1.
Introduction
Multicrystalline silicon (mc-Si) is attracting increasing attention owing to its high efficiency and low cost[1–4]. However, because of the presence of grown-in structural defects, the properties of mc-Si solar cells are not as good as those of Czochralski silicon (CZ-Si) cells. Metal impurities, especially Fe-related impurities are also key factors to solar cell performance[5–9]. For instance, mc-Si contains high concentrations of iron, typically in the range of 1014 to 1016 cm?3. Iron impurities in mc-Si are mostly precipitated at structural defects like grain boundaries and dislocations, with only ~1012–1013 cm?3 existing as an interstitial state or Fe–B complexes after crystal growth, considering the very low solubility of Fe in Si lattice[10–13]. The combined effect of impurities and structural defects usually induces much more carrier recombination centers in mc-Si compared to CZ-Si, so that the minority carrier lifetime and solar cell performance of mc-Si solar cells is reduced compared to CZ-Si cells.
The key advantage of mc-Si photovoltaic materials is its low cost. To date, the manufacturing cost-per-watt of the casting method is much cheaper compared to that of the Czochralski method. Therefore, the yield of mc-Si ingot becomes very important and sensitive. However, macroscopic shadows are always observed in mc-Si ingots after solidification by infrared (IR) test, as shown in Fig. 1(a). Industrial experiences have shown that these shadows can cause degradation in solar cell efficiency. It was previously believed that the shadow consists of microcrystals, where massive extremely small grains are formed because of the constitutional supercooling effect induced by the segregation of various impurities[14–16]. Thus, the boundaries of the microcrystalline grains should have high impurity concentration. These impurities absorb infrared light, leading to IR shadows in the scanning infrared microscopy (SIRM) imaging system, whereas our experience has shown that not all the shadows are caused by microcrystalline grains. In many cases, the grains penetrating the shadows are continuous, that is, no microcrystalline structures are formed and found. The composition of this kind of strip-like shadow and its impact on wafer quality and cell performance have not yet been elucidated.
In this work, a strip-like shadow formed without cracks or microcrystals in mc-Si ingot was investigated using scanning infrared microscopy (SIRM), photoluminescence imaging (PL), laser scanning confocal microscopy (LSCM), field-emission scanning electron microscopy (SEM), X-ray energy-dispersive spectrometry (EDS), and microwave photoconductance decay (μ-PCD). The effect of these shadows on the solar cell efficiency was also investigated. The results indicated that these shadows were composed of scattered Fe–O–C precipitates, which reduced minority carrier lifetime slightly but cell efficiency severely, mainly due to the degradation in series and shunt resistance.
2.
Experimental
In this study, industrial p-type 850 kg mc-Si ingots were fabricated. The distribution and recombination behavior of strip-like shadows found in the cut block were characterized using SIRM (IRB-50, Semilab), PL imaging (LIS-R1, BT Imaging), and μ-PCD (WT-2000, Semilab). Then, the block was chemically polished using HF/HNO3 (1 : 3) and observed using LSCM (LSM-700, Zeiss) and field-emission SEM (Sigma, Zeiss), and the impurity points were characterized through EDS analysis. Then sliced wafers at and near the shadow were characterized by PL mapping to investigate the horizontal distribution of recombination-active defects. Finally, wafers with/without strip-like shadow defects were fabricated into standard screen-printed aluminum back surface field (Al-BSF) solar cells, and the performance parameters were given and analyzed.
3.
Results and discussion
Generally, strip-like shadows in mc-Si are found through routine nondestructive scanning infrared imaging measurements. Fig. 1 shows the SIRM and PL images of the vertical cross-section of an as-cut block with strip-like shadow. In Fig. 1(a), the shadow can be seen clearly, and a precipitate-like shadow structure is found above the strip. It was previously considered that shadows in IR mapping usually reflect the formation of invisible cracks or microcrystalline grains. However, PL mapping of the same block has shown that the grains grow continuously across the shadow region, as shown in Fig. 1(b). Despite the fact that PL spectroscopy can only detect defects within the maximum depth of ~10 μm, and that infrared light transmits through the whole ingot, reflecting the overall absorption caused by the defects, it can be inferred from Fig. 1(b) that the defects causing this strip-like shadow in the SIRM image have little effect on the grain structure, in other words, the strip-like shadow is not caused by invisible cracks or microcrystals.
