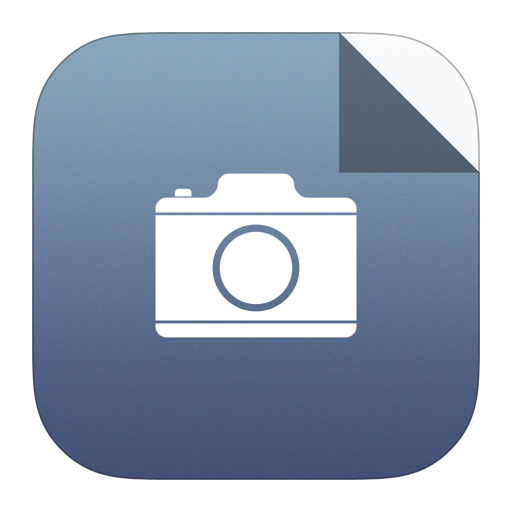
Larger image
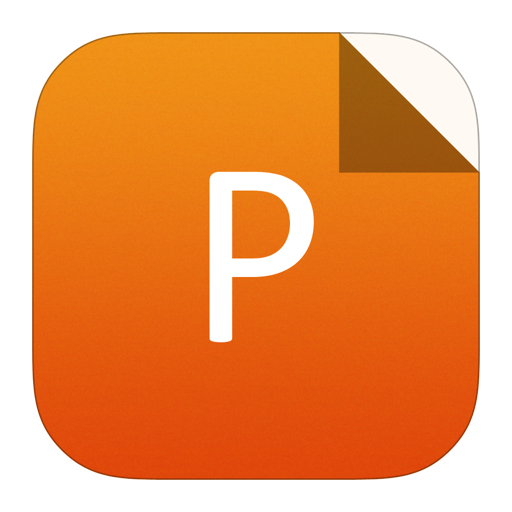
PowerPoint slide
Figure8.
(Color online) Optical images of the mobile robot controlled by the human gestures via sEMG electrodes.
5.
Conclusion
The work illustrates advantages in HMI based on the stretchable surface electrode with self-similar serpentine structure, which could satisfy larger deformation (>30%) validated by FEM simulation and experiments. The electrodes offer enhanced levels of wearability, expanded options in electrodes sterilization and reuse, and minimized artifacts from body motions compared to previously reported technologies. The sEMG electrode with thin, soft substrate provides conformal contact with the skin surface via van del Waal interaction alone, and it reduced the motion artifacts caused by the motion of the skin. Exploring the HMI capabilities into the capacitive sEMG electrode represents promising directions in future research.
Acknowledges
The authors would like to thank the Comprehensive Experiment Center for Advanced Manufacturing and Equipment Technology in HUST and Micro-nano Manufacturing Technology Platform in Wuhan National Laboratory for Optoelectronics.