1.
Introduction
In the past decades, Moore’s law was maintained by applying some new structures and materials, such as high-k materials to improve the ability of gate, fin structure to suppress the short channel effect, and strain silicon to improve carrier mobility. In large dimension of more than 100 nm, the dopant still plays a supporting role in current devices by just gaining or losing electrons. However, as the size of semiconductor devices continues shrinking, the random distribution of the individual dopant gradually has a significant impact on the device performance in which inhomogeneity should be supposed[1, 2]. Random dopant distribution induces the shift of threshold voltage, whose standard deviation can be quantified[3]. Also, transistors with ordered dopant arrays can improve threshold voltage reproducibility for metal oxide semiconductor field effect transistor (MOSFET)[4].
Meanwhile, the dopant atoms become individually discrete[5] and start to decide whether the current can flow through a transistor at the given gate, source and drain voltages. Different from single electron transistor with an artificial Coulomb island[6], single-dopant atom transistor[7, 8] is based on the control of individual dopant atoms. Besides, an approach to controlling the wave functions of individual atoms is proposed due to the hybridization of a single electron donor state between its nuclear potential and a nearby quantum well[9]. Furthermore, some investigations of silicon-based quantum computers utilize a single impurity atom[10, 11], particularly a single phosphorus atom for a spin-to-charge conversion.
Until now, the probing of single dopants at the atomic level[12, 13] and the atomically precise placement of individual dopants[14] have been achieved, which can provide fundamental study on the characteristics and behaviors of few-dopant systems[15, 16]. The investigation of few dopants in semiconductor should receive more focus. Undoubtedly, individual dopant atoms will act as the main functional part of transistors in the future.
2.
Control of dopant atoms
2.1
Single ion implantation
A promising candidate for deterministically placing a single atom is single-ion implantation, which was first proposed by Matsukawa et al. in 1977[17]. A single ion was extracted from the focused ion beam (FIB) by means of ultrafast switching of the ion beam. Later on, improvements with precision within 5 nm and deflection sensitivity better than 10?6 (dR/R) per nanometer were achieved owing to a series of efforts by Persaud et al., such as the integration of the ion beam with a scanning probe tip containing an aperture[18], the application of an integrated hollow pyramid instead of a hand mounted pyramid[19] and so on.
With the help of single-ion implantation, Takahiro Shinada et al.[4] achieved a deterministic dopants placement with high accuracy of 60 nm. The ordered dopant arrays effectively suppress not only the variation in the threshold voltage shift but also the off-state current caused by statistical fluctuations in the number and distribution of dopants.
2.2
Hydrogen-resist lithography
The hydrogen-resist lithography approach of near-atomic precision in dopant atom placement becomes a more competitive alternative in the longer term. Unlike ion implantation, hydrogen-resist lithography with the help of scanning tunneling microscopy (STM) yields single dopant atoms placement with atomic precision. The tip of an STM removes the hydrogen from certain regions of the surface of silicon wafer after covering the surface with a layer of hydrogen. Since the remaining hydrogen works as a mask, the phosphorus atom can be bound only in the predefined silicon surface when the wafer is exposed in phosphine (PH3) atmosphere. Finally, the phosphorus atom is adsorbed and incorporated by a critical anneal with help of the hydrogen-resist layer to block surface diffusion of phosphorus atom.
Based on this approach, Schofield et al. demonstrated the positioning of single phosphorus atoms in Si with an accuracy of 1 nm[14]. Later on, Fuechsle et al. fabricated an atomic scale device, i.e. single atom transistor, which includes a single dopant atom for the quantum-dot functionality, two in-plane gates to tune the electrostatic potential at the position of the dopant atom, and the source/drain electrodes for the I–V electric measurement[6]. The I–V curves at extremely low temperatures have shown the controllable quantum characteristics, from which one can learn more about the states of the dopant atom. Anyway, the single atom transistor has touched the ultimate limit of nanoscale devices.