Adjacent wafers next to and at the shadow region were taken to perform PL mapping, which are the referential wafer A and defective wafers B1 and B2, as shown in Fig. 1(b). Fig. 2 shows the 156 × 156 mm2 PL images of these wafers. Note that there is no obvious difference in grain structure between the 3 wafers, supporting that the strip-like shadow is not caused by cracks nor microcrystals. Meanwhile, many recombination centers shown as “black spots” can only be found in Figs. 2(b) and 2(c), which are not succeeded from the original dislocations shown in Fig. 2(a). These spots distribute discontinuously, in quite small sizes, as shown in the red boxes in Figs. 2(b) and 2(c), which does not fit the features of dislocation. Dark patterns in PL images usually refer to macroscopic dislocation clusters, where dislocations propagate continuously when observing adjacent wafers. In this work, it can be inferred that the black spots in the red boxes are impurity precipitates so that the size of the spot does not grow larger from Fig. 2(b) to Fig. 2(c).

class="figure_img" id="Figure1"/>
Download
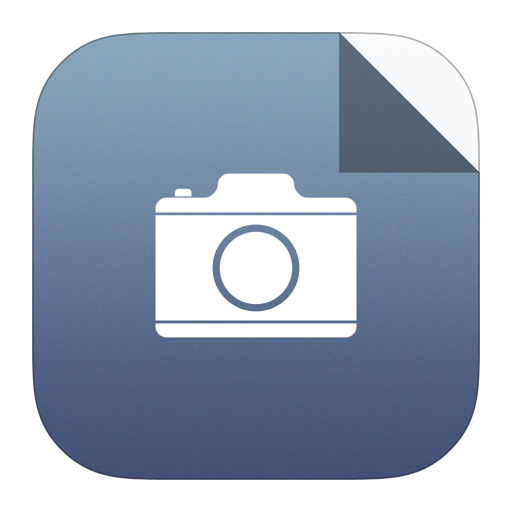
Larger image
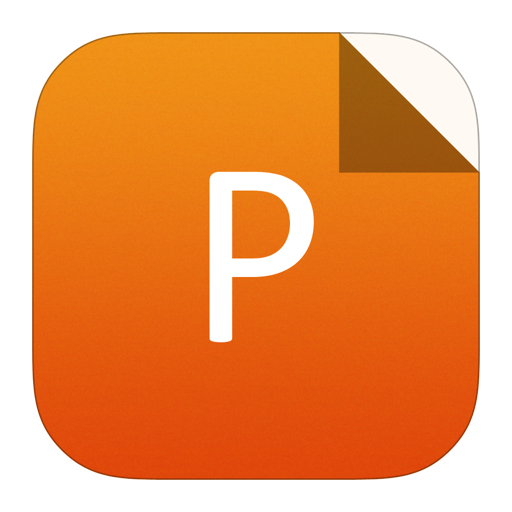
PowerPoint slide
Figure1.
(a) The scanning infrared microscopy (SIRM) and (b) photoluminescence (PL) images of the cross-section of a mc-Si ingot with strip-like shadow.
We consider whether metal impurity precipitates can be the reason for IR absorption. Table 1 shows the metal contents of samples from the referential sample A and defective sample B1 measured by inductively coupled plasma mass spectrometry (ICP-MS). The results show that the Fe content of the defective sample is approximately twice compared to the reference, indicating Fe-related impurities could be related to the defects. Microscopic photos of etched defective samples taken by (a, b) LSCM and (c) FE-SEM are shown in Fig. 3, the samples were etched under the same condition. Note that a row of black etch pits can be found in the defective sample in Fig. 3(a), the linear density is per several hundreds of micrometers. Higher-magnification images in Figs. 3(b) and 3(c) show that these pits are circular (or gourd-like when one pit superposes another), and a precipitate with a diameter of ~25 μm embedded in the etch pit is found as expected in Fig. 3(c). Table 2 shows the results of the elemental components of the precipitate analyzed by EDS, where position 1 is regular Si bulk next to the etch pit, position 2 and 3 are points selected inside the etch pit. Table 2 shows that the contents of impurity Fe at the center of the precipitate are higher, especially for sample point 2, whose primary components are the impurities Fe, O, and C. This is consistent with the results shown in Table 1, namely, that extra content of Fe exists in precipitates. Thus, it can be inferred that the shadow in the sample is composed of micro precipitates of Fe–O–C. It can be inferred that the precipitate is soluble in acidic solutions and its hardness is low, because this kind of precipitate has no obvious effect on the wafer slicing process according to our observation.
