1.
Introduction
The excessive development of non-renewable resources and the aggravation of ecological pollution make the rational development and utilization of solar energy seem particularly important[1-4]. Photoelectrochemical (PEC) water splitting technology can decompose water into clean hydrogen and oxygen with the assistance of sunlight, promote the development and utilization of renewable energy, and has broad prospects in the field of energy conversion and application in the future[5-9]. However, due to the high charge recombination probability and low light absorption efficiency, the presently reported photocurrents are far from the practical requirements[10, 11]. As a special semiconductor, ferroelectric materials have attracted much attention due to the internal polarized electric field that can promote electron–hole separation and reduce carrier recombination[12-15]. Meanwhile, owing to the abnormal photovoltaic effect of ferroelectric materials[16, 17], the photovoltaic voltage will not be limited by the energy gap (Eg) of crystals[18], which theoretically has a high charge separation efficiency.
Recently, as a typical ferroelectric material, BiFeO3 (BFO) has attracted a lot of interest owing to its robust ferroelectricity, relatively small band gap (~2.3 eV), and strong absorption of visible light[19-22]. Although BFO has often been used in PEC water splitting systems, the overall PEC activity of BFO is still too low for industrial applications because of inherent defects in the material itself. Effective charge separation is essential for enhancing PEC activity of photoelectrodes[23, 24]. Although many strategies have been used to modify BFO to facilitate the separation of photoelectron–hole pairs[7, 13, 19], strategies for modifying BFO photoelectrodes using Au buffer layers have not been reported. In our previous studies, we found that an Au buffer layer can improve the crystalline quality of the film, enhance the conductivity of the material, and make it easier to separate electrons and holes[25, 26]. In addition, compared with other methods, such as forming heterojunction with other semiconductors or using catalysts, the strategy of Au buffer layer modification is simple and more feasible.
Herein, we deposited Au buffer layer on FTO glass as a substrate to prepare an efficient FTO/Au/BFO photoelectrode. The effect of Au buffer layer on BFO performance was systematically studied, and the Au/BFO photoelectrode exhibited an extraordinary improvement in PEC water splitting due to the improvement of charge separation efficiency under light irradiation. More importantly, the ferroelectric polarization electric field (Ep) enables the electron–hole pairs in the photoelectrode to be more effectively separated and they also reduce carrier recombination. The PEC performance of Au/BFO photoelectrode was further improved by applying a polarized electric field. Therefore, this research provides a new idea to explore and enhance the PEC performance of ferroelectric films.
2.
Experiments
2.1
Materials and chemicals
The chemical reagents used in this experiment were all purchased from Sinopharm Chemical Reagents Co., Ltd., with analytical purity, which can be used directly.
2.2
Preparation of FTO/Au/BFO photoelectrode
In this work, FTO/Au/BFO photoelectric electrodes were prepared by magnetron sputtering and sol-gel method. BFO precursor solution was prepared according to the method reported previously[23]. Simply put, Fe(NO3)3·9H2O (0.2 M), Bi(NO3)3·5H2O (0.2 M) and citric acid monohydrate (0.4 M) were, respectively, dissolved in ethylene glycol methyl ether and continuously stirred by magnetic agitation for 1 h (Supporting Information). Au films were deposited on clean FTO conductive glass by DC magnetron sputtering (Supporting Information). Then, the BFO sol was dropped on the FTO/Au substrate and the BFO films were deposited in a spin coater (further details can be found in the Supporting Information).
2.3
PEC measurements
The PEC characteristics of the sample were tested using a three-electrode system electrochemical workstation. Among them, the pair electrode was Pt sheet, Ag/AgCl as the reference electrode, and the sample was used as working electrode (further details can be found in the Supporting Information).
2.4
Characterizations
The X-ray diffraction (XRD) spectrum was used to study the crystal structure of the samples (Bruker D8). A field emission scanning electron microscopy (JEOL, 7800F) was used to study the surface morphology of photoelectrode. UV–Vis spectrophotometer (SHIMADZU, UV2600) was used to characterize the light absorption of the films.
3.