2.3
Local space and concentration
As we all know, the gate length scaling is an effective way to continue Moore’s law. The decrease of gate-length dependent channel space results in the proportional decrease of the dopant-atom number. It has been concluded that there will be 24–56 (the average number is 40) dopant atoms within a (20 nm)3 subcube in the silicon material with an average concentration of 5 × 1018 cm?3[3]. Therefore, it is likely to roughly control the number of dopant atoms of a nanoscale device by the definition of the concentration and the gate length. Of course, advanced calculation is needed for this method to define the precise number of dopant atoms, which will be described in Section 4. In 2008, Lansbergen et al. utilized the low biasing condition to create a sufficiently small current-carrying region, so that dopant atoms can be able to be identified in the channel[9]. Inspired by this work, we combine the control of the gate length and concentration with the low biasing condition to create a bulk-channel region in junctionless nanowire transistor, in which the number of dopant atoms can be defined[20]. As a result, we get a small cross section of current-carrying region in bulk channel with adequate mobility, which is influenced much less by surface scattering than that in the inversion channel.
3.
Ionization energy of dopant
3.1
Estimation of ionization energy
Generally speaking, the ionization energy of III- or V-group dopant atoms in silicon can be roughly evaluated using the equation of hydrogen-like model as follows
$$left[{-frac{{{hbar ^2}}}{{2m^*}}{nabla ^2} + Vleft(r ight)} ight]fleft(r ight) = Efleft(r ight), $$ ![]() | (1) |
where m* is the effective mass of electron, V(r) is the additional potential, E is the energy of electron, f(r) is the wave function of electron and

High density of dopant induces the shift of Fermi level from intrinsic level to dopant level. In other words, the dopant states are gradually occupied, leading to incomplete ionization. A percentage of up to 25% of non-ionized dopant atoms exist at room temperature near the Mott transition[21]. It is important that higher dopant concentration in a semiconductor increases the free energy of the system[22], thus reducing the ionization energy. The value of the ionization energy has been parameterized by Altermatt et al. as follows[23],
$$E = frac{{{E_0}}}{{1 + {{left({{N_{ m{dop}}}/{N_{ m{ref}}}} ight)}^c}}}, $$ ![]() | (2) |
where E0 is the ground state energy, which can be calculated by using the nonlinear Thomas-Fermi-Dirac screening theory with adequate precision[24], Nref is the reference doping concentrations, c is a fit parameter. For phosphorus-doped crystalline silicon, E0 = 45.5 mV, Nref = 3 × 1018 cm?3, and c = 2.
Moreover, the ionization energy can be extracted from the mobility of the carriers based on the scattering mechanism. We found that the drain current has a minimum at 15 K for a junctionless nanowire transistor[25]. Impurity scattering is dominant for the mobility below 15 K and increase with the increase of the temperature. Above 15 K, the mobility grows with the increase of the temperature because of the increasing of the concentration of carriers by thermal activation. No doubt, Coulomb scattering is much stronger than impurity scattering ranging from 15 to 100 K. Moreover, phonon scattering gradually determines the mobility above 100 K. Part of the trend is shown in Fig. 1. Considering the impurity scattering mobility and the ionized impurity concentration, we got the following equation as the function of the ionization energy,

