1.
Introduction
Photoeletronic detection, which can be implemented by either direct detection or coherence detection, is widely used as one of the most important information technologies. Compared with direction detection, coherence detection has many super advantages, such as multi-parameters detection[1, 2], higher detection sensitivity[3], lower detection power, and the higher signal–noise ratio[4, 5]. The performance of the coherence detection is inversely proportional to the linewidth of the light source, so the linewidth has a major impact on those super advantages. In recent years, the surge in data load has led to the expansion of coherent communication from local networks to data centers[6]. The rise of autonomous vehicles leads to the vehicle-mounted frequency-modulation continuous-wave Lidar into the civil market[7]. The internet of things[8] makes the distributed optical sensing widely used and connected. Besides, integrated microwave photonics[9], integrated optical beam steering[10], photonic analog-to-digital conversion[11], and the generation of low-noise and widely tunable microwave to terahertz signal[12] need low noise diode lasers as information carriers. All the above-mentioned applications require a light source not only narrow linewidth, but low cost and energy consumption.
Although fiber/gas/solid-state lasers with Hz-scaled linewidth can be implemented through different assembly techniques on lab platforms[13-15], these light sources are bulky in size, heavy in weight, and are also expensive and highly energy-consuming. In contrast to the above light sources, semiconductor lasers based on III–V direct band-gap materials are attractive because of their small footprint, high power efficiency (up to 85%), and low cost. However, the conventional DFB/DBR semiconductor lasers based on internal feedback is very hard to reduce the linewidth below MHz, because of the intrinsic large cavity loss and short cavity length[16, 17]. In order to overcome the limitation of these semiconductor lasers, high Q passive external cavity feedback has been introduced and proven to be an effective method to reduce the linewidth[18-21]. The passive external cavity, which is used in the external cavity optical feedback technology, can be divided into three types: discrete external cavity, assembled external cavity and waveguide external cavity. Among them, the discrete and assembled external cavities are the non-integrated external cavities, which include free-space bulk diffraction gratings[22], high Q whispering gallery mode resonators[23] and Bragg fibers[24], etc. Although extremely narrow linewidth lasers have been achieved utilizing these non-integrated cavities, they are still large-sized and highly sensitive to acoustic perturbation. Moreover, they lack long-term stability or have high diffraction loss in the coupling from free space to tightly guided waveguides. In addition, they are expensive because they cannot fabricate through batch processing. Compared with the above-mentioned non-integrated external cavities, WEC-NLSLs can greatly reduce the size, volume and weight while achieving narrow linewidth. Because the waveguide external cavity can be manufactured on a single substrate through semiconductor batch processing, which makes it possibly integrated with other components and improves the stability, the reliability and the cost. Therefore, such a WEC-NLSL is required for the above-mentioned coherent detection applications and becomes a focus of research in recent years.
In this paper, WEC-NLSLs are reviewed. In Section 2, we show an analysis model of a semiconductor laser with a waveguide external cavity and discuss the possible approaches to reduce linewidth. In Section 3, technologies that are related to improving the linewidth performance of WEC-NLSL are analyzed from dielectric waveguide materials to integration methods. In Section 4, we describe the latest technologies regarding the performance of these WEC-NLSLs, and the challenges they faced. Finally, the future research direction and applications have been prospected.
2.
Principle of WEC-NLSL
A WEC-NLSL, as is illustrated in Fig. 1(a), includes a semiconductor active section and a passive external cavity. The active section, which typically contains a III–V semiconductor quantum wells structure, is used to provide the optical gain for the whole cavity and thereby determines the lasing wavelength range. The passive external cavity is used to select the lasing wavelength and in the meantime to reduce the linewidth.

class="figure_img" id="Figure1"/>
Download
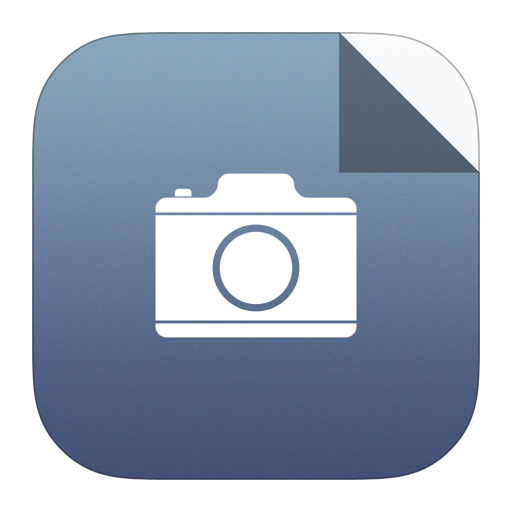
Larger image
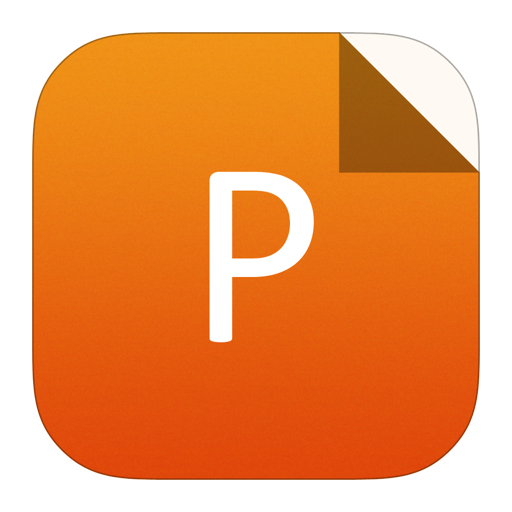
PowerPoint slide
Figure1.
