1.
Introduction
With the development of quantum computing[1, 2], current algorithms for ensuring secrecy in data communication would pose a high security risk. The most promising approach to truly secure communication is quantum communication, offering secrecy based on physical laws. Currently developed quantum cryptography systems such as quantum key distribution (QKD)[3] rely on the no-cloning theorem of quantum bits (qubit). A very famous proposal of QKD was proposed by Bennett and Brassard in 1984 (BB84)[4], using the polarization states of single photons to distribute a secret key. The presence of an eavesdropper can be easily detected due to increased error rates in the key distribution. However, there are many challenges to realize such protocols, e.g. the development of perfect single-photon sources. These are not only critical for quantum cryptography, but also for quantum computing[5], quantum metrology[6] and quantum networks[7].
The first single-photon source was created by using an emission cascade in mercury atoms[8]. Today, after more than four decades’ development, there are many different types of single-photon sources, such as atomic ensembles[9], ion traps[10], single molecules[11, 12], semiconductor quantum dots (QDs)[13], defects in diamonds[14, 15], non-linear crystals[16], and many more[17]. With these sources, several QKD experiments have been realized in free-space, from laboratory scale of tens of centimetres to over 10 km[18–20]. The wavelength used in these experiments ranged from 550 to 780 nm. However, increasing the QKD distance using that wavelength range is very challenging due to low signal-to-noise ratios: On one hand, solar radiation strongly contributes to the background, since it is relatively high at that wavelength range (Fig. 1(a)). On the other hand, Rayleigh scattering in the atmosphere is proportional to 1/λ4 and therefore adds to the noise for short wavelength. In addition, practical applications vastly require data transmission over optical fibers. Due to the signal attenuation in the fiber, the loss of photons within visible wavelength range is more than 3 dB/km (see Fig. 1(b)), which is unsuitable for long-distance transmission. The two telecommunication windows with minimized attenuation at 1310 and 1550 nm, which are known as the O-band (0.3 dB/km) and C-band (0.15 dB/km), are more applicable and are widely used in the fiber-based long-distance telecommunications. Because of the relatively low solar radiation and Rayleigh scattering at these two windows, they are also applicable to free-space telecommunication. Therefore, many efforts are now spent on producing single-photon source at telecom wavelength.

class="figure_img" id="Figure1"/>
Download
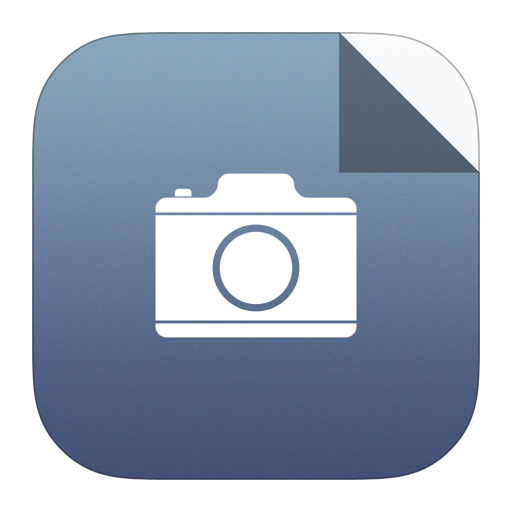
Larger image
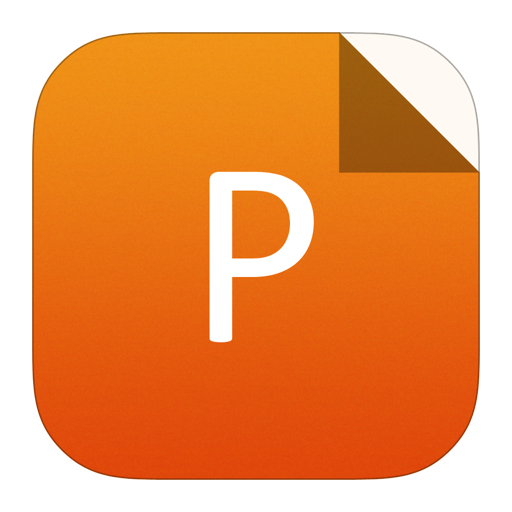
PowerPoint slide
Figure1.
(Color online) (a) Solar radiation spectrum from UV to near-infrared light. (b) Photon attenuation in optical fiber as a function of wavelength. Wavelengths at 1300 and 1550 nm are called telecom O-band and C-band, respectively, which are commonly used in fiber-based applications. (a) Adapted with permission from Ref. [21]. (Springer Nature) (b) Adapted from Ref. [22].
2.
Single photon emission
2.1
Concept
An ideal single-photon emission consists of exactly one photon at a time, which can be triggered on-demand, meaning that the user can emit a single photon at will. Furthermore, the emitted photons should be indistinguishable[23]. A simple two-level scheme of single-photon emission is shown in Fig. 2. One electron is excited to an excited state (high level) by an external pulse, then within a certain time it falls back to the ground state (low level) emitting a single photon.

class="figure_img" id="Figure2"/>
Download
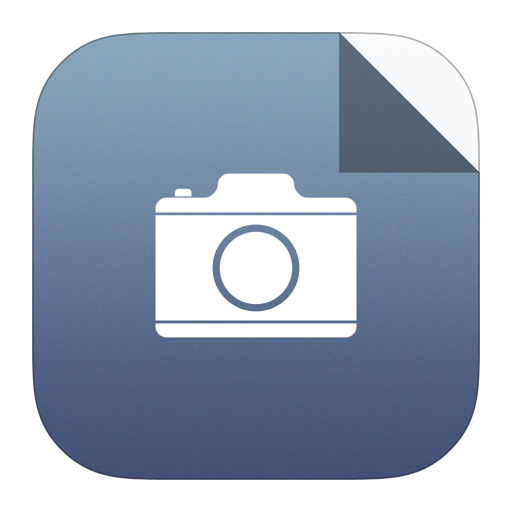
Larger image
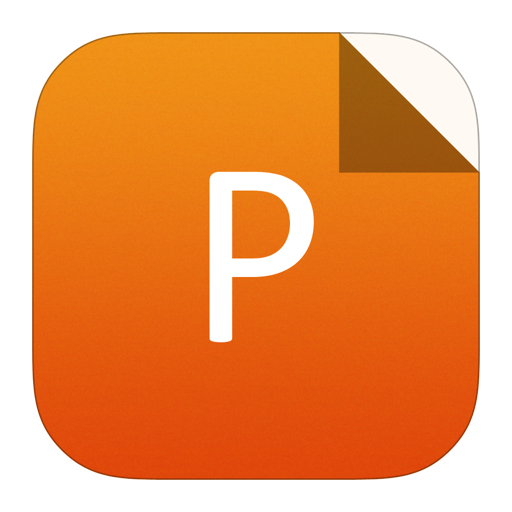
PowerPoint slide
Figure2.
(Color online) Scheme of single photon excitation and emission in a two-level system.
The single photon emission should obey sub-Poissonian statistics:
$Delta n < sqrt {bar n} ,$ ![]() |
where ?n is the standard deviation of the photon numbers in a certain time interval, and

