全文HTML
--> --> -->连续波腔衰荡光谱(CW-CRDS)采用窄线宽的连续激光器, 并通过压电陶瓷扫描腔长[7—9], 最初由Romanni提出[2], 其特点在于采用了腔长扫描方式, 光谱间隔可任意长, 非常适合低压光谱(如0.1—0.3个大气压)的精细结构及其光谱参数(如碰撞展宽系数[10]、Dicke收敛系数[11]和速度依赖的碰撞展宽系数[12])的测量. 但腔长扫描时, 任意波长的激光均可耦合进腔内, 激光波长的不稳定性会直接影响衰荡时间的精确测量, 尤其在吸收谱线两翼斜率较大位置处, 激光波长轻微波动即可导致衰荡时间的较大波动, 进而降低光谱信噪比, 且这种激光波长导致的光谱噪声难以通过多次平均的方式消除[13—17]. 如Tan等[13]采用掺钛蓝宝石激光器测量极低压下17O富集的H2O谱线时, 激光波长波动噪声对谱线两翼的干扰非常显著; Kassi等[14]采用分布反馈激光器测量低压下CO2光谱时也出现了类似现象; Bicer等[17]采用分布反馈激光器测量大气压下的CH4和H2O光谱时, 该噪声虽有所减弱, 但仍大于其他类型的噪声. 针对激光波长波动问题, 目前多采用激光稳频技术, 如Pound-Drever-Hall锁频[18,19]技术, 该技术可将激光的频率稳定性提高1—2个数量级; 光频梳作为当前的研究热点[20—22], 可实现低于几兆赫兹的激光绝对频率的精确测量. 不过上述方法均需要较高的成本, 且系统复杂. 另外, CW-CRDS需要昂贵的波长计来实时测量激光频率, 不仅操作复杂且会带来仪器噪声[13—17].
在本文结合波长扫描[23]与傅里叶变换思想, 提出了一种简单、易操作的基于傅里叶变换和快速波长扫描的CRDS方法(FTS-CRDS), 该方法通过快速波长扫描, 得到周期性的衰荡时间, 然后对其进行傅里叶变换, 提取其特征频谱以复现气体吸收光谱. FTS-CRDS不需复杂的锁频技术, 也不需用波长计实时测量激光绝对波长, 可有效减小激光波长波动等噪声. 随后利用该方法对CO分子较弱吸收谱线R(5) 6371.299 cm–1和R(6) 6374.406 cm–1进行了测量, 得到了该谱线在背景气N2下的碰撞展宽系数、Dicke收敛系数和速度依赖的碰撞展宽系数. 实验结果表明, 与传统的CW-CRDS相比, FTS-CRDS可有效减小激光波长波动等噪声, 提高光谱信噪比, 可实现光谱参数的高精度测量.
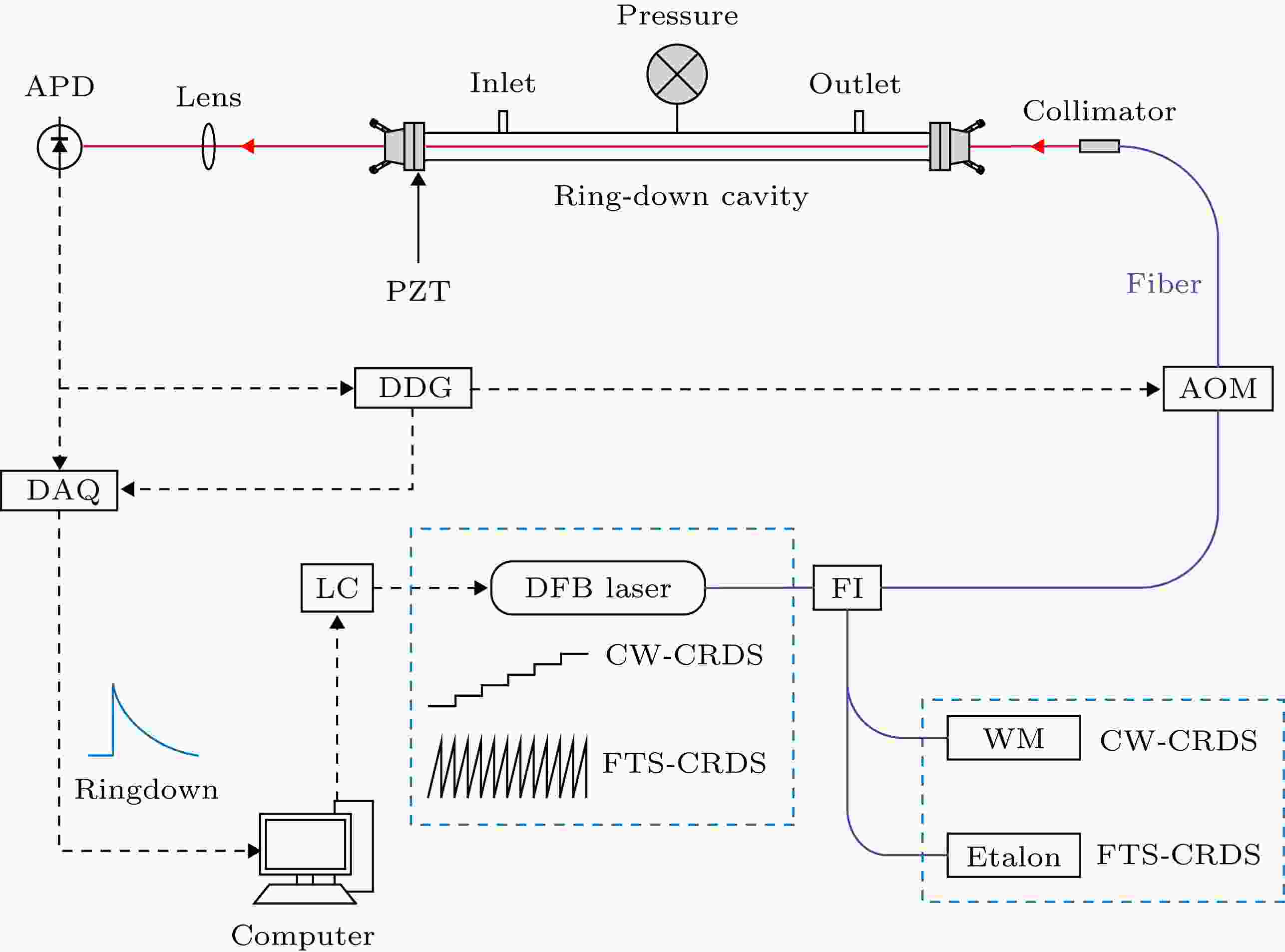