class="figure_img" id="Figure1"/>
Download
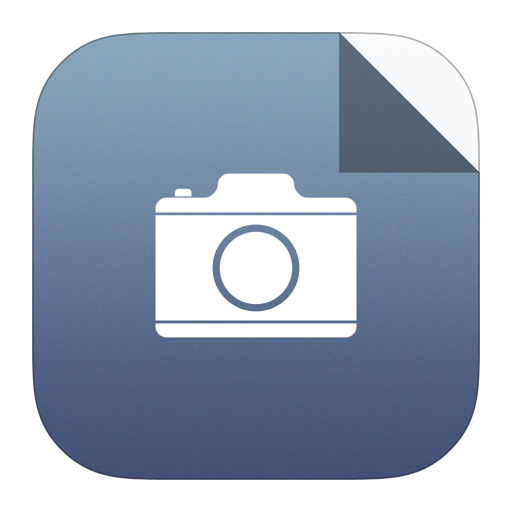
Larger image
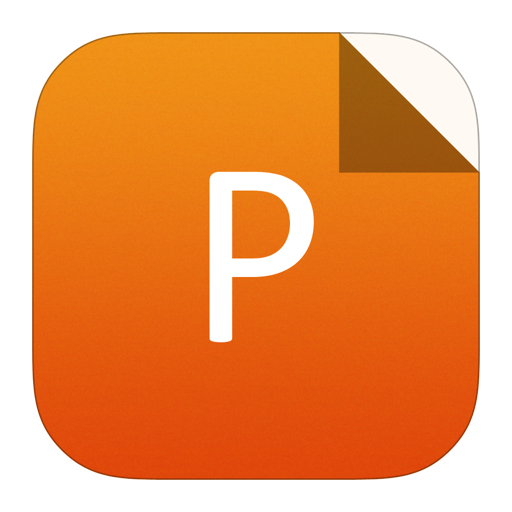
PowerPoint slide
Figure1.
Normalized electron mobility of theoretical calculation and experimental extraction[25].
$${mu _{ m{e}}} propto {T^{3/4}}exp ({E_{ m {D}}}/2{k_{ m{B}}}T)/m{^{*1/2}}, $$ ![]() | (3) |
where μe the electron mobility, m* the effective electron mass, kB the Boltzmann’s constant, ED the ionization energy. As a result, the ionization energy ED can be easily estimated according to
m{e}}}}}{{partial T}}left| {_{T = 15,{
m K}} = 0}
ight.$

3.2
Enhancement of ionization energy
The ionization energy is enhanced due to dielectric confinement[26, 27] which occurs where there is a significant dielectric mismatch between the wire and its surroundings. With the decrease of the diameter of the nanowire, ionization energies increase[25, 26]. The quantum confinement also partly contributes to the increase of ionization energies. For example, the quantum confinement instead of dielectric effect is dominant for radii below 2 nm in the freestanding phosphorus-doped Si nanocrystals[28, 29]. When the dielectric effect comes into account, a self-energy correction must be introduced and given by the formula[29]
$$E-{{E}_{0}}approx frac{2{{e}^{2}}}{{{varepsilon }_{text{in}}}R}frac{{{varepsilon }_{text{in}}}-{{varepsilon }_{text{out}}}}{{{varepsilon }_{text{in}}}+{{varepsilon }_{text{out}}}}Fleft( frac{{{varepsilon }_{text{in}}}}{{{varepsilon }_{text{out}}}} ight)text{ }, $$ ![]() | (4) |
where F is a function given in Ref. [27], R is the radius, e is the elementary charge, and the εin and εout are dielectric constant of a cylinder of radius R and of its surroundings, respectively. Sanquer et al. have done some investigations about single donor electronics[30, 31]. They extracted the ionization energy by calculating the resonance and further confirmed the enhancement of the ionization energy of a single As donor due to dielectric confinement. A simplified formula about dielectric confinement correction was given as
m{meV}}}}{{R left({
m{nm}}
ight)}}$

4.
Quantum dots induced by ionized dopant atoms
4.1
Dopant-induced quantum dots
A quantum dot (QD) is sometimes referred to as an artificial atom, which is a single object with bound and discrete electronic states[32, 33]. When the size of transistors comes into nanoscale, the ionized dopant atoms in the channel can work as quantum dots, which have been confirmed by identifying a p-type nanoscale dopant-induced dot in thin GaAs p–n multilayers earlier in 2003[34]. Later, Tabe et al. also found that the single electron could fill the phosphorus-donor potential well when they studied the effects of electron injection in the well by Kelvin probe force microscopy[35]. Thus, the ionized dopant atom can surely be regarded as a quantum dot in silicon FETs.