However, it is experimentally challenging to meet these properties. Often, there is only a certain probability to get single photon per trigger. In many cases, we get no photon or multi-photons. Therefore, the emission properties need to be precisely characterized with regard to the single-photon character.
2.2
Characterization of single-photon emission
In order to measure whether the light source emits single or multiple photons, the Hanbury Brown and Twiss experiment may be performed[25]. The setup is shown in Fig. 3. The photons are sent to a non-polarizing 50 : 50 beam splitter. Two single-photon detectors (D1 and D2) are placed at the two outputs of the beam splitter and measure the photons from each output. The two detectors are connected to a correlation device which determines the time delay between the detection events. The recorded data can be displayed in a histogram, showing the second-order correlation function of the single-photon emission:

class="figure_img" id="Figure3"/>
Download
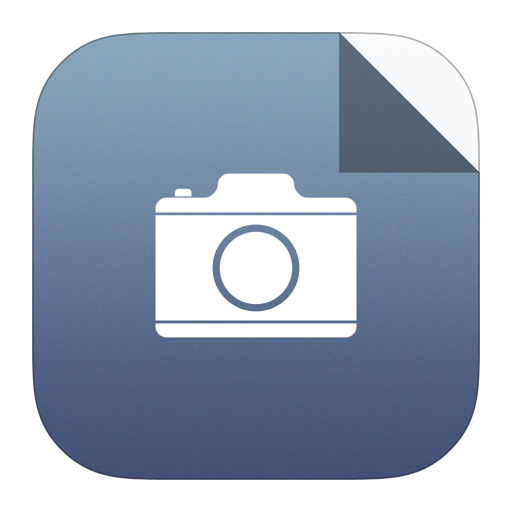
Larger image
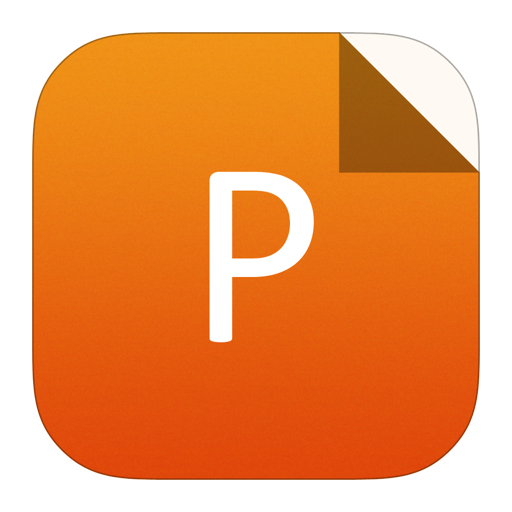
PowerPoint slide
Figure3.
(Color online) Scheme of Hanbury Brown and Twiss setup for autocorrelation measurement. The beam of light is sent to a beam splitter with single-photon detectors at the two outputs. An electronic correlator then determines the time delay between the two detector signals. Single photon emission results in the absence of simultaneous detection events on D1 and D2, in contrast to the case of multi-photon emission. Reprinted with permission from Ref. [24]. (Springer Nature)
${g^{left( 2 ight)}}left( tau ight) = frac{{{{leftlangle {{n_1}left( t ight){n_2}left( {t + tau } ight)} ight angle }_t}}}{{{{leftlangle {{n_1}left( t ight)} ight angle }_t}{{leftlangle {{n_2}left( {t + tau } ight)} ight angle }_t}}},$ ![]() |
where
ight)$