(Color online) External cavity feedback semiconductor laser. (a) Block diagram. (b) Equivalent model.
The linewidth, which is defined as the spectral width of a laser’s lasing mode, is affected by the noise due to the phase fluctuation in the laser output power. At the lower frequency range, the noise spectrum density, which is closely related with the measured frequency, is dominated by various 1/f noises and other types of “technical noises” that originate from external sources. At higher frequencies where 1/f noise and other technical noises become vanished, white noise caused by the random process of spontaneous emission and carrier fluctuations becomes dominant. This is irrelevant to the measured frequency and completely determined by the laser structure, which makes the main contribution to the minimum achievable linewidth of a laser. In this paper, we limit our study to the linewidth under higher frequency noise, which is also referred to as the Lorentz linewidth[25].
To obtain the steady-state analysis and qualitatively evaluate the linewidth of a WEC-NLSL, the entire waveguide external cavity is treated as a resonant mirror, so the equivalent cavity model of the WEC-NLSL is shown in Fig. 1(b).
According to the above equivalent model and the adiabatic chirp theory, the linewidth of a WEC-NLSL can be expressed as follows[26]:
$$Delta nu = frac{{Delta {nu _0}}}{{{F^2}}} = frac{{Delta {nu _0}}}{{{{left( {1 + A + B} ight)}^2}}},$$ ![]() | (1) |
$$A = frac{1}{{{tau _0}}}{ m{Re}}left{ {jfrac{{ m{d} { m{ln}} {R_{ m{eff}}}(omega )}}{{ m{d}omega }}} ight} = -frac{1}{{{tau _0}}}frac{{ m{d}{varphi _{ m{eff}}}}}{{ m{d}omega }},$$ ![]() | (2) |
$$B = frac{{{alpha _{ m{H}}}}}{{{tau _0}}}{ m{Im}}left{ {jfrac{{ m{d}{ m{ln}} {R_{ m{eff}}}(omega )}}{{ m{d}omega }}} ight} = frac{{alpha _{ m{H}}}}{{{tau _0}}}frac{{ m{d}{ m{ln}} left| {{R_{ m{eff}}}left( omega ight)} ight|}}{{ m{d}omega }},$$ ![]() | (3) |
$$Delta {nu _0} = frac{1}{{4pi }}frac{{upsilon _{ m{g}}^2hupsilon {n_{ m{sp}}}{alpha _{ m{tot}}}{alpha _{ m{m}}}}}{{{P_0}left[ {1 + dfrac{{{R_1}}}{{left| {{R_{ m{eff}}}left( omega ight)} ight|}}dfrac{{1 - {{left| {{R_{ m{eff}}}left( omega ight)} ight|}^2}}}{{1 - R_1^2}}} ight]}}left( {1 + alpha _H^2} ight),$$ ![]() | (4) |
where Δν0 represents the Schawlow–Townes linewidth of the solitary Fabry–Pérot laser with mirror reflectivity R1 and
m{eff}}left(omega
ight)
ight| $

m g}{L_{
m a}}}}{c}$


m{m}}} = - dfrac{1}{{{L_{
m{a}}}}}{
m{ln}} left( {{R_1}left| {{R_{
m{eff}}}left( omega
ight)}
ight|}
ight)$

In Eqs. (1)–(4), factor A reflects the increase in a roundtrip accumulated phase, which is equivalent to the effective cavity length enhancement mainly provided by the external cavity. Factor B represents the magnitude of the optical negative feedback effect, which is proportional to the magnitude of αH. From the equations above, one can see that both factors A and B have a direct impact on the laser’s spectrum linewidth. An increase of the factor A means that the passive section length of the laser cavity becomes longer, leading to the increased photon lifetime. Fig. 2(a) shows the effective cavity length as a function of the optical frequency in the external cavity configuration, and one can see that only when the lasing frequency is aligned with the resonance peak frequency, both the effective cavity length and factor A is maximized. An external cavity also induces an optical negative feedback effect, which is mainly described by factor B. The optical negative feedback effect can stabilize the lasing frequency, thereby effectively reducing the linewidth, as shown in Fig. 2(b). Since factor A is maximized at the resonance frequency of the external cavity, and factor B peaks at the frequency that is slightly detuning from the external cavity resonance, the optimal point must be carefully selected to obtain the narrowest laser linewidth. Also, as the linewidth enhancement factor αH has a positive effect in factor B but a negative effect in the Schawlow–Towns linewidth, the quantum well structure should be carefully optimized as well. Furthermore, an increase in the output power P0 leads to a reduction in the linewidth, as the linewidth is inversely proportional to the laser power as shown in Eq. (4). However, the higher laser power will result in high amplified spontaneous emission noise, which further broadens the linewidth.