Results and discussion
In this experiment, BFO films were prepared on FTO and FTO/Au substrates by a simple sol-gel method. We observed the morphology of the samples with a scanning electron microscope (SEM), as shown in Fig. 1. In Fig. 1(a), the obtained BFO films show an irregular porous structure on a uniform surface. Obviously, FTO/Au/BFO images still show porous structures, but the density of surface defects on the surface of the sample was significantly reduced (Fig. 1(b)). Through SEM image analysis, we believe that the existence of the Au film was the key factor to improve the crystallization of BFO film. In addition, there is a clear interface between BFO and FTO in the cross-section image of the Au/BFO sample (see Supporting Information, Fig. S1), but the Au buffer layer was too thin to be observed in the image.

class="figure_img" id="Figure1"/>
Download
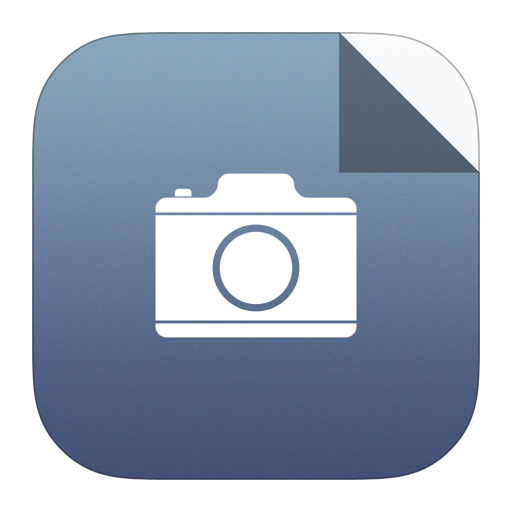
Larger image
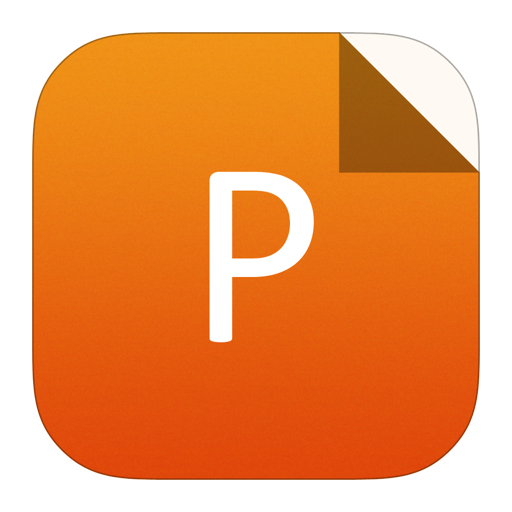
PowerPoint slide
Figure1.
(Color online) SEM images of (a) BFO and (b) Au/BFO. (c) XRD spectra of BFO and Au/BFO. (d) XPS spectra of BFO.
The crystal structures of Au/BFO and BFO were revealed by XRD patterns in Fig. 1(c). The BFO films presents a polycrystalline structure, and the location of characteristic peaks on each crystal plane is consistent with the data of PDF standard card reported previously (No. 72-2112). No diffraction peaks of other impurities were found, which indicates that the preparation of BFO films was successful. Compared to FTO/BFO, the characteristic peak of Au appears in the diffraction peak of FTO/Au/BFO, which undoubtedly confirms the existence of Au[25]. The characteristic peak of Au was relatively weak, which may be caused by the ultra-thin buffer layer of Au. Meanwhile, the diffraction peak of BFO did not change, which indicates that the introduction of Au buffer layer will not destroy the crystal structure of BFO. In addition to studying the crystal structure, the chemical composition of the prepared BFO films was characterized by XPS. Fig. 1(d) confirms that the BFO films consists of the elements O, Fe and Bi[27-29]. The presence of the doublet Bi 4f peaks centered at around 158.6 and 163.9 eV, which confirms the existence of bismuth in the form of Bi3+ (see Supporting Information, Fig. S2(a)). The Fe 2p peaks observed at 724.2 and 710.6 eV were assigned to Fe 2p1/2 and Fe 2p3/2. The satellite characteristic emission at 717 eV further supports the oxidation state of Fe3+, and no Fe2+ and Fe signals were observed (see Supporting Information, Fig. S2(b)). The O1s peak with a binding energy of 529.4 eV can be classified as Bi/Fe-O (see Supporting Information, Fig. S2(c)). These results indicate that the BFO was successfully prepared.