ight)}}left( 0
ight)$


class="figure_img" id="Figure4"/>
Download
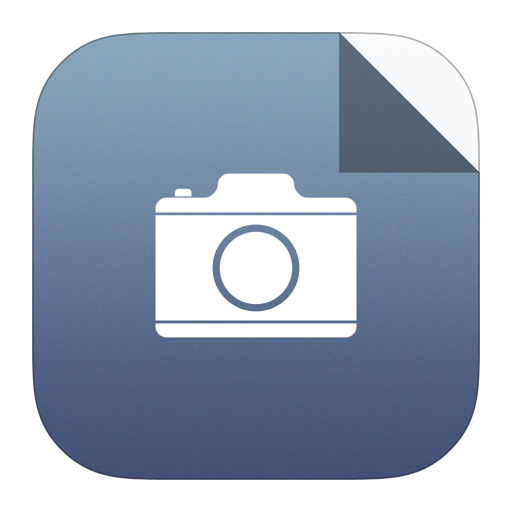
Larger image
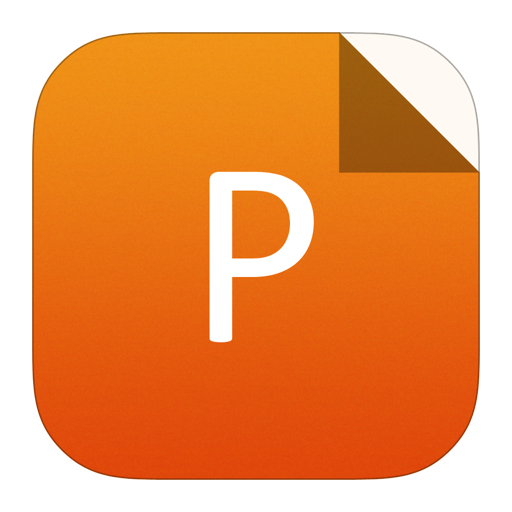
PowerPoint slide
Figure4.
(Color online) Second order correlation function measured under two types of optical excitations. (a) Continuous wave excitation. (b) Pulsed excitation.
3.
Telecom wavelength single-photon sources
Compared with single photon sources emitting at the visible wavelength range, few research has been conducted on telecom sources till now. But due to their potentials in application, telecom wavelength single-photon sources are becoming more and more attractive. Table 1 lists different types of such single photon sources.
Material system | Operating temperature | g(2)(0) | Ref. |
Atomic sources | Room temperature | 0.06 | [26] |
Parametric down conversion | Room temperature | 0.001 ± 0.0003 | [27] |
InAs quantum dots | Cryogenic temperature | 0.00044 ± 0.00002 | [28] |
Silicon Carbide | Room temperature | 0.05 ± 0.03 | [29] |
Carbon nanotubes | Room temperature | 0.01 | [30] |
Gallium Nitride | Room temperature | 0.05 ± 0.02 | [31] |
Table1.
Summary of six types of single photon sources emitting at telecom wavelength.
Table options
-->
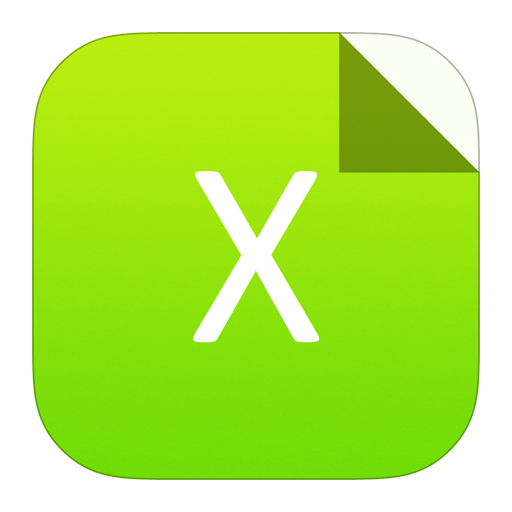
Download as CSV
Material system | Operating temperature | g(2)(0) | Ref. |
Atomic sources | Room temperature | 0.06 | [26] |
Parametric down conversion | Room temperature | 0.001 ± 0.0003 | [27] |
InAs quantum dots | Cryogenic temperature | 0.00044 ± 0.00002 | [28] |
Silicon Carbide | Room temperature | 0.05 ± 0.03 | [29] |
Carbon nanotubes | Room temperature | 0.01 | [30] |
Gallium Nitride | Room temperature | 0.05 ± 0.02 | [31] |
3.1
Atomic sources
Atomic cascade transitions were the first experimentally realized single photon sources[8]. According to quantum mechanics, single atoms have many discrete energy levels. The transition between each two levels correspond to a certain energy (or photon wavelength, from ultraviolet to infrared range). Many of these transitions emit photons in the visible wavelength range[32–34]. However, in the last decade a lot of efforts were put on erbium ions[35], atomic rubidium, cesium, sodium, potassium as well as atomic ensembles[36]. The erbium ion 4I13/2 to 4I15/2 transition can emit photon with wavelength at 1.5 μm while rubidium and caesium atomic transitions can span the whole telecom O-band and C-band.
By using two lasers to pump the hot rubidium vapour cell, a cascade transition will occur and two single photons will be generated, one with short wavelength at 780 nm and the other within the telecom O-band[26, 37] (Fig. 5). After filtering one wavelength, single photon emission at either the telecom or visible region can be obtained. Since the two photons are emitted one after another, one of the photons can act as a heralding signal for the other single photon emission. With such a cascade transition, the polarization state of the first emitted photon is correlated with the second photon polarization such that it results in polarization entanglement[26]. Determining the polarization state of one photon will simultaneously fix the second photon’s polarization, which Einstein referred as ‘a spooky action at a distance’. In addition, thanks to the long storage lifetime (milliseconds) of atomic ensembles[38], it is also a very good candidate for quantum memory application[39–41]. However, their low radiative rates restrict the brightness, and the limited trapping time could induce decoherence[42]. The required optical setups for trapping atoms in cavities limit the scalability of these type of single photon sources.

class="figure_img" id="Figure5"/>
Download
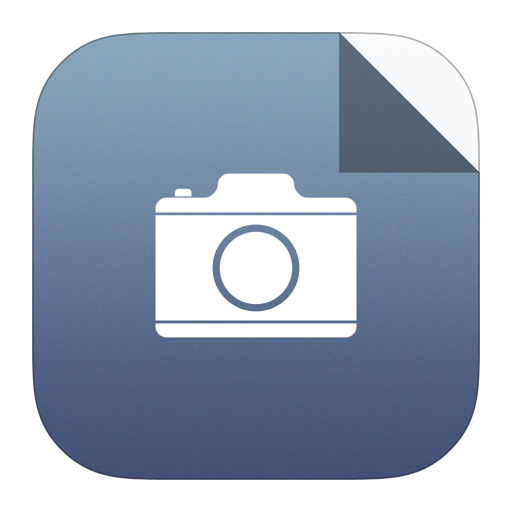
Larger image
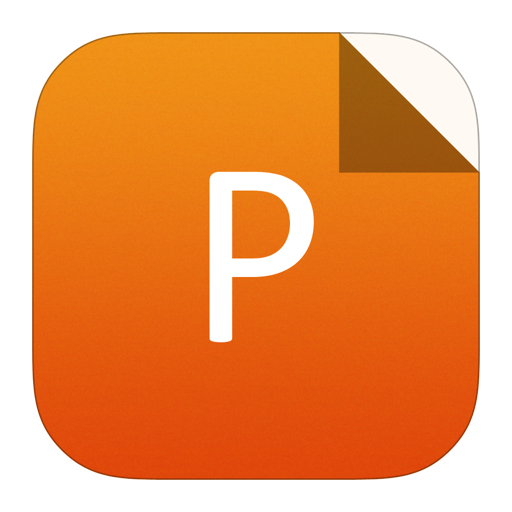
PowerPoint slide
Figure5.
(Color online) (a) Scheme of single photon emission from Rb atoms. Two pumping lasers are needed for Rb excitation (795 and 1324 nm), and two wavelength of single photons will be emitted (780 and 1367 nm). (b) The configuration of Rb energy levels. Reprinted with permission from Ref. [26]. (The Optical Society)
3.2
Spontaneous parametric down conversion
Till now, the most widely studied and used single-photon emitters are based on spontaneous parametric down conversion (SPDC). It was first experimentally achieved in 1970[43, 44]. By guiding a strong pump laser light on a second-order nonlinear crystal, e.g. β-barium borate (BBO) or a periodically poled lithium niobate (PPLN) waveguide, with a certain probability, we can obtain two beams of light with lower energy, called signal and idler[45–47]. The signal and idler photons should meet the phase matching requirement:
m{p}}} = {omega _{
m{s}}} + {omega _{
m{i}}}$