Figure1. The system schematic diagram of FTS-CRDS and CW-CRDS. LC, laser current and temperature controller; FI, fiber isolator; AOM, acousto-optic modulator; APD, avalanche photodiode; DDG, digital delay generator; PZT, piezoelectric transducer; WM, wavelength meter; DAQ, data acquisition system; WM, wavelength meter.
图1中蓝色虚线框以外的部分由两种方法共享, 均以分布反馈半导体激光器为光源, 激光线宽约2 MHz. 为了减少对激光器的光反馈, 让激光束通过光隔离器传输, 声光调制器关断时间为40 ns. 光学腔(长度为50 cm)由一对高反射率(反射率约0.999975)镜片组成. 实验中通过PZT扫描腔长, 使腔长扫描范围大于一个自由光谱范围, 确保任意波长的激光均可耦合进腔内. 腔另一端的出射光通过透镜收集后由光电探测器接收, 当探测器达到预设触发电平时, 由数字延迟发生器向射频源发送脉冲信号, 使声光调制器失去能量, 从而关闭进入腔内的激光以形成单指数衰减信号. 采用高速数据采集卡同时采集脉冲信号和单指数衰减信号, 采集卡采样频率为20 MHz, 并利用LabVIEW程序对实验数据实时处理, 快速拟合[24,25]得到衰荡时间.
激光电流(图2(a)和图2(b)中蓝色曲线)周期性扫描, 使得激光波长可扫描分子整条吸收谱线, 同时高速扫描腔长以保证衰荡信号的连续采集, 由于腔内气体分子吸收导致的损耗会随激光波长变化而周期性改变, 因此衰荡时间也随之改变, 从而得到蕴含气体吸收信息的周期性衰荡时间, 如图2(a)中黑色曲线所示. 与此同时, 采用法布里-珀罗(Fabry-Parot, F-P)标准具标定激光相对波长, 测量信号如图2(b)中红色曲线所示, 其中黑色曲线为拟合得到的激光相对波长(本文采用激光器的波长与电流呈负相关). 随后, 对图2(a)中黑色曲线所示的周期性衰荡时间信号进行傅里叶变换, 并从频域上滤除激光波长波动等噪声, 其频谱如图3所示. 最后, 通过提取特征频谱以复现气体吸收光谱. 该方法在测量方式上采用了波长连续扫描, 可有效减小波长波动等影响, 且无需波长计实时测量激光绝对波长, 可避免波长计引入的仪器噪声.