class="figure_img" id="Figure2"/>
Download
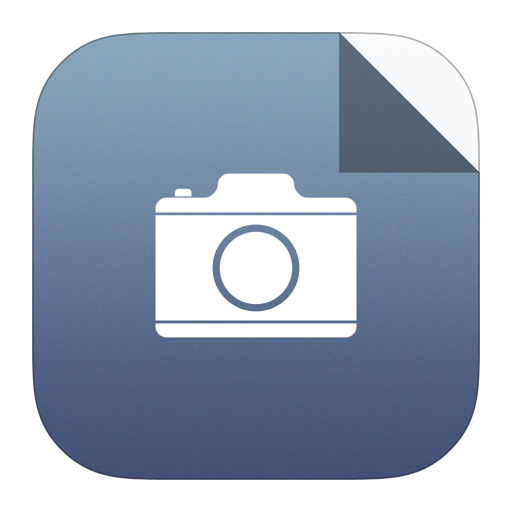
Larger image
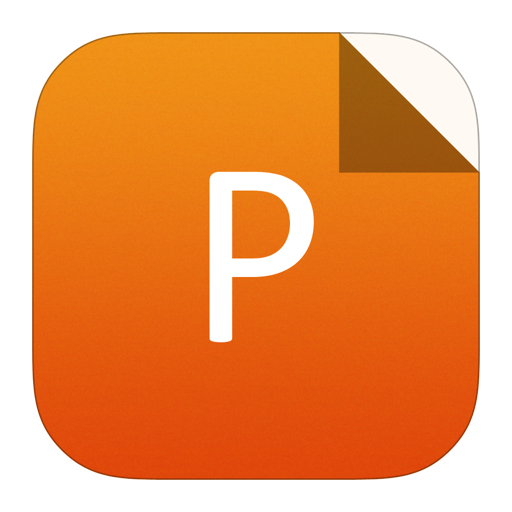
PowerPoint slide
Figure2.
(Color online) (a) Illustration of the role of factor A. (b) Illustration of factor B (optical negative feedback).
As discussed above, only the loss-reduction behavior can monotonously improve the linewidth performance of the semiconductor lasers. However, the internal loss αi is difficult to reduce as the active material and structure are basically fixed. Therefore, the loss-reduction in the external cavity becomes the most effective way to achieve narrow linewidth through the increase of factor A and B, which is the reason why narrow-linewidth semiconductor lasers are usually realized by the external cavity.
3.
Technologies of WEC-NLSL
3.1
Low-loss waveguide
As is discussed above, linewidth improvement can be made monotonously by employing a low-loss/long passive section external waveguide. Hence, our discussion only focuses on the external passive waveguide, from the point of both material choice and waveguide configurations.
In order for the passive waveguide to work more effectively in terms of linewidth improvement, a key requirement is that the waveguide has low loss. Thus far, silicon oxynitride (SiON), silica (SiO2), Si-wire, silicon nitride (Si3N4), and silicon-on-insulator (SOI) have been selected as waveguide external cavity platforms and kHz-scaled linewidth has been achieved. Their basic optical properties are listed in Table 1.
Platform | Propagation loss (dB/cm) | Group index | Refractive index contrast |
SiON/SiO2[27] | 0.05 | 1.4816 | 0.025 |
SiO2/Si[28] | 0.023 | 1.465 | 0.02 |
Si-wire/SiO2 [29] | 2.4 | 3.47 | – |
Si3N4/SiO2[30] | 0.013 | 1.996 | 0.5 |
Ultralow-loss SOI[31] | 0.16 | 3.61 | 2.145 |
Table1.
Optical properties of the waveguide external cavity platforms.
Table options
-->
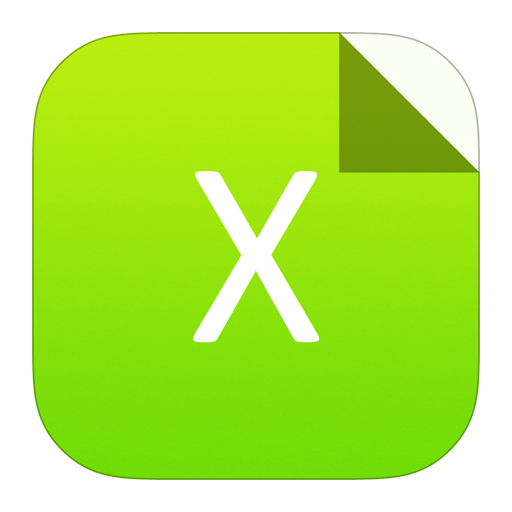
Download as CSV
Platform | Propagation loss (dB/cm) | Group index | Refractive index contrast |
SiON/SiO2[27] | 0.05 | 1.4816 | 0.025 |
SiO2/Si[28] | 0.023 | 1.465 | 0.02 |
Si-wire/SiO2 [29] | 2.4 | 3.47 | – |
Si3N4/SiO2[30] | 0.013 | 1.996 | 0.5 |
Ultralow-loss SOI[31] | 0.16 | 3.61 | 2.145 |
As shown in Table 1, the SiON and silica waveguide external cavity have low propagation loss, the WEC-NLSL with a linewidth of tens of kHz was firstly realized[32]. However, the refractive index contrast of SiON/SiO2 is small. In order to realize the miniaturization of WEC-NLSL, a trade-off has to be made between the linewidth performance and the device footprint, so the linewidth cannot be further reduced based on this platform. Moreover, high tuning power consumption due to their weak thermos-optical (TO) effects is another limiting factor[33]. The external waveguides based on the Si-wire, which exhibits large group index and refractive index contrast, are more compact and also have low power consumption, but the linewidth is still tens of kHz. This is attributed to the nonlinear effect in Si-wire dielectric waveguides (such as two-photon absorption). Compared with the above materials, Si3N4 exhibits well-known advantages, such as large refractive index difference, large transparency range (400–2400 nm), low linear propagation loss (~0.1 dB/cm), low nonlinear effects. The linewidth of WEC-NLSLs based on this platform is substantially improved, which is mostly on the order of kHz or even the sub-kHz level. Besides, the ultralow-loss SOI waveguide with extremely large refractive index contrast, which makes it possible to fabricate very compact and high-density components, becomes another competitive material platform for the external cavity. So far, the linewidth of sub-kHz has been achieved based on this platform. Moreover, other high contrast and low-loss dielectrics materials were being investigated[34, 35].