m{p}}} = {{{q}}_{
m{s}}} + {{{q}}_{
m{i}}}$


class="figure_img" id="Figure6"/>
Download
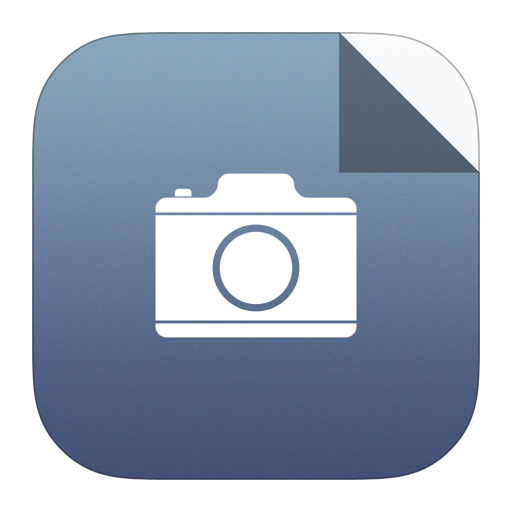
Larger image
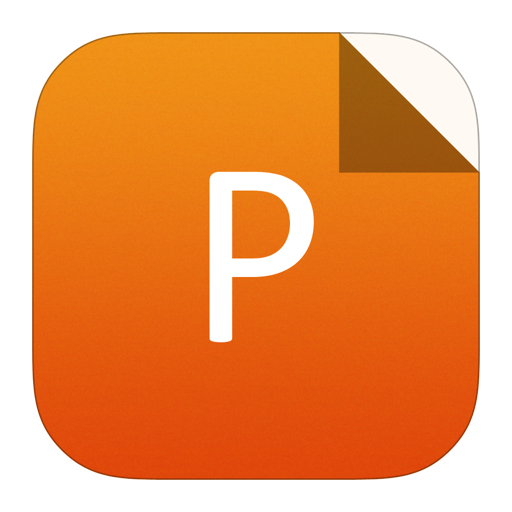
PowerPoint slide
Figure6.
(Color online) (a) Scheme of SPDC process and phase matching. (b) High purity of single photon emission. The dots are experiment data, the blue and red curves are theoretical fitting with and without considering detector noise. The inset is g(2)(0) = 0.001. Reprinted with permission from Ref. [27]. (The Optical Society)
However, SPDC is not an ‘on-demand’ single-photon source. After the pump laser passes the nonlinear crystal, single photon emission can only be obtained with a certain probability. For different pump powers, either multiple photons will be emitted, or no photons are generated at most of the time. Although post-selection can ensure high single-photon emission
ight)}}left( 0
ight) = 0.001 pm 0.0003}
ight)$

Recently, an ultra-fast single photon source at telecom wavelength has been realized[60]. The scheme is shown in Fig. 7. The wavelength of photons emitted by a pulsed telecom laser with 10 GHz repetition rate is doubled by second harmonic generation (SHG). The photons then pump the SPDC non-linear crystal. Idler photons with wavelength 1543.73 nm are selected by a dense wavelength demultiplexer (DWDM) and sent to a Hanbury Brown-Twiss setup, while the signal photons with wavelength 1537.40 nm are selected by a narrow-band Bragg filter (FBG) and trigger the avalanche photodiode (APD) to herald the generation of idler photons. With the help of such ultra-fast telecom laser and non-linear optics, heralded rate of single photons at 2.1 MHz and g(2)(0) of 0.023 has been obtained.