Fig. 2(a) shows density–voltage (J–V) curves of the FTO/BFO and FTO/Au/BFO photoelectrodes. Both BFO and Au/BFO showed photocathode characteristics in accordance with the literature reports[23]. Compared with BFO, the photocurrent density of Au/BFO in the whole scanning voltage was significantly increased, which undoubtedly confirms the excellent characteristics of Au/BFO in photoelectric conversion. For convenience of comparison, density–time (J–t) curves of –0.4 V were tested under the same experimental conditions (Fig. 2(b)). The photocurrent intensity of Au/BFO was approximately 3 times that of the BFO. Similarly, the IPCE of Au/BFO was significantly improved to 16.4% compared to BFO (Fig. 2(c)). These results indicate that the introduction of the Au buffer layer significantly improves the performance of the photoelectrode in the PEC water splitting. In addition to increasing the photocurrent density, we also studied the stability curves of the photoelectrode under illumination for 10 000 s. As shown in Fig. S3(a), the photocurrent of BFO photoelectrode showed an obvious decreasing trend. However, the photocurrent density of Au/BFO remained above 80% after 10 000 s, and remained stable in general without significant decrease. In our previous study, the ferroelectric properties of BFO films were discussed in detail using piezoresponse force microscopy (PFM)[23]. Meanwhile, we also tested the polarization–electric field (P–E) hysteresis loops of BFO and Au/BFO, which further confirmed the ferroelectric properties of the two samples (see Supporting Information, Fig. S3(b)). Theoretically, the charge separation performance of ferroelectric materials could be further optimized by applying polarization voltage[24]. As shown in Fig. 2(d), when the positive polarization voltage of 3 V was applied to the Au/BFO photoelectrode, the PEC performance of Au/BFO was further improved. Unsurprisingly, the photocurrent density of Au/BFO decreased significantly when the photoelectrode was negatively polarized at –3 V. In other words, photocurrent switching of the Au/BFO photoelectrode can be achieved by applying different polarization voltages. These results indicate that the combination of Au thin film and BFO promotes the separation and transport of charge in Au/BFO photoelectrode, reduces the loss caused by charge recombination, and enables Au/BFO to achieve efficient photoresponse and more stable photocurrent generation. Similarly, these results also confirm the unique advantages of using ferroelectric materials in the field of photoelectric conversion.

class="figure_img" id="Figure2"/>
Download
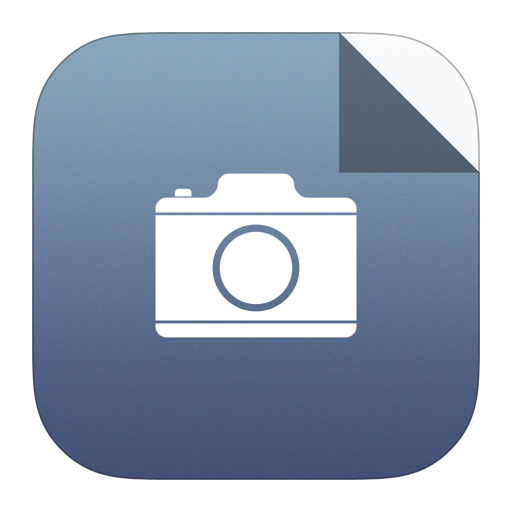
Larger image
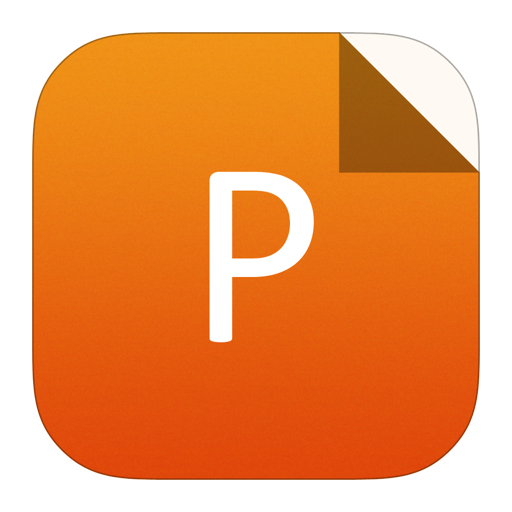
PowerPoint slide
Figure2.