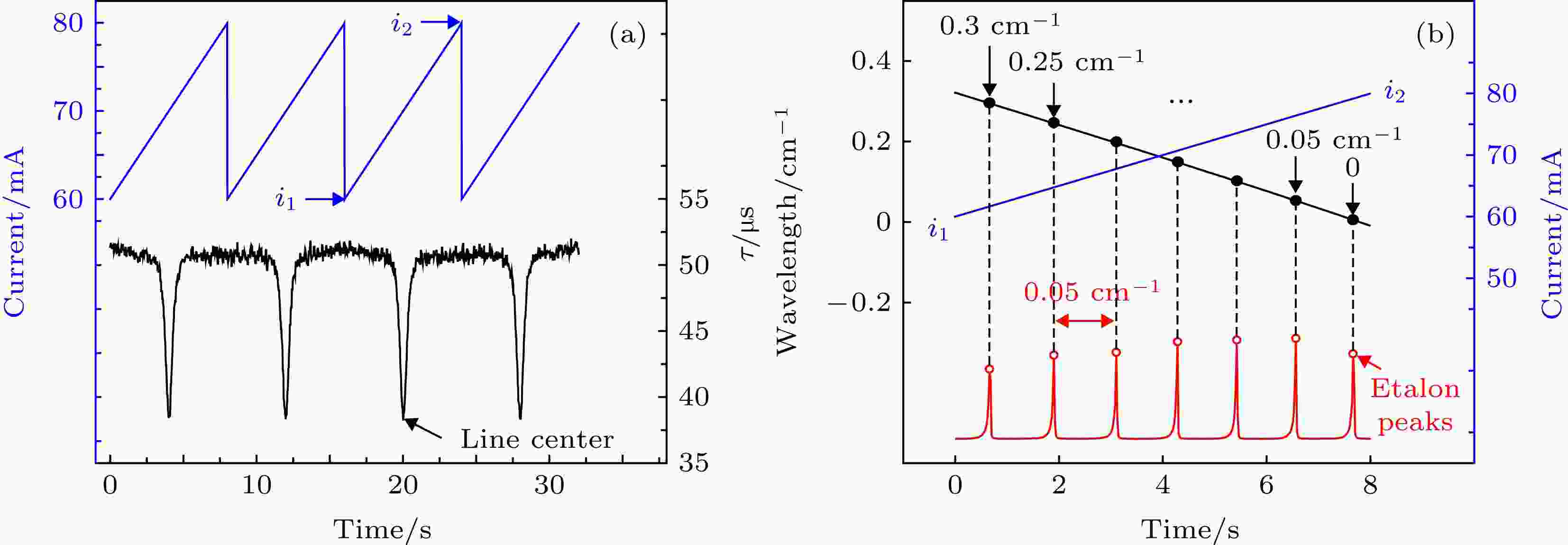
Figure2. (a) Instantaneous laser current (blue) and instantaneous ring-down time (black), τ(t); (b) wavelength (red) and etalon signal (black). Note that the (a) and (b) figure shows an example with only 4 of 100 the circles.