When the energy level of electrons in the potential of a single dopant-induced quantum dot is shifted to the Fermi level by gate voltage, the quantum transport occurs through the dopant-induced quantum dots. A conductance peak will form in the voltage-current curve where the transport occurs, which can be used to define the number of the quantum dots[36]. Single-electron tunneling in single-donor transistors has been observed at the temperatures ranging from 0.1 up to 100 K in the different type of devices[9, 15, 30, 37, 38]. Resonances have been observed at energies below the bottom of conduction band because of the isolated dopants in the channel. Conductance peaks were used to reveal the D0 and D1 charge states and their binding energy[15].
In 2008, a group in the Netherlands found that a single electron donor state in the nuclear potential of its donor atom may hybrid with a nearby quantum well when the gate was applied to a bias voltage[9]. Later on, they focused on the influence of applied fields and donor depth on the charging energy of D– states[39] and the implementation of wave function control over a single donor atom[40], which is a basic component of quantum device application.
4.2
Coupling of dopant-induced quantum dots
Different behaviors can be observed with the number increase of the discrete dopant atoms. Single-electron tunneling via multiple-donor clusters results in more current peak than that via individual donor atoms. Multiple-split peak feature was exhibited in a longer channel FET indicating the formation of a multiple-QD array[37]. The formation of multiple-split peak feature is the result of the interdot tunneling, which produces some new states of the entire dot array[36]. The multiple QDs induced by ionized dopant atoms have no interaction in low concentration below 1 × 1018 cm?3, because the average distance of two nearest phosphorous donors is remarkably larger than the 2rB, in which Bohr radius rB is around 2 nm in silicon[40, 41]. As the concentration increases, the interdot tunneling or interdot capacitance, i.e. the interaction during dots, is no longer negligible.
We have studied the current–voltage spectroscopies of dopant-induced quantum dots in single-channel and multiple-channel n-doped junctionless nanowire transistors (JNTs)[42, 43]. There is nearly the identical size of dopant-induced QDs and the similar inter-QD coupling effect in single-channel and multiple-channel JNTs. We observed the similar multiple-split current peak features for both single-channel and multiple-channel JNTs at the initial stage of conduction below the temperature of 75 K. However, the current oscillation at the initial stage gradually disappears above 75 K in our device because the thermal effects overcome the barrier of different energy subbands. To understanding the current oscillation features clearer, we calculated the transconductance gm and showed the curves of the gm as a function of gate voltage Vg at 6 K for varying Vds[43]. The position of the gm valleys is independent in the Vds within the voltage window. Moreover, the average spacing of gm valleys, combined with the equation
m g}} = e/Delta {V_{
m g}}$

An ionized phosphorous dopant atom can form three states, D–1 D0 and D1, when the dopant binds zero, one and two electrons respectively. With the ionized dopants get closer to each other, the discrete energy sates start to merge into lower and upper Hubbard bands because of the repulsion of the electron with the same spins, which is called Mott transition[21]. We observed two current oscillations containing seven subpeaks in heavily n-doped JNT at low temperature and small VDS, as shown in Fig. 2, which indicates the formed Hubbard band contains energy gap and discrete energy level[44]. Of course, the current oscillations are gradually weakened by the thermal energy with the temperature increasing to 70 K. It should be pointed out that the seven subpeaks can be clearly demonstrated in the gm–VGS curves instead of the IDS–VGS curves. The seven subpeaks correspond to the coupling of seven QDs, which agrees well with the above estimation of 11 QDs if the ionization rate[45] is taken into consideration. The subpeak spacing, which reflects the interaction of interdot coupling[46], increases with the increasing VGS. More positive VGS causes strong interdot coupling interaction with the transport channel widening so that some individual dopant atoms gradually develop into a dopant cluster. The quasi-one-dementional (1D) transport is eventually realized when the coupling interaction becomes strong enough in the channel. As a result, the electron behaviors are evolving by adjusting the gate voltage from QD regime characterized by Coulomb oscillations to 1D regime characterized by the current plateaus.