(Color online) (a) J–V, (b) J–t and (c) IPCE curves of BFO and Au/BFO. (d) Photocurrent of the Au/BFO and positively or negatively poled Au/BFO under –0.4 V vs Ag/AgCl.
To explore the influence of the Au buffer layer on the light absorption performance of photoelectrode, we used an UV–vis spectrophotometer to study the light absorption of the two samples in the wavelength range of 300–800 nm. Compared with the BFO photoelectrode, the light absorption capacity of the whole region was slightly improved after the introduction of Au buffer layer (Fig. 3(a)). In addition, the effect of Au buffer layer on light absorption was further explained by calculating the light harvesting efficiency (ηLHE) of the photoelectrode. We calculate ηLHE with the absorbance data ηabs:

class="figure_img" id="Figure3"/>
Download
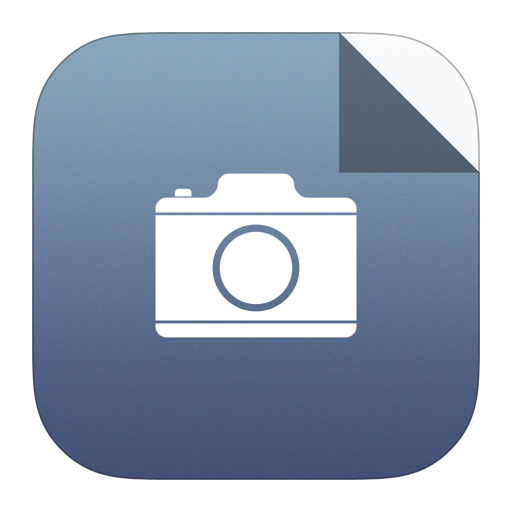
Larger image
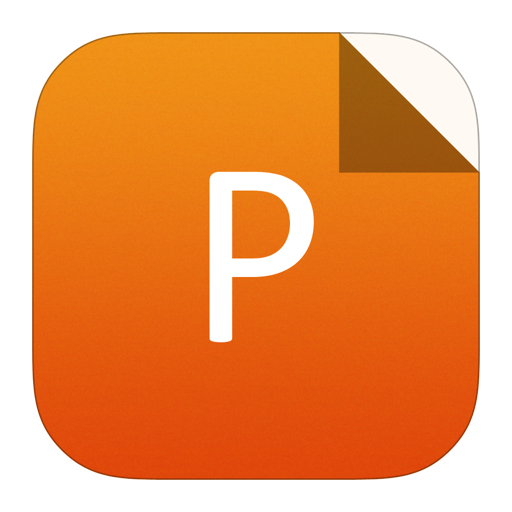
PowerPoint slide
Figure3.
(Color online) (a) Absorbance spectra, (b) light harvesting efficiencies (ηLHE), (c) electron flux and (d) charge separation efficiency (ηseparation) of BFO and Au/BFO.
$$ {eta }_{ m{LHE}}=left(1-{10}^{-{eta }_{ m{abs}}} ight)times 100{text{%}}text{,} $$ ![]() |
where ηabs = 100 – transmittance (T) – reflectance (R)[30]. In Fig. 3(b), although the ηLHE of Au/BFO was significantly enhanced within the wavelength range of 500–800 nm, the comparison with Fig. 3(a) shows that the light absorption capacity of BFO and Au/BFO was relatively weak within this range. Obviously, the significant improvement of PEC performance of Au/BFO photoelectrode was not mainly determined by light absorption.