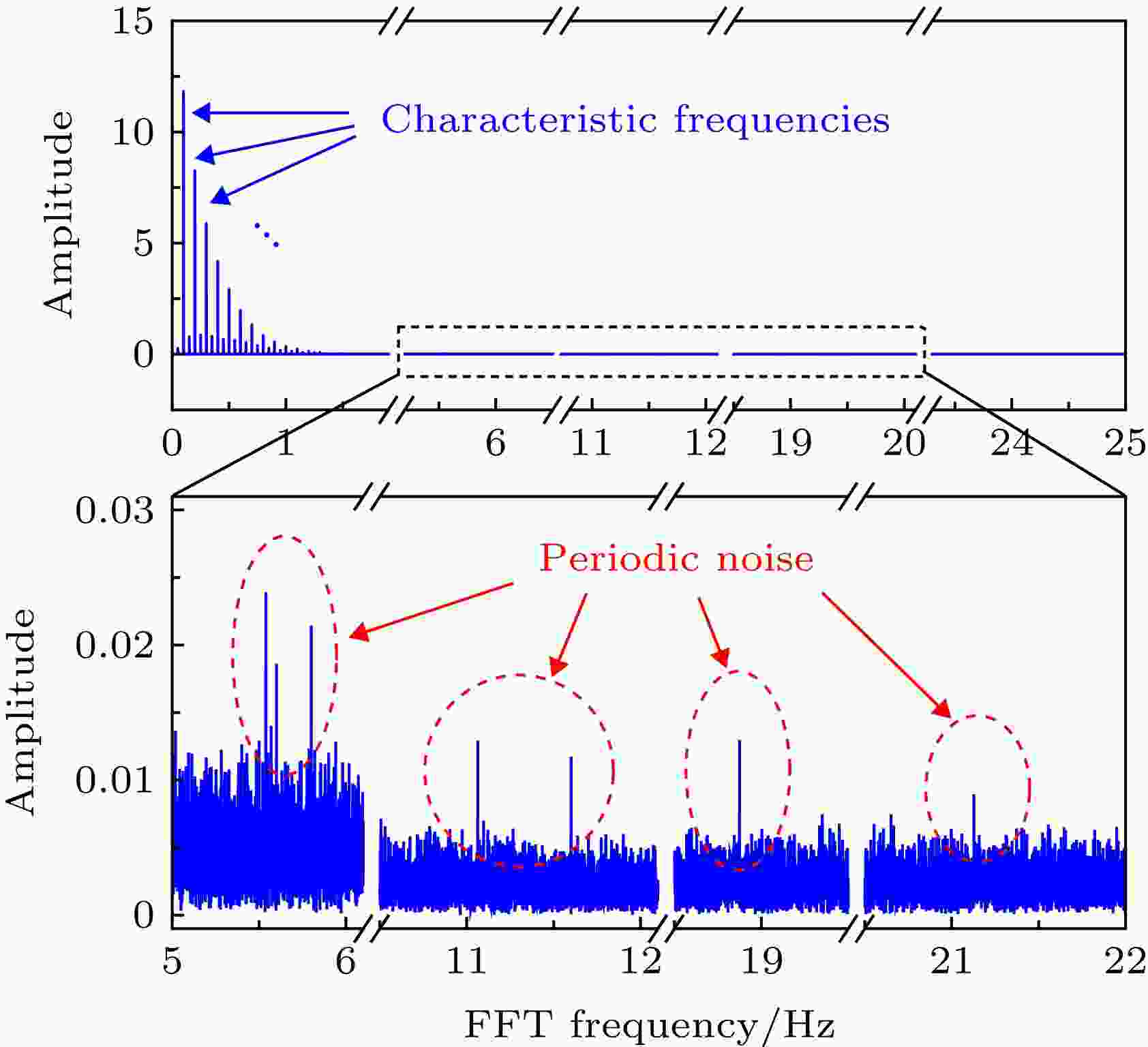
Figure3. The amplitude spectrum of τ(t) and the periodic noises (~5.5, ~11, ~19 and ~21 Hz).
FTS-CRDS方法对衰荡信号的获取和处理详细步聚如下.