class="figure_img" id="Figure7"/>
Download
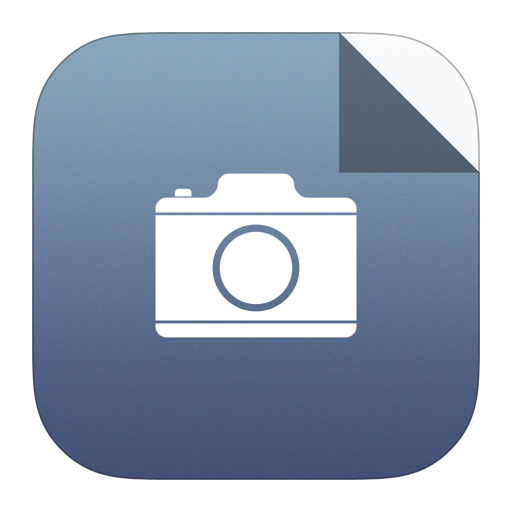
Larger image
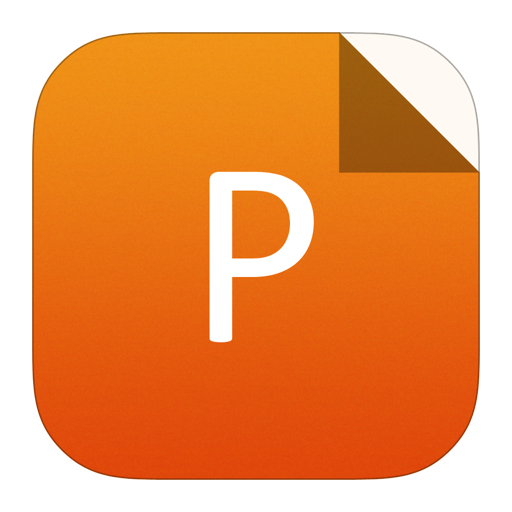
PowerPoint slide
Figure7.
(Color online) (a) Scheme of experimental setup of ultra-fast heralded single photon source. (b) Selected signal photons (ITU 50) and idler photons (ITU 43) from the SPDC spectrum (black line). Reprinted with permission from Ref. [60]. (John Wiley & Sons)
3.3
Semiconductor quantum dots
Another type of well-studied single photon source is epitaxially grown semiconductor QDs. Comparing with SPDC source, QDs can achieve on-demand and therefore bright single photon emission. When a QD is excited, it will emit one single photon at a time, which is very attractive for practical applications. Furthermore, QDs can be electrically triggered[60] and are scalable due to compatibility with semiconductor technology. There are various materials systems that have been investigated so far: GaAs/GaAs[61–63], InAs/GaAs[64–67], InP/InGaP[68], InP/GaP[69, 70], InGaP/GaP[71], InGaAs/GaP[72], InGaAs/GaAs[73, 74], and more. However, due to the relatively wide band gap, almost all these material systems can only emit photons at the visible to near infrared range. Although bulk InAs has a very small band gap, the emission from InAs QDs is significantly blue-shifted[75] when grown on a GaAs substrate, due to strong strain caused by large lattice constant mismatch.
The general structure of QDs systems is shown in Fig. 8. On the substrate, the QDs layer is sandwiched between two barrier layers. The QDs have smaller band gap so that the excited electron-hole pair can be confined in the QDs. One method to achieve telecom wavelength in the InAs/GaAs system is to grow a thick InGaAs metamorphic buffer layer between GaAs substrate and the InAs QDs[76]. With linearly increasing the In content from bottom to top, the lattice mismatch between InxGa1–xAs and InAs will be reduced and therefore the emission wavelength can be red-shifted to the telecom C-band[77]. The second method is to use InxGa1–xAs strain reducing layer[78]. After depositing the QDs, an InxGa1–xAs layer is used to cover the QDs instead of capping with GaAs. The lattice constant of InxGa1–xAs is larger than GaAs so that the strain on QDs is reduced. With the help of InxGa1–xAs strain reducing layers, not only InAs QDs, but also InGaAs QDs can achieve telecom wavelength emission[78–83]. The third method is to use an InP substrate. Comparing with GaAs, the lattice mismatch between InAs and InP is much smaller. Without metamorphic buffer layers or strain reducing layers, this type of InAs QDs can emit single photons at telecom C-band[28, 84–88]. However, the lattice constant of InP is much larger than silicon, making it more difficult to combine with the mature silicon technology. It is both expensive and brittle, and it is hard to grow distributed Bragg mirrors on InP wafers. Due to the small band gap of InAs as well as shallow confining potentials, the QDs can only emit single photons at very low temperature. Under cryogenic temperature and resonant pumping, very pure single photon emission with g(2)(0) = 4.4 × 10–4 can be obtained[28]. Increasing the temperature results in a decreasing band gap of the QDs, leading to a red-shifted emission. However, more phonons will be excited at high temperature, causing photoluminescence peak broadening and degradation of the single photon emission[80]. Although we can still observe single photon emission up to 80 K, the purity is decreased significantly, with g(2)(0) = 0.34[88].

class="figure_img" id="Figure8"/>
Download
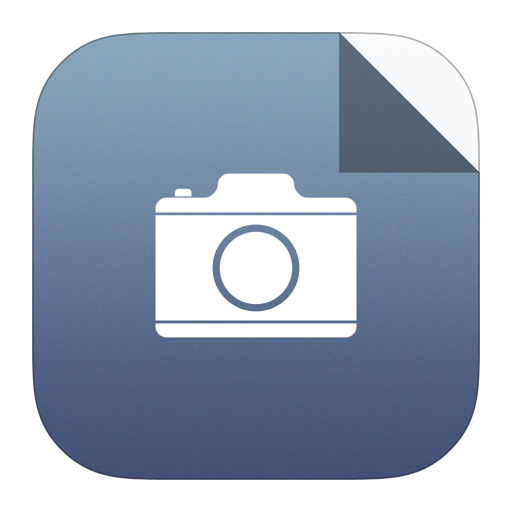
Larger image
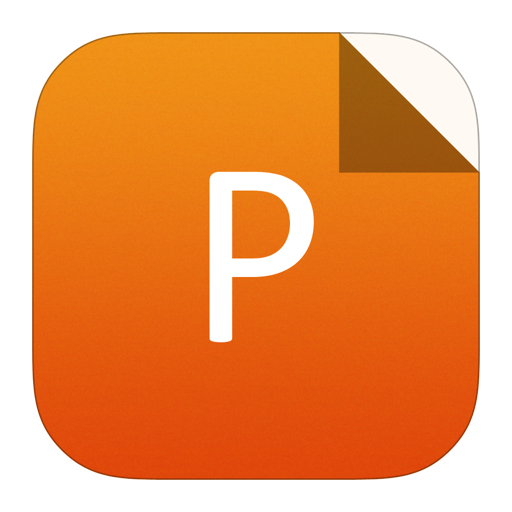
PowerPoint slide
Figure8.
(Color online) Three types of QDs grown epitaxially on GaAs and InP substrate, respectively. Reprinted with permission from Refs. [77, 81, 84]. (AIP Publishing)
Epitaxial QDs are usually grown by metal-organic vapour phase epitaxy or molecular beam epitaxy. The size of individual QDs is not perfectly identical, resulting in a distribution of emission wavelengths. This is detrimental for experiments requiring multiple sources of single photons, e.g. for quantum repeaters. Identical wavelengths are required to ensure the indistinguishability of photons from separate emitters. There are many ways to tune the wavelength, such as using electric field[89], magnetic field[90], strain[91–93], mainly demonstrated on non-telecom GaAs or InGaAs QDs. However, due to the difficulty to grow high quality telecom wavelength InAs QDs, very few research on wavelength tuning of InAs QDs has been reported. Till now, to the best of our knowledge, only very few group reported to use strain field to tune single photon emission at 1.55 μm with tuning range of 0.25 nm[94].
One challenge for single photon sources based on QDs is the extraction efficiency. The employed semiconductors have high refractive indices so that most of the photons are trapped in the material due to total internal reflection. Many structures are studied to enhance the extraction efficiency, such as micro-pillars[82], photonic crystals[95, 96], solid immersion lens[97], and more. However, these methods are mainly focused on GaAs-based material system emitting below 1 μm. In the case of InAs/InGaAs QDs emitting at 1.3 and 1.55 μm on InP substrate or InGaAs metamorphic buffer layer, not many fabrication techniques have been reported. Theoretically, numerical simulations showed that a cylindrical or cuboidal mesa structure with InP/AlGaInAs distributed Bragg reflector can enhance the extraction efficiency to above 25%[98]. While experimentally, extraction efficiency of 10% with InGaAs/GaAs QDs integrated into disc-shaped mesas has been reported[99]. And by using an optical horn structure, the extraction efficiency of 11% can be achieved[87]. An InAs/InP quantum dots in photonic crystal cavity can further improve the efficiency to 36%[100], which is very promising for future applications.
3.4
Defects in silicon carbide
Silicon Carbide (SiC) is a wide band gap semiconductor. There are three major polytypes: 3C-, 4H- and 6H-, with band gaps 2.36, 3.23, 3.05 eV, respectively. For studying the optical and magnetic properties, the defects in SiC are more attractive. The divacancy in 4H-SiC and carbon vacancy-antisite pair in 6H-SiC showed near infrared photon emission[101, 102], very close to the telecom wavelengths.
Recently, the single photon emission in 3C-SiC at telecom wavelength range has been reported[29]. The SiC membrane was grown epitaxially on a silicon substrate. Intrinsic defects in the 3C-SiC epitaxial layer can act as the single-photon emitters, as shown in Fig. 9. The center wavelength range of different emitters is between 1080 to 1265 nm. Such a wavelength shift (from 1080 to 1265 nm) might be caused by stacking faults in the SiC membrane. The second-order correlation function
ight)}}left( 0
ight) = 0.05 pm 0.03$