Sample | Fe | Cr | Ni | Cu | Zn | Ca | Mg | Al | Na | K | Total |
A | 44.90 | 2.47 | 1.11 | 365.26 | 3.13 | 9.76 | 3.63 | 5.97 | 2.43 | 16.02 | 454.68 |
B1 | 86.24 | 2.99 | 1.14 | 353.53 | 2.48 | 9.55 | 4.62 | 6.90 | 2.94 | 22.67 | 493.06 |
Table1.
Metal impurity contents of normal sample A and defective sample B1 (ppbw).
Table options
-->
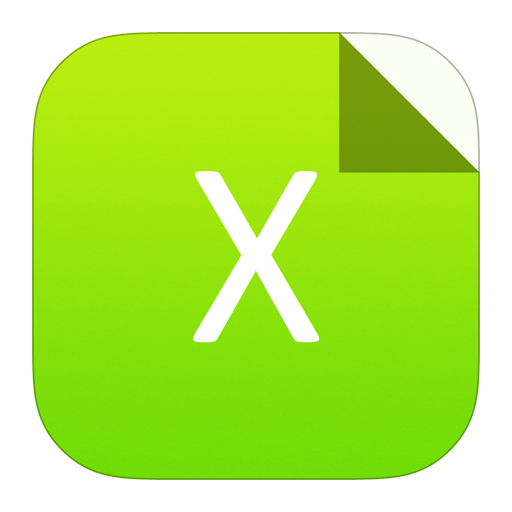
Download as CSV
Sample | Fe | Cr | Ni | Cu | Zn | Ca | Mg | Al | Na | K | Total |
A | 44.90 | 2.47 | 1.11 | 365.26 | 3.13 | 9.76 | 3.63 | 5.97 | 2.43 | 16.02 | 454.68 |
B1 | 86.24 | 2.99 | 1.14 | 353.53 | 2.48 | 9.55 | 4.62 | 6.90 | 2.94 | 22.67 | 493.06 |

class="figure_img" id="Figure3"/>
Download
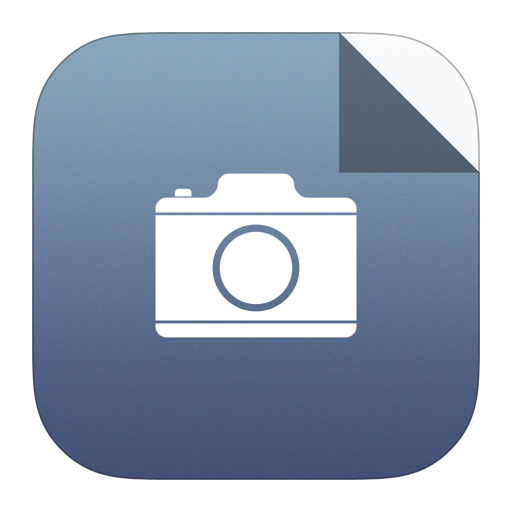
Larger image
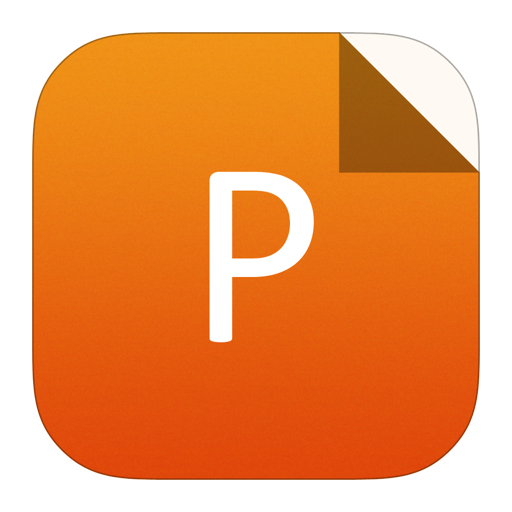
PowerPoint slide
Figure3.