Meanwhile, the electron flux on the photoelectrode in the wavelength range of 300–420 nm was integrated to calculate the photocurrent density (Jabs) at 100% absorption photon conversion efficiency, and the results are shown in Fig. 3(c). Jabs were estimated to be –1.917 and –1.918 mA/cm2 for BFO and Au/BFO, respectively, with only slight differences between them. This further indicates that in our experiment, the improvement of PEC performance of Au/BFO photoelectrode was not the main effect of light absorption. In general, the effective separation characteristics of photo-generated charge are conducive to improving the PEC performance of the photoelectrode. AgNO3 is usually added to the electrolyte as an electron scavenger for P-type semiconductors to investigate the charge separation efficiency in the PEC system[31]. The ηseparation of BFO and Au/BFO were calculated according to the equation: ηseparation ≈
m{AgNO}}_{3}} $

m{AgNO}}_{3}}$

Rapid separation of photogenerated carriers is crucial for enhanced PEC activity[32]. As shown in Fig. 4(a), PL spectra of BFO and Au/BFO was excited by the 405 nm laser. In general, lower recombination rate and higher separation efficiency of excited electron–hole pairs will lead to lower PL intensity[33]. The emission bands of BFO and Au/BFO were both concentrated around 430 nm, and the PL spectra of Au/BFO shows weaker emission intensity compared with the pristine BFO, which means that the Au buffer layer can effectively suppress the recombination between electrons and holes in BFO. We believe that the reduction of photogenerated carrier recombination rate is mainly attributed to the effective charge dissociation. In addition, we also tested the transient PL (TRPL) spectra of two samples. As shown in Fig. 4(b), a slower PL decaying in the Au/BFO samples can be observed, which means that Au/BFO has a longer fluorescence lifetime. Generally, a longer fluorescence lifetime represents a more efficient electron–hole pair separation[34]. In other words, the Au buffer layer is conducive to improving the ηseparation of the photoelectrode.

class="figure_img" id="Figure4"/>
Download
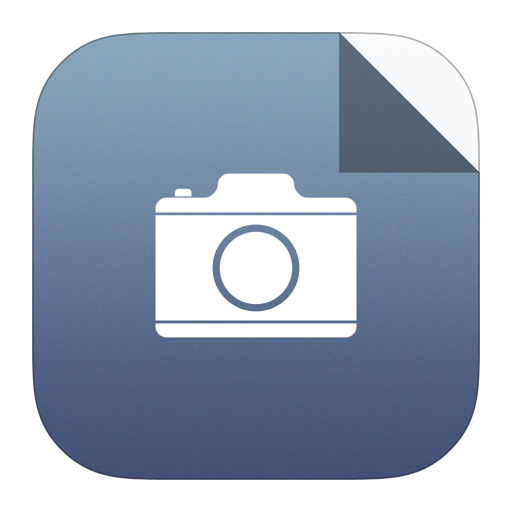
Larger image
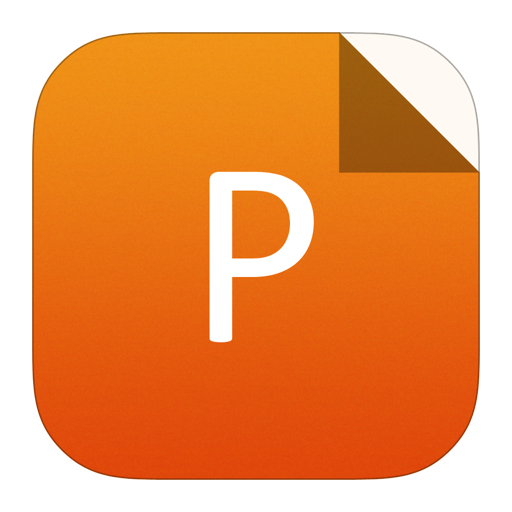
PowerPoint slide
Figure4.
(Color online) (a) The PL spectra and (b) transient PL decay spectra of BFO and Au/BFO.