class="figure_img" id="Figure9"/>
Download
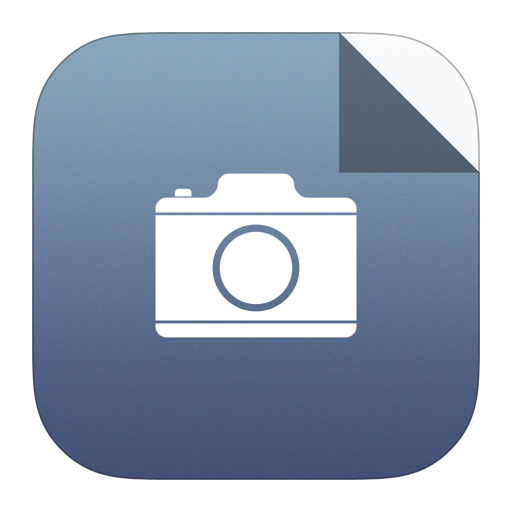
Larger image
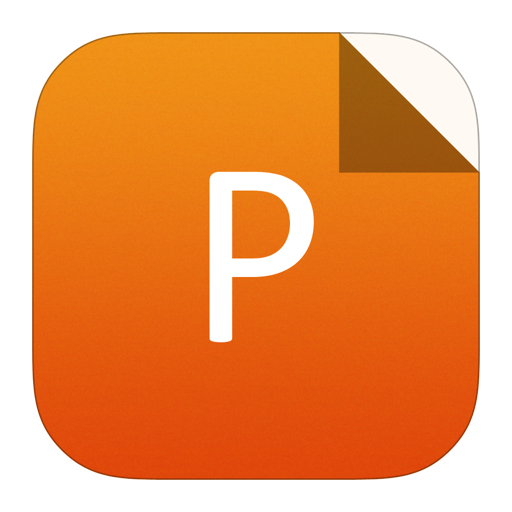
PowerPoint slide
Figure9.
(Color online) (a) Confocal map of four single photon emitters in 3C SiC epitaxy layer. (b) Room temperature photoluminescence spectra of three representative single photon emitters. (c) Second order autocorrelation measurement of the single photon emission. Reprinted with permission from Ref. [29]. (Springer Nature)
3.5
Carbon nanotubes
Carbon nanotubes have been widely studied since more than two decades ago due to their extraordinary electrical, mechanical and thermal properties[103]. After the first observation of photon antibunching in the photoluminescence measurement of a single wall carbon nanotube (SWCNT)[104], this material system became an attractive candidate for single photon sources. SWCNT is one-dimensional nanomaterial, the excitons in SWCNT are confined in two dimensions but can diffuse along the length of the nanotube[105]. To be an efficient single photon emitter, a zero-dimensional trap is required. Such a trap can be induced by the attachment of some chemical functional groups then the exciton is localized in the trap and emits single photons at cryogenic temperatures[106]. Owing to distinct lattice structures and diameters of the nanotubes, the wavelength of the single photons from undoped SWCNT can vary from around 880 to around 1140 nm[107]. With oxygen doping of the nanotubes, the wavelength can be further red-shifted to more than 1200 nm[107–109].
In addition, by introducing ether-d and eposide-I groups as the oxygen dopants to SWCNT, a deep trap state will be created below the band-edge excitons (E11 excitons), as shown in Fig. 10[106]. Compared to the undoped SWCNT, the wavelength of the doped SWCNT can be shifted to 1300 nm, the telecom O-band. However, the single-photon emission is relatively stable at cryogenic temperature, photoluminescence bleaching and intensity fluctuations occur when the nanotubes are placed on other solid-state substrates at higher temperatures up to room temperature[109]. In order to solve this issue, SWCNTs are encapsulated in a SiO2 matrix on silicon so that the dopants are solitary from substrate and environment influences. With such structure, single photon emission is still possible at room temperature, with g(2)(0) = 0.32[102]. This is also compatible with the current silicon technologies.