首先, 利用LabVIEW控制激光器电流, 使激光器电流连续扫描分子吸收谱线, 扫描频率f为1/8 Hz, PZT的扫描频率(如100 Hz)远高于激光波长的扫描频率, 以确保每个电流点都能捕获到衰荡信号, 同时对衰荡信号进行拟合得到相应的衰减常数. 通过上述连续扫描过程, 即可得到蕴含气体吸收信息的周期性的衰减常数, τ(t), 如图2(a)中黑色曲线所示. 随后对τ(t)进行快速傅里叶变换, 提取特征频率(kf)的傅里叶系数Ak(实部)和Bk(虚部), 将其代入下式可得到重构的衰减常数:
其次, 利用F-P标准具获取激光波长与扫描时间的关系, 如图2(b)所示, 选取任意一个锯齿波周期, 令电流最低点对应的激光相对波长为v1 (本文采用激光器的波长与电流呈负相关), 波长从v1变化到v2时, 对应的扫描时间t'从0变化到T (T = 1/f ). 考虑到激光扫描时波长非线性不可忽略, 采用下述多项式对激光相对波长进行拟合:
最后, 将时间t'代入(1)式得到单个锯齿波周期对应的衰减常数:
在CRDS中, 吸收系数与衰减常数存在如下关系[13—17]:
4.1.CO光谱实验结果分析
实验中采用CW-CRDS和FTS-CRDS方法分别对CO分子的6374.406 cm–1谱线进行了测量, 气体温度、压力和CO浓度分别为288 K, 18 kPa和0.1%(背景气N2). 在CW-CRDS方法中, 激光电流从70 mA以50 μA的间隔步进至90 mA, 在每个电流点测量200次, 总测量点数为8 × 104个, 用时约27 min, 测得的衰荡时间如图4(a)所示. 相应地, FTS-CRDS采集了200个锯齿波周期, 其扫描频率为1/8 Hz, 每个周期400点, 电流扫描幅度(70—90 mA)、总点数和时间与CW-CRDS相同, 测量结果如图4(b)所示. 与图4(a)相比, 图4(b)谱线中心两侧(蓝色与绿色区域)衰荡时间波动较小, 测量数据整体更加平滑. 由于FTS-CRDS采用快速波长扫描的方式, 测量中不需固定激光电流, 对激光电流和温度的稳定性依赖较小, 因此可有效减小激光波长波动等影响.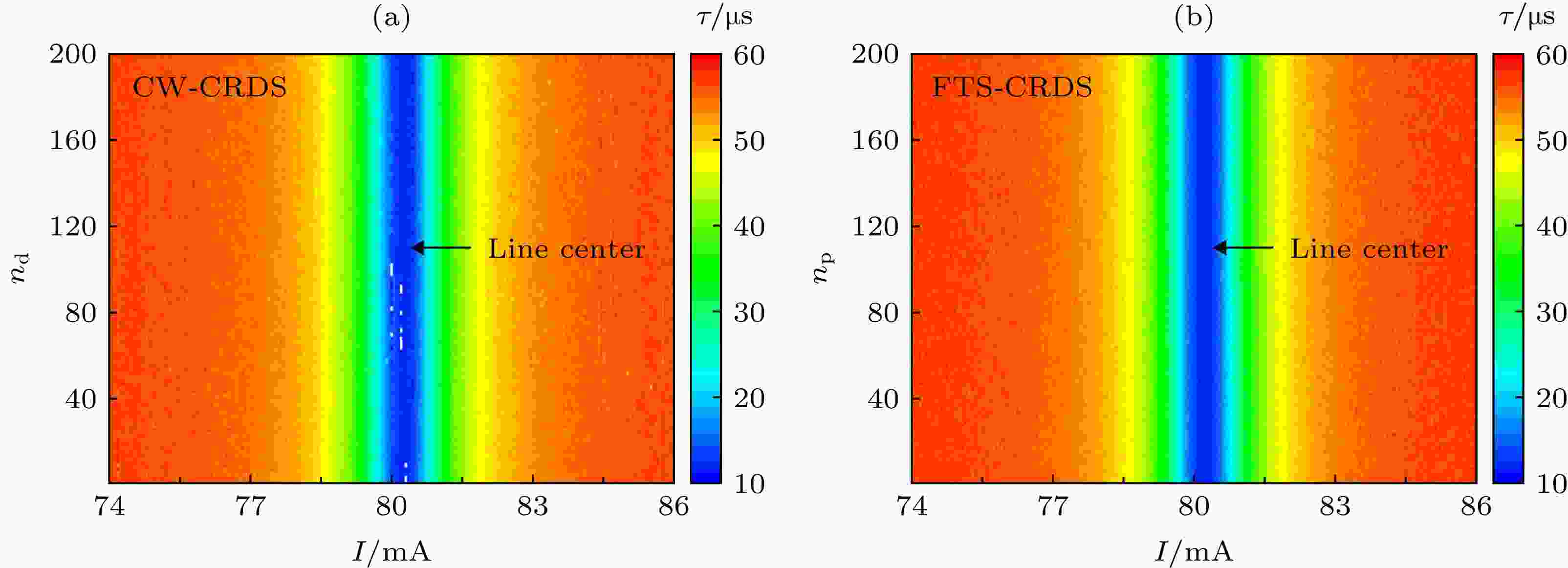
Figure4. (a) Ring-down time measured by CW-CRDS, nd is the number of measurements per current, white points represent out-of-range data; (b) ring-down time measured by FTS-CRDS, np is the number of cycles; the x axis represents laser current with the range of 74?86 mA.