Electrochemical impedance spectroscopy (EIS) was used to further analyze the reasons for the improved PEC performance of the photoelectrode[16, 35]. The Nyquist curves of the two photoelectrodes are shown in Fig. 5(a). In general, the arc radius of Nyquist curves was closely related to charge transfer resistance. A smaller arc radius means a smaller interface resistance; that is, a higher separation efficiency of photogenerated electron–hole pairs[36]. In contrast, the arc radius after the introduction of Au buffer layer becomes smaller, indicating that the existence of the Au buffer layer was conducive to faster charge migration and efficient separation of electrons and holes, which can improve the PEC performance of the photoelectrode. To understand the charge transfer kinetics, we tested the Mott-Schottky curves of the photoelectrode in the electrolyte. The negative Mott-Schottky curve slope indicates the P-type characteristics of the BFO and Au/BFO photoelectrodes[16]. The Mott-Schottky equation and the Mott-Schottky curve slope can be used to calculate the carrier density of semiconductors[37]:

class="figure_img" id="Figure5"/>
Download
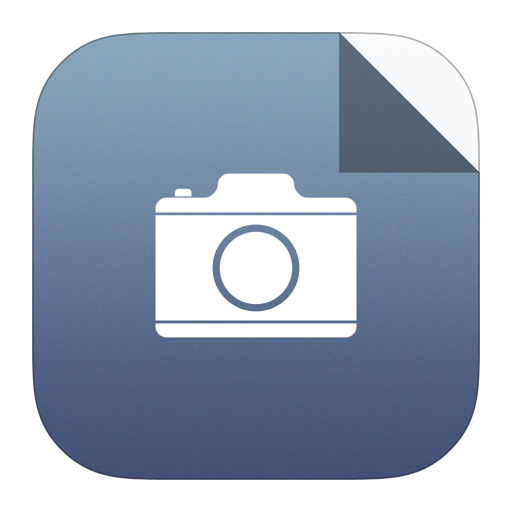
Larger image
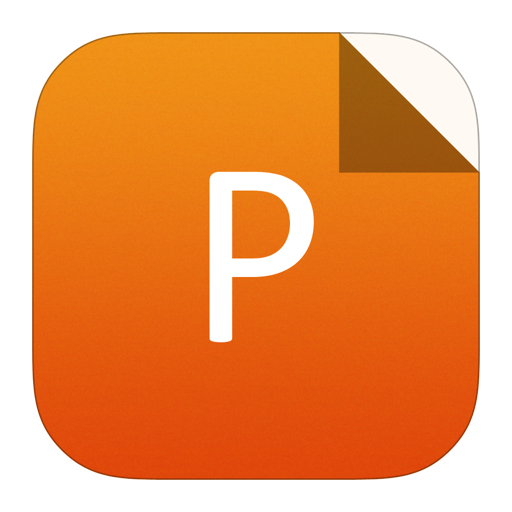
PowerPoint slide
Figure5.
(Color online) (a) Nyquist plots of electrochemical impedance spectra and (b) Mott-Schottky plots of BFO and Au/BFO.
$$ frac{1}{C}=frac{2}{e{varepsilon }_{0}varepsilon {N}_{mathrm{d}}}left[left(V-{V}_{mathrm{f}mathrm{b}} ight)-frac{kT}{e} ight]text{,} $$ ![]() |
$$ {N}_{mathrm{d}}=frac{2}{e{varepsilon }_{0}varepsilon }{left[frac{mathrm{d}left(dfrac{1}{{C}^{2}} ight)}{mathrm{d}V} ight]}^{-1}text{,} $$ ![]() |
where e, Nd, ε, ε0 and V correspond to electron charge, carrier density, dielectric constant, permittivity of vacuum and applied bias at the electrode, respectively. As depicted in Fig. 5(b), the fitting line of the Mott-Schottky curve slope shows that the Mott-Schottky curve slope of Au/BFO electrode was relatively low, signifying the high carrier density. It is well-known that the increase of carrier density can promote the separation and transfer of photoexcited electrons, thus reducing the occurrence of photogenerated electrons and hole recombination, which is of great significance to improve the PEC performance of photoelectrodes.
Based on these results, the charge transfer mechanism in Au/BFO photoelectrodes will be discussed. The work function of FTO, Au and BFO are 4.9, 5.1 and 4.8 eV, respectively[25, 38], as shown in Fig. 6(a). When BFO is in contact with FTO or Au respectively (Figs. 6(b) and 6(c)), the free electrons will flow from FTO or Au to BFO until FTO or Au is aligned with the Fermi energy level of BFO. At equilibrium, the surfaces of BFO and FTO or Au were positively and negatively charged respectively. Meanwhile, the energy band at the interface of FTO/BFO or Au/BFO bends upward under the effect of an electric field. Since the work function of Au was greater than that of FTO, the Au/BFO interface will have greater bending. This band bending promotes the photo-excited electron-hole pairs migration and reduces bulk recombination. In the absence of a polarization voltage, the electrons in BFO will slowly flow to the electrolyte and participate in H+ reduction to H2 (Fig. 6(d)). When Au/BFO was polarized by applying a positive voltage (Fig. 6(e)), Ep with a direction from the BFO/electrolyte interface to Au was generated on the BFO film[31]. The energy band at the BFO/electrolyte interface bends downward, which provides a driving force for charge separation and facilitates charge carrier transport. In contrast, a negative polarization voltage causes the energy band to bend upwards (Fig. 6(f)). It was difficult for electrons in Au/BFO to migrate to the electrolyte, which reduces the charge separation efficiency, increases the occurrence of electron–hole recombination, and thus decreases the current density.