class="figure_img" id="Figure10"/>
Download
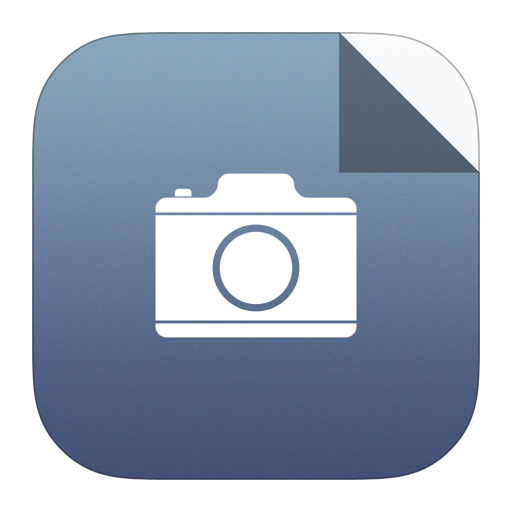
Larger image
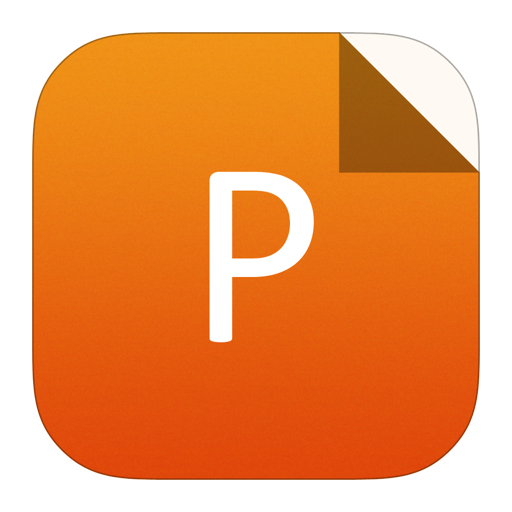
PowerPoint slide
Figure10.
(Color online) (a) Upper panel: Scheme of single wall carbon nanotube with oxygen-doping (Ether-d and Epoxide groups). Lower panel: Trap energy levels at doped areas. Oxygen-doping creates deep trap states below the E11 state of the nanotube, leading the localization of excitons near the doping sites. (b) Photoluminescence wavelength distribution of undoped and doped nanotubes. Reprinted with permission from Ref. [106]. (Springer Nature)
In order to further shift the single-photon emission to telecom C-band, the aryl


class="figure_img" id="Figure11"/>
Download
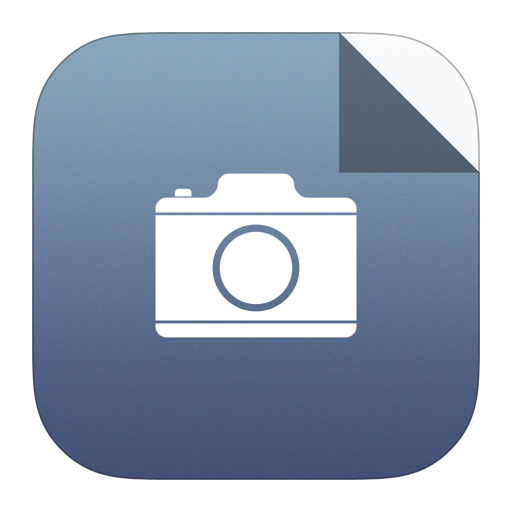
Larger image
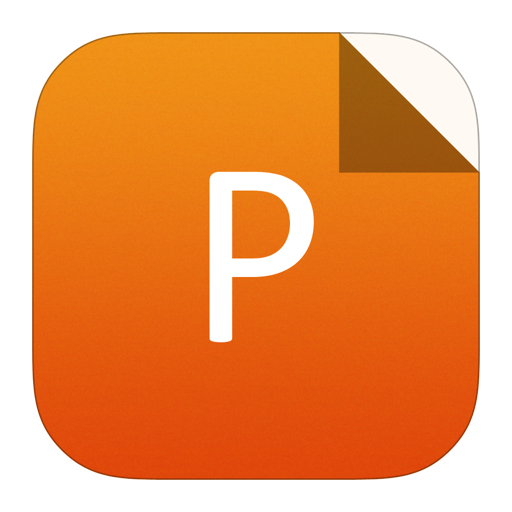
PowerPoint slide
Figure11.
(Color online) Photoluminescence spectra of two types of aryl-functionalized carbon nanotubes with different chiralities ((a) (6,5), (b) (7,5), (c) (10,3)) and their corresponding second-order correlation function. Reprinted with permission from Ref. [30]. (Springer Nature)
4.
Applications of single photon sources
Single photon sources have many applications. The most important and practical application for telecom wavelength single photon sources is quantum key distribution thanks for the low transmission loss in optical fiber and free-space. Quantum teleportation is also an important part among quantum technologies. Till now, the main workhorse of single photon source is the SPDC source.
4.1
Quantum key distribution (QKD)
After Bennett and Brassard’s proposal in 1984[4], many researches are focused on experimental realization of QKD and its distance-enhancement. Details of QKD has been reviewed in Ref. [3]. In the last few years, some big steps for commercial QKD applications have been achieved. The big challenge of free space QKD in the daytime is to collect signals from strong solar radiation. By using telecom wavelength single photon source and with the development of fiber-coupling technology and single photon detector, over 53 km QKD in daylight was demonstrated with total channel loss around 48 dB[21]. Comparing with atmosphere noise, the noise in the outer space is much lower. In 2016, the quantum satellite Micius was launched in China, orbiting at an altitude of 500 km around earth. QKD between ground and outer space was firstly distributed over 1200 km, with a kilohertz key rate[110]. For daily applications, the most efficient way to transmit information is to use optical fibers. QKD with key rate of 1.26 Mbit/s over 50 km was implemented in 2014[111]. Two years later, the transmission distance was enhanced one order higher, to 404 km, though the key rate was decreased to 1.16 bit/h[112]. With the help of twin-field QKD scheme, QKD over 505 km with key rate about 1 bit/s was achieved[113]. However, the loss in the optical fiber channel is still very high. This may be circumvented by the implementation of proposed quantum repeaters[110]. A quantum repeater is used to restore and amplify the information that people want to send. It is an essential component for long-distance quantum computation. A practical way for long-haul QKD is to combine free space and fiber-based transmission. In 2018, an intercontinental QKD between China and Austria was illustrated (Fig. 12)[114]. The total distance was over 7600 km, which paves the way towards global quantum communication.