(a) and (b) LSCM images and (c) FE-SEM images of etched defective sample.
Position | Si | B | C | O | Fe | Ca | K | N | Al | Mg |
1 | 85 | 10 | 1 | 1 | 1 | 1 | 1 | — | — | — |
2 | 1 | — | 19 | 20 | 51 | 4 | 3 | — | 1 | 1 |
3 | 35 | 11 | 5 | 8 | 24 | — | 13 | 1 | 2 | 1 |
Table2.
Results of EDS analysis of impurity points (wt%).
Table options
-->
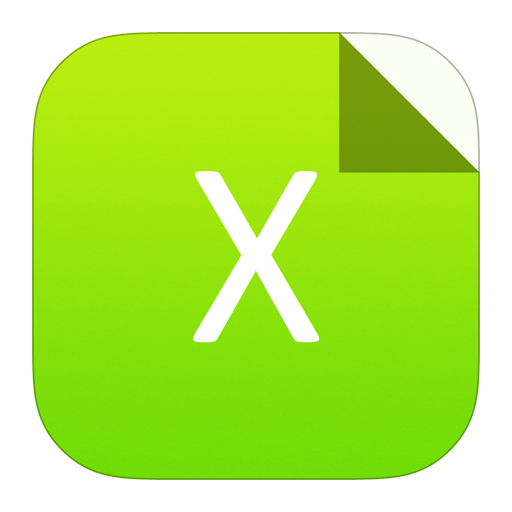
Download as CSV
Position | Si | B | C | O | Fe | Ca | K | N | Al | Mg |
1 | 85 | 10 | 1 | 1 | 1 | 1 | 1 | — | — | — |
2 | 1 | — | 19 | 20 | 51 | 4 | 3 | — | 1 | 1 |
3 | 35 | 11 | 5 | 8 | 24 | — | 13 | 1 | 2 | 1 |
Since the segregation coefficient of Fe in liquid Si is very small, the concentration of Fe keeps increasing and becomes supersaturated at the solid/liquid interface during the solidification. It can be inferred that both the temperature fluctuations and the entry of foreign matter can destroy the equilibrium state of Fe, resulting in instantaneous nucleation. These nuclei could lead to massive precipitation of other impurities such as O and C to form impurity particles. Thus, a laminar layer rich in these impurity particles is formed. Owing to the diffusion of these impurity particles in the silicon melt, similar flocculent precipitates of the impurities are formed above this enrichment layer[17–19]. Since the enrichment layer is usually a solid/liquid interface, the precipitates are usually formed at the grain boundaries, as shown in the red boxes in Fig. 2.
Interestingly, Fig. 3(b) shows that a few fine etch lines (shorter than 10 μm) are located around the gourd-like etch pit, indicating that the dislocations are formed around the precipitates. Inclusions or precipitates in Si caused inner stress during heat treatment, and the stress is usually released by generating dislocations. These fine lines can be the dislocation sources for the dislocation clusters found continuously in Figs. 2(b) and 2(c) (but not in 2(a)). Therefore, the phenomenon suggests that Fe–O–C precipitates can act as dislocation sources in mc-Si ingots. Fig. 4 shows the SIRM and μ-PCD images of the shadow region. The distribution and recombination behaviors can be determined through comparing Figs. 4(a) and 4(b). Obviously, the carrier lifetime of the “strip” is reduced compared to the perfect Si lattice, but much higher compared to the area with dislocation clusters, as shown in Fig. 4(b). Meanwhile, the propagation of the precipitates can be observed in Fig. 4(a), but the carrier lifetime is not obviously affected by the propagated precipitates. Therefore, it suggests that Fe–O–C precipitates do reduce the minority carrier lifetime, by much less compared to the effect of dislocation clusters. In addition, it should be clarified that the detection depth of μ-PCD is also shallow like PL measurements, so there is another probability that the recombination of inner precipitates shown in Fig. 4(a) cannot be reflected by the μ-PCD method, which needs further investigation.