图5(a)为CW-CRDS方法测得的吸收系数, 以及采用Voigt线型(VP)和Galatry线型(GP)拟合得到的最优结果. 从VP拟合结果可知, 残差中存在“w”形的精细结构, 其大小约为峰值吸收的1.7%, 其原因在于VP线型未考虑Dicke收敛效应或者速度依赖的碰撞展宽效应[11,12], 这种精细结构也验证了本文CW-CRDS测量结果的可靠性. 相比之下, GP线型考虑了Dicke收敛效应, 拟合时可消除Dicke收敛导致的“w”形的残差. 从GP拟合残差可知, 谱线中心两侧斜率较大位置存在较大的噪声, 其幅度最大约为4 × 10–8 cm–1, 相当于峰值吸收的1.6%, 这与Kassi等[14] (1.6%), Mondelain等[15](1.4%)测得的结果一致, 也即验证了此噪声主要来源于激光波长波动.
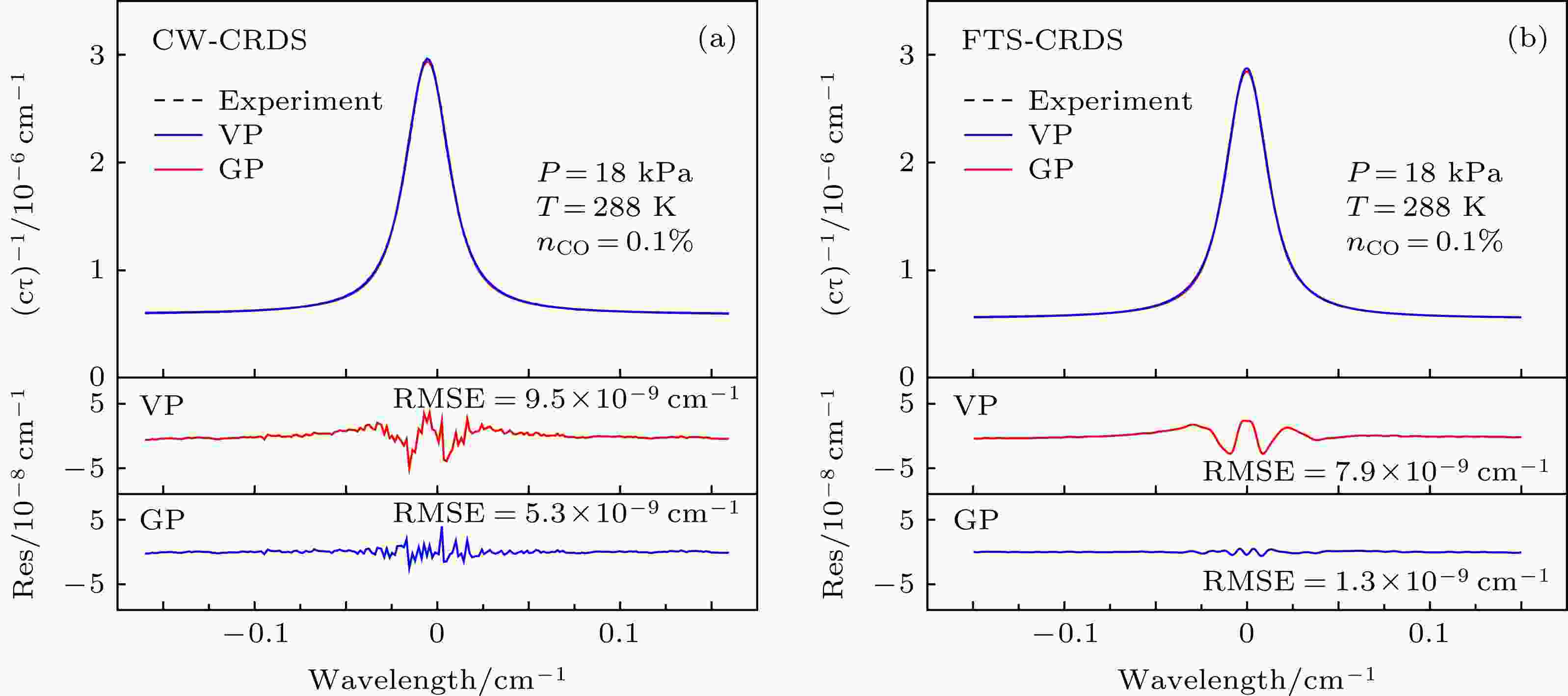
Figure5. The absorption spectra (black dotted line) of CO measured by the two methods and the best fit of Voigt profile (red) and Galatry profile (blue): (a) CW-CRDS; (b) FTS-CRDS; the x and y-axes scales of the residuals obtained by the two methods are the same
图5(b)为FTS-CRDS方法测得的气体吸收系数, 以及采用VP和GP的最优拟合结果. 在FTS-CRDS测量中, 首先利用F-P标准具测量激光相对波长, 计算得到多项式系数(a2 = 1.35 × 10–5, a1 = 0.012, a0 = 0.197); 其次, 对测得的200个周期衰减常数τ(t)进行傅里叶变换, 提取其特征频率的傅里叶系数并代入(3)式; 再次, 将多项式系数代入(2)式可得到对应的相对波长, 联立(2)和(3)式即可重构出衰减常数; 最后, 将重构的衰减常数代入(4)式即可得到气体吸收系数. 与图5(a)相比, 图5(b)中VP拟合残差w形精细结构更清晰, 且GP拟合残差在谱线两翼处波动较小, 谱线两翼噪声幅值仅约为峰值吸收的0.4%左右. 事实上, FTS-CRDS通过提取周期性衰减常数的整数倍特征频谱来复现气体吸收光谱, 因此可有效减小或消除激光波长动等噪声, 另外, 该方法无需采用波长计实时测量激光绝对波长, 可避免波长计引入的仪器噪声. 由图5实验结果可知, 两种测量方法的均方根误差(RMSE)相差4倍以上, 即FTS-CRDS方法将光谱信噪比提高了约4倍, 适合谱线参数的高精度测量.