class="figure_img" id="Figure12"/>
Download
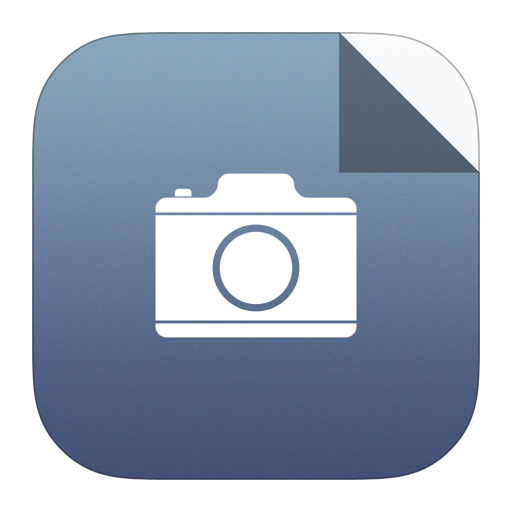
Larger image
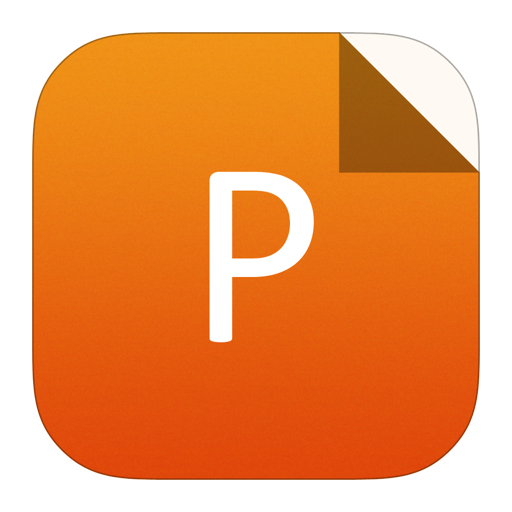
PowerPoint slide
Figure12.
(Color online) Illustration of satellite based QKD among three ground stations (Xinglong, Nanshan and Graz). Reprinted with permission from Ref. [114]. (American Physical Society)
4.2
Quantum teleportation
Entanglement is a very important mechanism in quantum mechanics. If two photons are entangled, the state of one photon cannot be treated independently from the state of the other photon. Entangled photon pairs can be generated directly by SPDC or cascade transitions in QDs or atoms. They can also be created by performing Bell state measurements on single photons. With entangled photons and single photons, quantum teleportation has been realized.
Quantum teleportation was first experimentally verified by teleporting the polarization of one photon to another photon in the lab[115]. Quantum teleportation is another way for quantum communication. In QKD, the sender (Alice) needs to send the qubits to the receiver (Bob) for communication. However, in teleportation, Alice doesn’t need to directly send the qubits to Bob. An entangled photon pair is needed and one of the photon pair will be sent to Alice, while the other will be sent to Bob. Next Alice will perform a Bell state measurement on that photon and the photon carrying the information she wants to teleport. Then by doing unitary transformation on the photon that Bob receives, Bob can reconstruct the qubit which Alice wants to send.
Quantum teleportation was first experimentally verified by teleporting the polarization of one photon to another photon in the lab[116]. While quantum teleportation is meant for long-distance communication and as an important ingredient for global quantum networks, it is necessary to increase the teleportation distance. With metropolitan optical fiber, over 10 km quantum teleportation has been achieved in the city[117, 118]. In free space, the teleportation distance can be enhanced to 100 km (Fig. 13)[119, 120]. With the launch of Micius satellite, up to 1400 km quantum teleportation has been performed, which is a crucial step towards global quantum network[121].

class="figure_img" id="Figure13"/>
Download
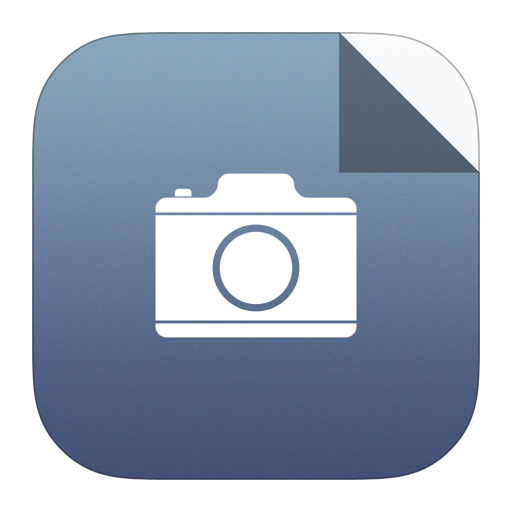
Larger image
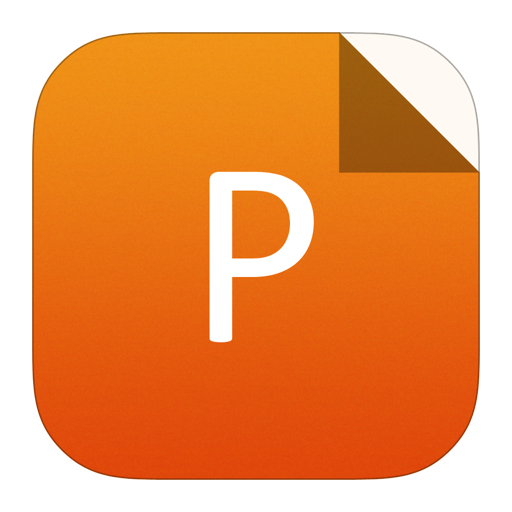
PowerPoint slide
Figure13.
(Color online) (a) Bird’s-eye view of experiment site in China. (b–d) Illustration of the entangled photon pair generation and distribution from Charlie, single photon state preparation and Bell state measurement from Alice and single photon state reconstruction from Bob. Reprinted with permission from Ref. [119]. (Springer Nature)
5.
Conclusion and outlook
Single photon sources, especially at telecom wavelengths, are important constituents for quantum communication networks. Although SPDC sources have matured and are commonly used, there is a fundamental limit to its brightness. In contrast, semiconductor QDs already show great potential as bright single and entangled photon sources. Although some challenges remain to be solved, such as low extraction efficiency and relatively low photon indistinguishability, it has become the major competitor of SPDC sources. Other telecom wavelength single photon sources are being explored and yet requiring a substantial amount of research to reveal their potential for future applications.
In order to implement telecom wavelength single photon sources in real-world scenarios, electrically driven devices with small footprints are necessary. Promising QD-based photon sources have been already realized[122] which are compatible with current semiconductor technology. Another direction for quantum applications is to improve the performance of single photon sources. For experiments involving multiple sources, single photon emission with identical wavelength is required. Strain tuning and active frequency feedback are successfully applied for GaAs QDs[92, 123], and may also be useful for QDs emitting at telecom wavelength. Using resonant excitation schemes or implementing QDs in microcavities can also improve the indistinguishability of the photons[124]. Enhance the extraction efficiency of QDs equally important: Some proper methods may include integrating single photon sources into cavities or coupling with solid immersion lenses[97, 125]. To have a close-to-perfect single photon source at telecom wavelength is a milestone towards real quantum communications.
Acknowledgement
The work was financially supported by the ERC Starting Grant No. 715770 (QD-NOMS) and the National Natural Science Foundation of China (No. 61728501).