3.2
Long optical cavity length
In order to appreciate the influence of the optical length and the loss in the external cavity waveguide on the Lorentz linewidth of the lasers more clearly, Boller’s team[36] made a quantitative estimate and the results are shown in Fig. 3. In order to avoid discussing the specially designed Vernier transmission spectrum for the feedback length, the calculation was performed by setting the parameter B to be zero.

class="figure_img" id="Figure3"/>
Download
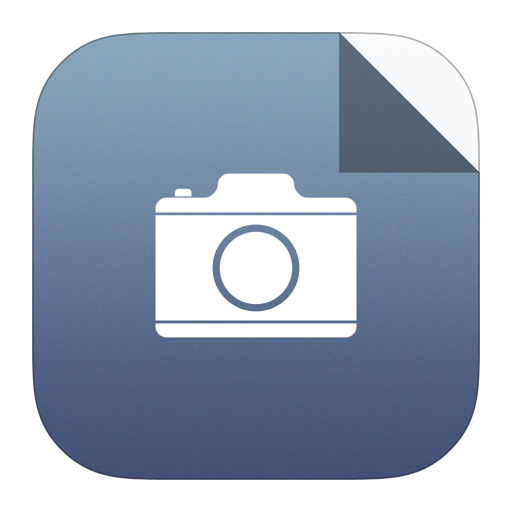
Larger image
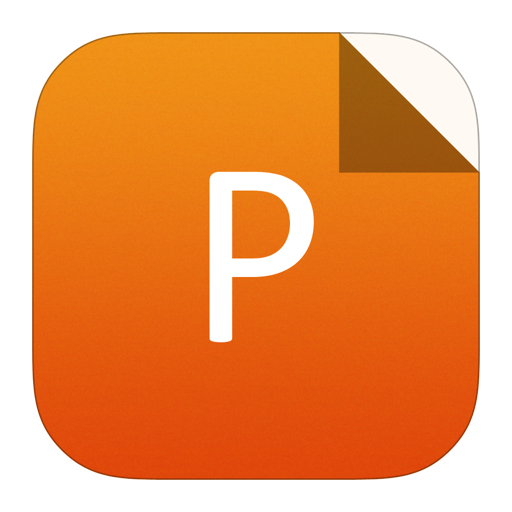
PowerPoint slide
Figure3.
(Color online) The relationship between the external cavity length, the waveguide loss and the intrinsic linewidth of the laser [36].
As shown in Fig. 3, no matter what external cavity material is selected, when the optical length is less than 1 mm, the linewidth is limited to 100 kHz. The longer the optical path of the external cavity, the more significant the impact of the waveguide loss on the linewidth. Therefore, one has to consider the feasible approach to extend the external cavity length within a small single chip. Currently, two approaches have been developed, namely micro-ring resonators (MRR) and spiral waveguides. MRR is a traveling-wave resonator, its effective optical path Leff can be expressed as the following[37]:
$${L}_{ m{eff}}=frac{lambda }{beta }left|frac{{ m{d}}varphi }{{ m{d}}lambda } ight|,$$ ![]() | (5) |
where β = 2πneff/λ is the propagation constant (complex) and φ = βL is the phase change of light. L is the circumference. At the critical coupling condition, Eq. (5) becomes
$${L}_{ m{eff}}=frac{1-kappa }{kappa }L,$$ ![]() | (6) |
where κ is the power coupling coefficient. From Eqs. (5) and (6), one can see that the effective cavity length Leff is not only determined by the physics length, but also strongly dependent on κ and propagation loss α. Fig. 4 shows the relationship between the enhancement factor (Leff/L) of the optical path and κ under different waveguide losses.

class="figure_img" id="Figure4"/>
Download
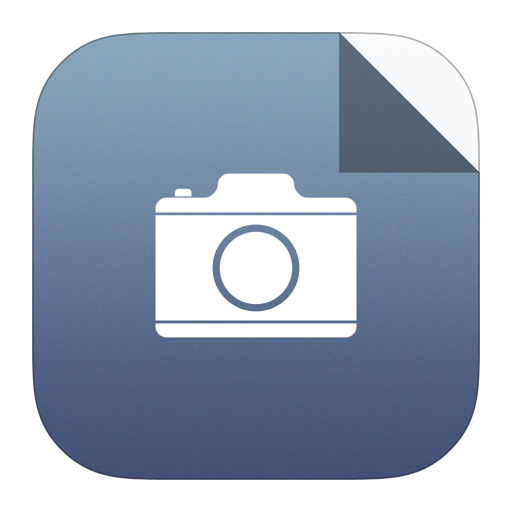
Larger image
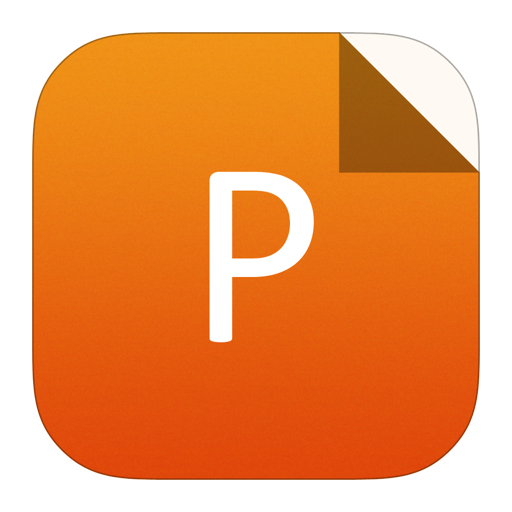
PowerPoint slide
Figure4.