class="figure_img" id="Figure4"/>
Download
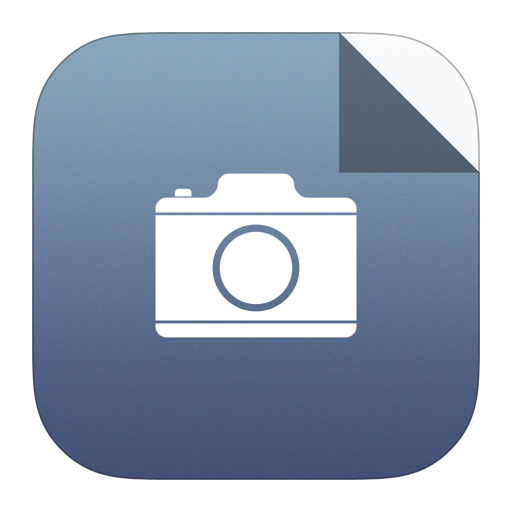
Larger image
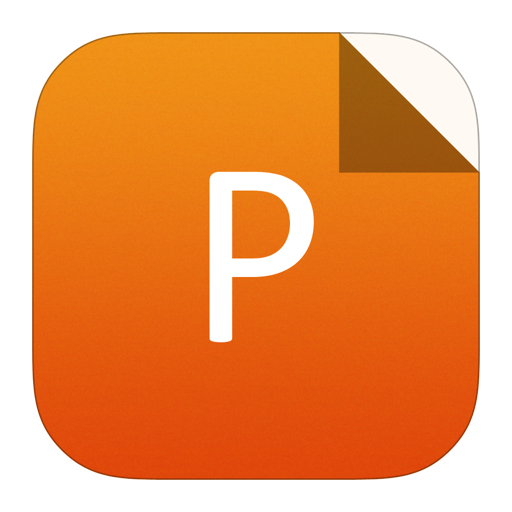
PowerPoint slide
Figure4.
(a) SIRM image. (b) μ-PCD image.

class="figure_img" id="Figure2"/>
Download
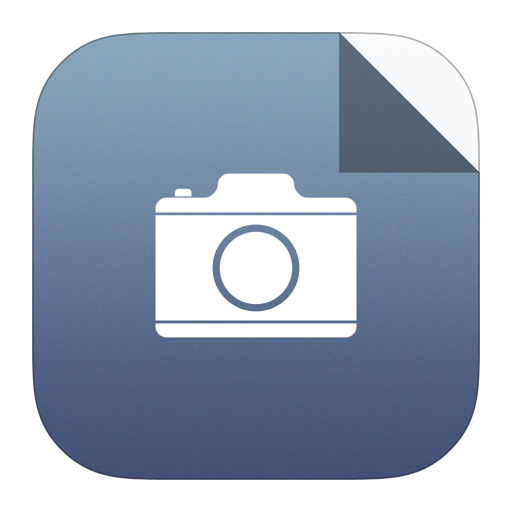
Larger image
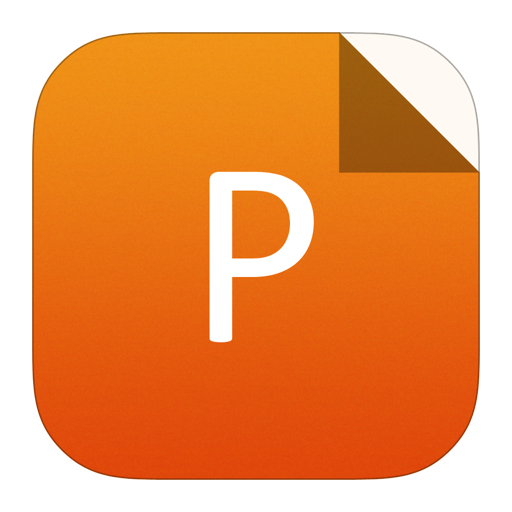
PowerPoint slide
Figure2.
PL images of (a) referential wafer A, and (b, c) two adjacent wafers B1 and B2 sliced at the shadow region.