2
4.2.CW-CRDS与FTS-CRDS光谱参数测量结果
为了验证FTS-CRDS测量精度, 利用该方法对不同压力下CO分子6374.406 cm–1光谱参数进行了测量, 并与CW-CRDS测量结果进行对比, 其中, 碰撞展宽系数(γc)及速度依赖的碰撞展宽系数(γ2)采用SDVP线型[12]拟合得到, Dicke收敛系数(β)采用GP及Rautian线型(RP)[11]拟合得到. 实验中, 气体温度为288 K, CO浓度为0.1%(背景气为N2), 压力范围1—20 kPa, 每个压力测量点温度波动小于0.1 K. 图6为两种方法的测量结果, 其中β和γ2数值远小于碰撞展宽系数γc, 测量时对实验光谱信噪比有较高的要求. 由于FTS-CRDS方法测得的光谱信噪比提高了4倍以上, 因此可准确获得γc, β和γ2系数, 图6(a)和图6(b)拟合线性度分别达到0.9999和0.99, 与CW-CRDS相比, FTS-CRDS方法测得的光谱参数具有更小的测量不确定度.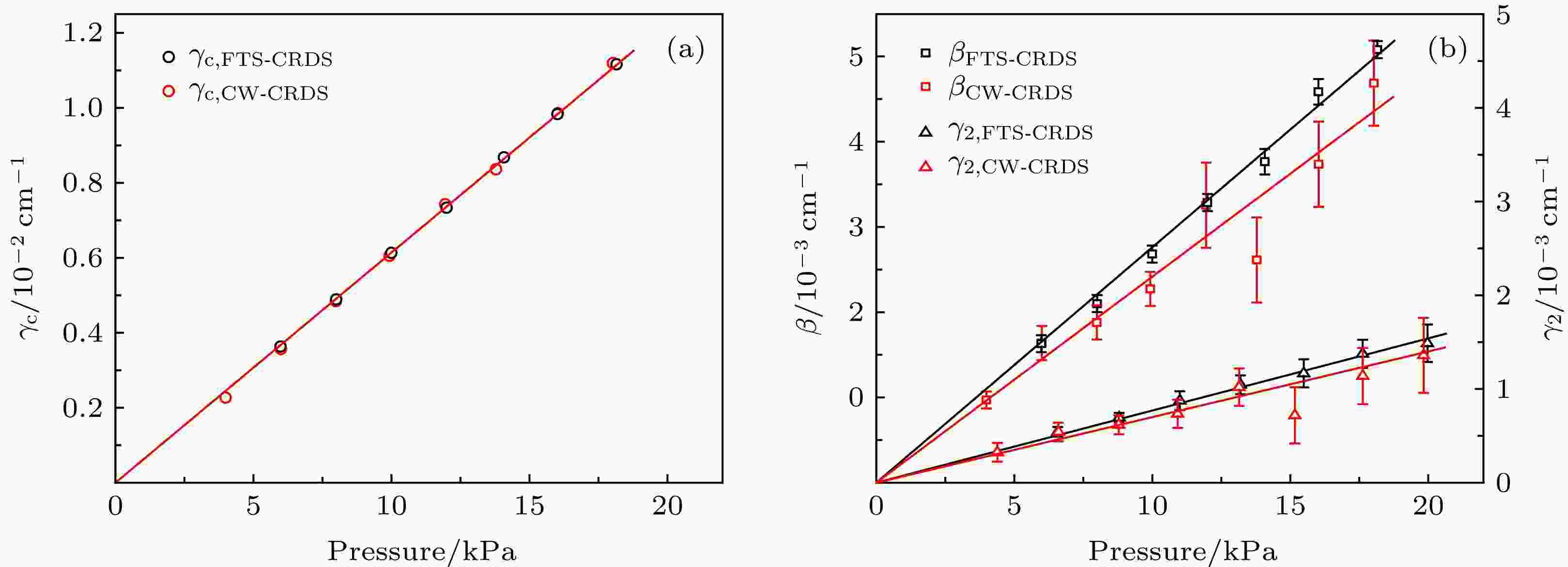
Figure6. The measured spectral parameters for various pressures (CW-CRDS (red), FTS-CRDS (black): (a) γc (hollow triangle); (b) β (hollow square), γ2 (hollow triangle).
表1为两种方法测得的CO分子6371.299 cm–1和6374.406 cm–1谱线的光谱参数, 两种方法采用四种线型拟合的碰撞展宽系数γc(T0)相差均不超过1%, 这表明两者测量结果吻合较好. 表1中所列的光谱参数测量不确定度主要来源于气体温度 (<0.1 K)、压力(<10 Pa)、浓度(<50 ppm)以及光谱拟合误差, 其中采用VP拟合得到碰撞展宽系数的相对不确定度约为0.4%, GP/RP/SDVP拟合的相对不确定度为0.1%, 因而计算得到CW-CRDS所测得的γc (T0)总的不确定度范围约为0—1%, FTS-CRDS约为0—0.9%. 与γc (T0)相比, β (T0)和γ2 (T0)对测量光谱的信噪比有更高的要求, CW-CRDS方法由于测得的光谱信噪比较低, 其测量不确定度范围约为15%—30%. 