(Color online) Optical path extension under different coupling coefficients and different losses.
As shown in Fig. 4, in the weakly coupled low-loss MRR, the effective optical path can be significantly increased. Thus, in order to achieve a long effective optical length, the MRR circumference and κ must be appropriately designed for waveguides with different losses. Fig. 4 shows that the Leff/L of about 400 when κ of a lossless MRR is about 10% (black line). This means that an MRR with a circumference of 2.5 mm can actually be equivalent to a 1-m-long optical length in terms of the linewidth performance. At such conditions, the Lorentz linewidth of Hz level is expected, as shown in Fig. 3. Based on this simple analysis, one can see that it is possible to achieve a very narrow-linewidth performance by using a well-designed WEC-NLSL. Besides, multiple MRRs can also provide wide spectrum tuning because of the Vernier effect.
3.3
Integration technology
Although the external cavity based on the MRR configuration described above is the most effective way for reducing the linewidth, the external cavity has to be integrated with an active gain section. And this would rule out the consideration of using pure Si-based materials as they are unable to produce light efficiently. Whilst germanium lasers on silicon have been demonstrated, unfortunately, their efficiency is inadequate for most practical applications[38]. Thus, III–V materials (InP, GaAs, etc) with a direct bandgap provide the only feasible solution for the active gain section, but their integration between the III–V active gain section and dielectric waveguide external cavity is proven to be full of challenges. Tremendous progress has been made in III–V quantum dot laser on Si[39], yet it is difficult to couple the light from the quantum dot layer into the silicon waveguide layer.
To date, the more commonly used integration methods are hybrid integration and heterogeneous integration. Hybrid integration refers to assembling the III–V active gain section and the external silicon photonic waveguide together by the butt-coupling technique. Although the waveguide external cavity and gain chip, which can be optimized independently, are relatively simple to fabricate in this hybrid integration, the challenge lies in the butt-coupling between the two chips. Firstly, the large coupling loss induced by the mode mismatch must be reduced as far as possible. The spot size converter (SSC), which is typically shown in Fig. 5(a), is widely employed to reduce their optical mode coupling loss. However, millimeter-level long converters are usually required for achieving small coupling loss, which is not favorable for compact narrow linewidth lasers. Secondly, the air gap between the two chips is unavoidable, thus the facet reflection is another problem, and polarization loss are also introduced, which degrade the laser linewidth. Moreover, the facets with extremely low reflectivity are difficult to implement. Both issues above will impact the laser linewidth performance.

class="figure_img" id="Figure5"/>
Download
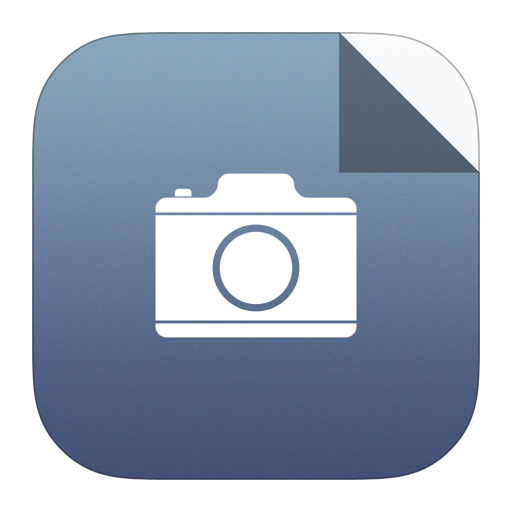
Larger image
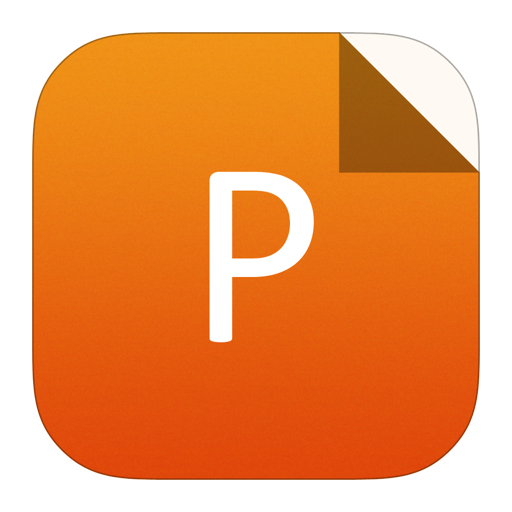
PowerPoint slide
Figure5.
(Color online) (a) SSC structure diagram[42]. (b) Heterogeneous integration[41].
Heterogeneous integration is a technique that directly bonds unprocessed III–V material on the top of silicon waveguides. Therefore, it is compatible with the Si CMOS process, which can effectively reduce the manufacturing cost. Compared with the hybrid integration, devices with heterogeneous integration have improved the mechanical performance, provided the bonding quality is adequate. However, the quality is actually affected by a number of factors such as bonding process, lattice mismatch, thermal expansion coefficients of the materials, and is proven to be a challenge. Currently, the main bonding methods are molecular bonding based on van der Waals force[40] and DVS-BCB adhesive bonding[41]. Molecular bonding [see Fig. 5(b)] is excellent, but high-quality molecular bonding with large area is a challenge. Compared with molecular bonding, adhesive bonding is easier to realize. However, BCB material between Si and III–V gain material becomes a limitation to the high performance of WEC-NLSL because of its poor thermal conductivity.