The effect of Fe–O–C precipitates on solar cell performance is also evaluated. Table 3 shows the average performance parameters of solar cells based on referential and defective wafers. It is obvious that the average efficiency of the cells based on defective wafers was 2.26% (relatively 12.4%) lower, compared to that of the referential cells. Among the parameters, the average short-circuit current density (Jsc), open-circuit voltage (Voc) and fill factor (FF) of the defective cells are relatively 2.1%, 3.6%, and 7.1% lower, respectively. The precipitate-related decrease in minority carrier lifetime should be a reason for degradation in Jsc and Voc of the defective cells, however, it should be noticed that the difference in FF is the largest among the parameters, therefore the parameters of series and shunt resistance are measured and shown in Table 4. Both the average series and shunt resistance of the defective cells are worse compared to those of the referential cells, which causes extra degradation in FF of the defective cells. Moreover, worse series and shunt resistance will also decrease Jsc and Voc, respectively. The latter can be reflected by the reverse current Irev, the leakage current under reverse voltage. Among the defective cells, 21.73% exhibited an abnormally increased Irev, compared to the value of 0.62% for referential cells, which support that the defective wafers have much more metal-containing precipitates lying within recombination-active grain boundaries[20].
Sample | Jsc (mA/cm2) | Voc (mV) | FF (%) | η (%) |
Reference | 35.98 | 636.5 | 79.8 | 18.27 |
With shadow | 35.24 | 613.3 | 74.1 | 16.01 |
Table3.
The performance parameters of solar cells based on the referential and defective wafers. (~1000 pieces each group)
Table options
-->
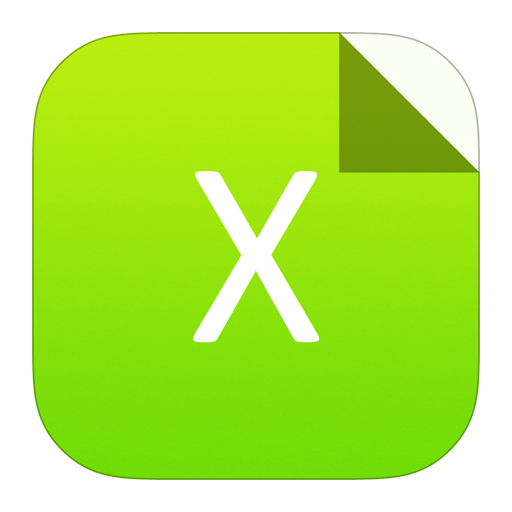
Download as CSV
Sample | Jsc (mA/cm2) | Voc (mV) | FF (%) | η (%) |
Reference | 35.98 | 636.5 | 79.8 | 18.27 |
With shadow | 35.24 | 613.3 | 74.1 | 16.01 |
Sample | Rs (mΩ) | Rsh (Ω) | Rate of Irev > 0.5 A |
Reference | 1.89 | 230 | 0.62% |
With shadow | 9.5 | 85 | 21.73% |
Table4.
The series, shunt resistance and the rate of high reverse current (Irev > 0.5 A) of the solar cells based on the referential and defective wafers.
Table options
-->
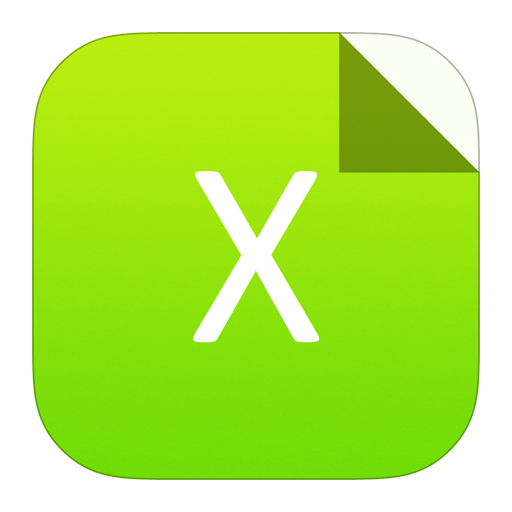
Download as CSV
Sample | Rs (mΩ) | Rsh (Ω) | Rate of Irev > 0.5 A |
Reference | 1.89 | 230 | 0.62% |
With shadow | 9.5 | 85 | 21.73% |
4.
Conclusions
The strip-like shadow in Si ingots was characterized and analyzed using FE-SEM, PL imaging, LSCM, EDS, and μ-PCD measurements. It was found that the shadow is mainly caused by Fe–O–C precipitates with a size of ~25 μm. These Fe–O–C precipitates are soluble in a mixed solution of hydrofluoric acid and nitric acid and do not affect wafer slicing. The Fe–O–C impurities at the strip-like shadow can slightly decrease the minority carrier lifetime but cause severe degradation in solar cell conversion efficiency by making worse series and shunt resistance.