与此相比, FTS-CRDS由于采用了波长扫描以及傅里叶滤波, 减小了谱线两翼处等噪声, 有效提高了光谱信噪比, 其测得的β (T0)和γ2 (T0)不确定度范围约为5%—15%, 与文献[28,29]采用稳频CRDS的测量结果0.7%—14%相接近.
v0/cm–1 | E''/cm–1 | Transition | ? | γc (T0)/10–2 cm–1·atm–1 | β (T0)/10–2 cm–1·atm–1 | γ2 (T0)/10–2 cm–1·atm–1 | ||||||
CW | FTS | HT | CW | FTS | CW | FTS | ||||||
6371.299 | 57.670 | R(5) | VP | 6.26b | 6.29b | 6.29a | — | — | — | — | ||
GP | 6.43b | 6.51b | 2.84d | 2.92c | — | — | ||||||
RP | 6.47b | 6.54b | 2.57d | 2.60c | — | — | ||||||
SDVP | 6.50b | 6.59b | — | — | 0.87d | 0.85c | ||||||
6374.406 | 80.735 | R(6) | VP | 6.10b | 6.11b | 6.12a | — | — | — | — | ||
GP | 6.20b | 6.25b | 2.65d | 2.77c | — | — | ||||||
RP | 6.25b | 6.29b | 2.38d | 2.39c | — | — | ||||||
SDVP | 6.26b | 6.33b | — | — | 0.69d | 0.79c | ||||||
注: γc, 碰撞展宽系数; β, Dicke收敛系数; γ2, 速度依赖的碰撞展宽系数; FTS和CW分别代表FTS-CRDS和CW-CRDS, HT表示HITRAN; a表示相同温度(T = 288 K)下HITRAN[30]的数据, 空气为背景气; b不确定度 0%—1%; c不确定度 5%—15%; d不确定度 15%—30%. |
表1FTS-CRDS和CW-CRDS测量的光谱参数及其不确定度
Table1.Measured spectral parameters and uncertainties by CW-CRDS and FTS-CRDS.