4.
The state of art of WEC-NLSL
4.1
Butt coupling technology-based hybrid lasers
The butt coupling technology, which is relatively mature and well-developed, is very attractive because it offers one the opportunity to optimize the gain chip and external cavity separately. Several dielectric waveguide platforms, such as SiON, SiO2, Si-wire, and Si3N4, have been developed for manufacturing external cavities to form so-called hybrid integrated lasers. The main representative research institutions are the NEC Corporation of Japan, Tohoku University, Tsinghua University, the University of Twente, Clemson University, Cornell University, and the University of California, Santa Barbara (UCSB).
Fig. 6 shows the progress made in reducing the laser spectral linewidth based on the butt coupling technology under different material platforms.

class="figure_img" id="Figure6"/>
Download
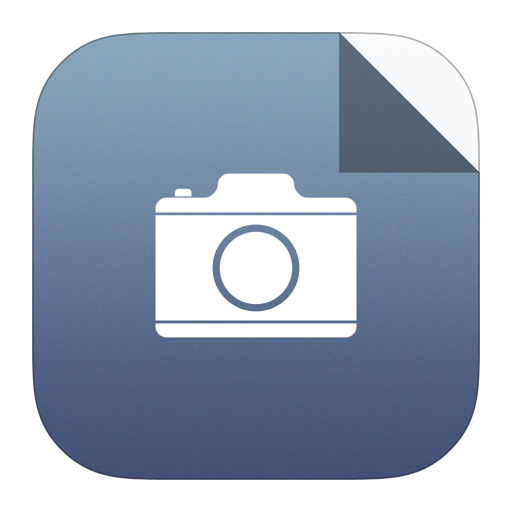
Larger image
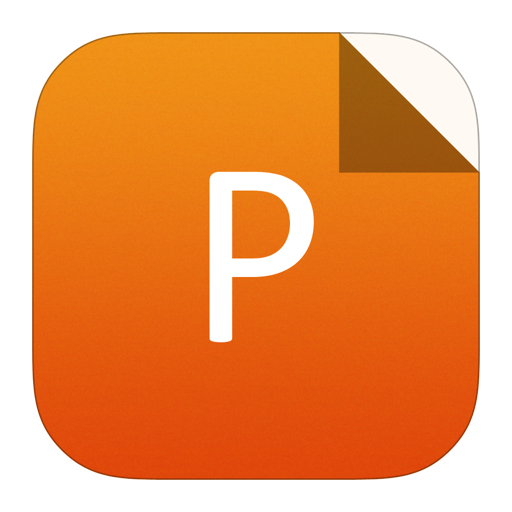
PowerPoint slide
Figure6.
(Color online) The intrinsic linewidth of hybrid integrated laser based on butt coupling technology: a-[43], b-[44], c-[45], d-[46], e-[47], f-[48], g-[49], h-[50], i-[51], j-[52], k-[53], l-[54], m-[55], n-[56], o-[57], p-[58], q-[59], r-[60], s-[61], t-[62], u-[63], v-[64].
As shown in Fig. 6, early researchs about WEC-NLSLs based on SiON materials were mainly conducted by NEC, with a footprint of about 6.5 × 4.5 mm2, and a high wavelength tuning power consumption. Its linewidth is around 100 kHz[32, 43]. At the same time, Nanyang Technology University and Bell Labs reported external cavity lasers based on the SiO2 dielectric waveguide platform, and a linewidth of hundreds of kHz has been obtained[47, 51]. Subsequently, Tohoku University has carried out a lot of researches on the Si-wire waveguide. By improving the frequency-selective structure of the waveguide external cavity, tens of kHz linewidths have been achieved[44, 45, 48, 49, 53, 56]. Compared with the SiON material external cavity lasers, the laser’s footprint has been reduced several times. In addition, NEC corporation reported a WEC-NLSL based on Si-wire waveguides for practical use in commercialized systems[52]. The excellent properties of Si3N4 material as an external cavity dielectric platform makes the WEC-NLSL based on this platform a major research object in recent years. The laser linewidth is mostly on the order of kHz[46, 50, 54, 55, 57-61, 63]. Optimizing the external cavity structure, such as using an ultralow κ grating or combining spiral waveguides, led to achieving sub-kHz linewidth[62, 64]. Among them, the 40 Hz linewidth[64], which is the narrowest laser linewidth reported so far, realized by the University of Twente using the MRR and spiral waveguide (Fig. 7).