class="figure_img" id="Figure6"/>
Download
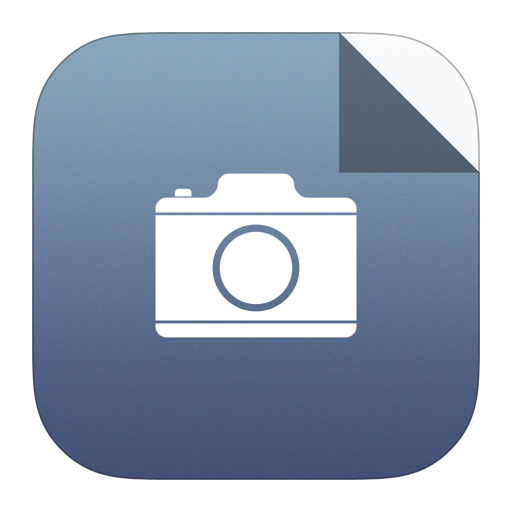
Larger image
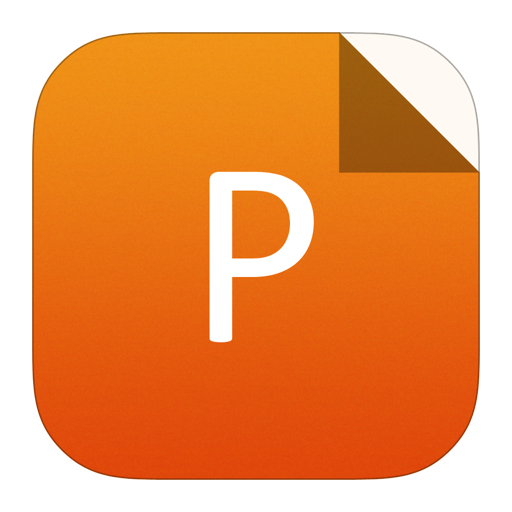
PowerPoint slide
Figure6.
(Color online) (a) Schematic of work function of FTO, Au and BFO. Schematic of the electron-transfer mechanism of (b) FTO/BFO and (c) FTO/Au/BFO. The photogenerated carrier transfer mechanism of Au/BFO photoelectrode under (d) no poling, (e) positive poling and (f) negative poling.
4.
Conclusion
In summary, an FTO/Au/BFO photoelectrode was prepared by introducing an Au buffer layer by magnetron sputtering. This can not only significantly improve the PEC performance of the photoelectrode but also improve its stability. The results demonstrate that the significant improvement of PEC performance of Au/BFO photoelectrode in this experiment was not mainly dependent on light absorption. The presence of the Au buffer layer can reduce the resistance of charge transfer, promote the transport of the charge carriers and improve the charge separation efficiency, which was an important reason to enhance the performance of PEC. In addition, ferroelectric polarization can further accelerate the separation and transport of photoelectric–hole pairs and improve the photoelectric conversion efficiency of PEC system. The introduction of the Au buffer layer enhances the charge separation of ferroelectric thin films, which provides an effective idea for the preparation of efficient photoelectrode.
Acknowledgements
This work was supported by National Natural Science Foundation of China (Grant No. 51702130), the Innovation/Entrepreneurship Program of Jiangsu Province and the project of Zhenjiang Key Laboratory of Advanced Sensing Materials and Devices (No. SS2018001). D. C. appreciates the support from Jiangsu Specially-Appointed Professors Program.
Appendix A. Supplementary materials
Supplementary materials to this article can be found online at