class="figure_img" id="Figure7"/>
Download
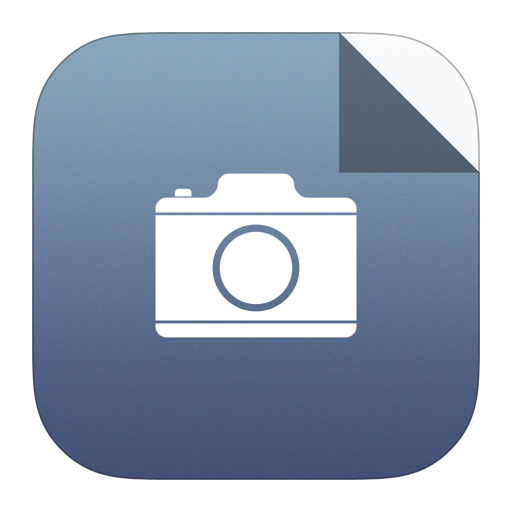
Larger image
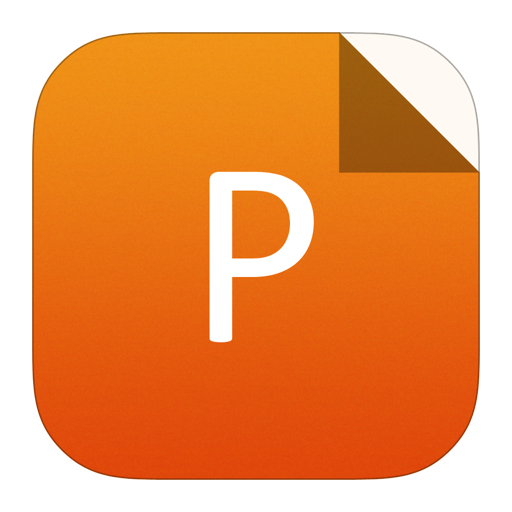
PowerPoint slide
Figure7.
(Color online) Schematic view of the hybrid laser based on a Si3N4 feedback circuit comprising a spiral and three MRRs[64].
Domestic researches on WEC-NLSLs started late, and only Huawei and Tsinghua University have made relevant reports[55, 60]. Although Huazhong University of Science and Technology has also fabricated similar waveguide external cavity lasers. Unlike the above two technologies, its SOA and Si waveguide external cavity were coupled through lens to get a linewidth of 130 kHz[65].
Due to the mature and well-developed butt coupling technology, Redfern Integrated Optics (RIO)[66] has already realized mass volume production of WEC-NLSL and can meet the demand of all kinds of sensing applications.
4.2
Heterogeneous integrated semiconductor lasers
Using heterogeneous integration technology for hybrid integration, the external cavity is manufactured only on the SOI platform. The main players include the III–V laboratory in France, the France CEA LETI, the University of Ghent in Belgium, and the UCSB in the United States. Among them, UCSB has done a lot of research and is in an international leading position.
UCSB first successfully bonded the III–V gain materials on a silicon waveguide in 2005[67], and then the University of Ghent in Belgium, in cooperation with the France III–V laboratory and CEA-LEti, manufactured the first WEC-NLSL with a linewidth of 1.7 MHz based on heterogeneous integration technology[68, 69]. Subsequently, UCSB successively reported several heterogeneous integrated lasers with different external cavity structures, but their linewidths were mostly around 100 kHz[70-74]. To further narrow the linewidth, they developed an ultralow loss SOI waveguide platform in 2018 and produced some high-performance external cavity frequency selection units, such as MRR and grating[31]. Based on this ultralow loss waveguide platform, they successfully reduced the linewidth down to sub-kHz[75, 76]. Among them, the laser using triple-MRRs obtained a linewidth less than 220 Hz[76], which is the narrowest linewidth of WEC-NLSLs based on the heterogeneous integration technology. The laser structure and frequency noise spectrum are shown in Fig. 8.

class="figure_img" id="Figure8"/>
Download
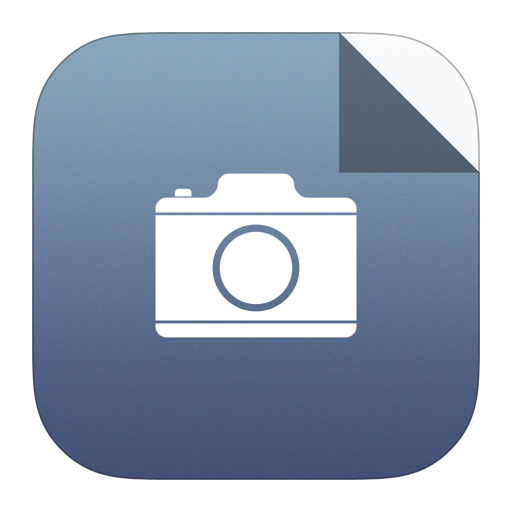
Larger image
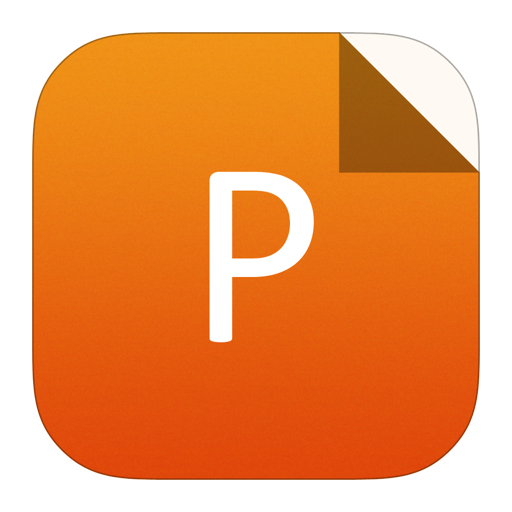
PowerPoint slide
Figure8.
(a) Laser structure diagram based on triple MRR. (b) Frequency noise spectrum[76].
The performance of heterogeneously integrated lasers produced by the Bowers’ team in recent years is shown in Table 2.
First author | Structure | SMSR (dB) | Tuning range (nm) | Min. linewidth (kHz) |
Hulme[70] | MRR | 35 | 40 | 338 |
Komljenovic[71] | MRR + LR | 45 | 54 | 50 |
Komljenovic[72] | MRR | >40 | 29 | 260 |
Liang[73] | MRR + LR | >40 | 40 | 150 |
Tran[74] | MRR + LR + MZI | >50 | 55 | 50 |
Huang[75] | Grating | >55 | – | 1 |
MRR + Grating | – | 0.5 | ||
Tran[76] | Dual MRR + LR | >45 | 40 | 2 |
Triple MRR + LR | >40 | 110 | <0.22 |
Table2.
The performances of heterogeneous integrated lasers.
Table options
-->
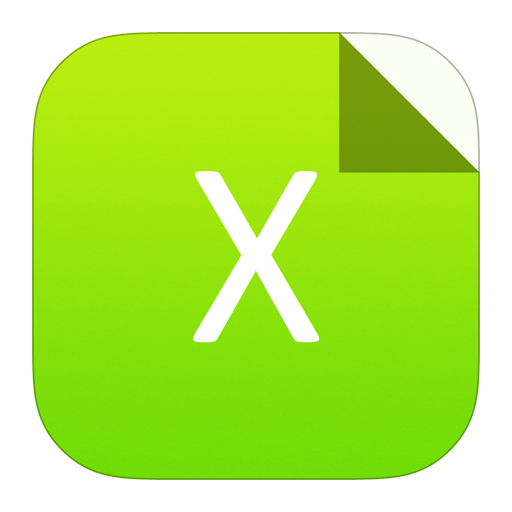
Download as CSV
First author | Structure | SMSR (dB) | Tuning range (nm) | Min. linewidth (kHz) |
Hulme[70] | MRR | 35 | 40 | 338 |
Komljenovic[71] | MRR + LR | 45 | 54 | 50 |
Komljenovic[72] | MRR | >40 | 29 | 260 |
Liang[73] | MRR + LR | >40 | 40 | 150 |
Tran[74] | MRR + LR + MZI | >50 | 55 | 50 |
Huang[75] | Grating | >55 | – | 1 |
MRR + Grating | – | 0.5 | ||
Tran[76] | Dual MRR + LR | >45 | 40 | 2 |
Triple MRR + LR | >40 | 110 | <0.22 |
Compared with butt coupling technology, there are no commercial lasers based on heterogeneous integration technology. However, this technology is considered to be one of the most promising technologies for realizing an efficient narrow linewidth light source.
5.
Summary and prospect
In summary, with the development of low-loss materials, hybrid integration and delicate designs based on the MRR unit, the intrinsic linewidth of the WEC-NLSL is gradually reduced down to the limitation. Moreover, other performances of these lasers are also enhanced rapidly. For example, by hybrid integrating a booster semiconductor optical amplifier, the laser output power reached up to 100 mW[52]. A wavelength range of more than 110 nm was obtained using three MRRs configurations[76]. With different gain chips, Cornell University has realized a triple-band hybrid laser system for optical beam steering in autonomous driving[61, 63].
WEC-NLSLs have already achieved a linewidth of sub-kHz, which meets the linewidth requirements of the most coherent detection applications. However, frequency stability and mode hopping become a common problem for this kind of narrow-linewidth laser. The long external cavity length will undoubtedly reduce the mode spacing, which will result in a serious frequency noise problem and degrades the whole performance of the lasers. Thus, how to obtain a stable frequency and narrow linewidth output is one of the key issues that must be solved in the practical applications of WEC-NLSLs. The optical negative feedback method proposed by Tohoku University seems to be a way forward to realize frequency stabilization and narrow linewidth simultaneously[77]. Besides, even though narrow linewidth has been achieved in such WEC-NLSLs, phase matching of the whole laser cavity must be satisfied for such lasers to realize stable operation, which is usually achieved by adjusting the refractive index of each resonance unit through thermal-optical effect, as all the external cavities are dielectric materials. This will result in high energy consumption and low tuning speed.
Compared with the narrow linewidth semiconductor lasers with dielectric waveguide external cavity based on hybrid and heterogeneous integration technology, monolithic integration completely based on the III–V semiconductor material system is a viable approach to achieve the required spectral linewidth as well as the cost and energy consumption, as it eliminates the need for the complex hybrid integration process, the whole chip can be fabricated through the conventional III–V semiconductor batch processing, and the coupling loss between the gain section and the waveguide external cavity can be negligible. However, the intrinsic loss of III–V materials is high, which prevent them from realizing a narrow linewidth laser. Researchers are working on the optimal fabrication process and laser structure. The Eindhoven University of Technology (TU/e) in the Netherlands reduces the InP waveguide loss from 2 dB/cm to below 0.4 dB/cm via local diffusion of zinc in 2016[78]. In 2019, Stefanos et al. in the TU/e[79] used MRR as an internal cavity to improve the wavelength selectivity and achieved a InP-based monolithic integration laser with a linewidth of 63 kHz. Our team have adopted a dual-core vertical coupling structure to compensate for the loss in the passive waveguide[80], meanwhile, amplified spontaneous emission noise can be filtered due to their gain narrowing phenomenon[81]. Based on this and our proposed structure, kHz-level monolithic integrated narrow linewidth semiconductor lasers are expected[82, 83]. We believe that III-V-based monolithic integrated WEC-NLSLs should be the best solution for all the above-mentioned problems and it is developing rapidly.
Acknowledgements
This work was supported by Jiangsu Province Key R&D Program (Industry Prospect and Common Key Technologies) (No. BE2014083); Jiangxi Natural Science Foundation Project (No. 2019ACBL20054)