class="figure_img" id="Figure2"/>
Download
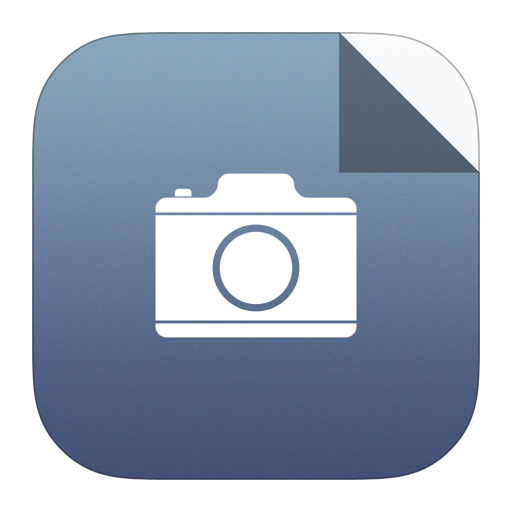
Larger image
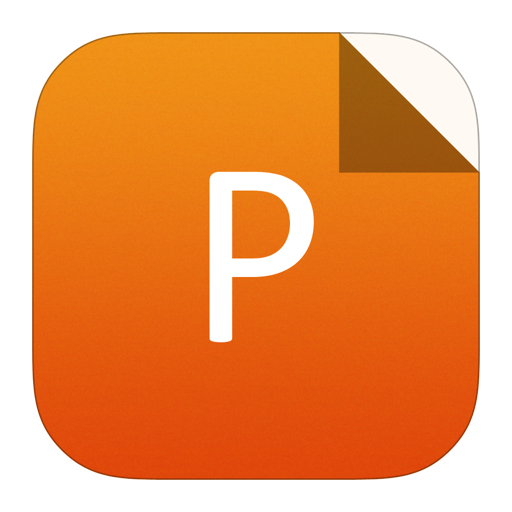
PowerPoint slide
Figure2.
IDS–VGS curves at T = 6 K with VDS ranging from 0.2 to 1.0 mV in step of 0.2 mV (up) and transconductance curves as a function of VGS (down)[44].
Recently, it has been reported by Moraru’s group that a-few-donor quantum dots provides a breakthrough for single-electron effects in selectively-doped devices at room temperature[47, 48]. A deep-level quantum dot caused by coupling of a few closely located donor atoms significantly suppresses thermal activation of carriers over the barrier. It provides a new approach to Coulomb blockade operation towards room temperature, apart from dielectric confinement in Si nanowires[49].
5.
Delocalization of electron from dopant atom
5.1
Hopping
The hopping is a quantum tunnel procedure in which electrons tunnel from one local state to another with or without the help of phonons[50]. The hopping of electrons can be denoted as nearest neighbor hopping or variable range hopping according to the activation energy of electrons for a successful hopping and the distance between the two local state centers. A hopping electron will always try to find the lowest activation energy and the shortest hopping distance, and the probability P was given as
$$P sim exp left({ - frac{{2r}}{a} - frac{{Delta E}}{{{k_{ m B}}T}}} ight), $$ ![]() | (5) |
where r is the optimum hopping distance, a is the localization length and E is the activation energy[51].
In an actual device, the random potentials caused by dopants will result in smearing of the band edge so that a mobility edge at some energy Ec separates localized states in the tail from extended states. With the increasing of density of dopants, the Fermi level will be gradually close to Ec and eventually go beyond Ec, which results in a conductance transition called Anderson transition[52]. As early as 1975, the Anderson transition was observed experimentally in silicon inversion layers[53]. Actually, the conductance is not zero when the Fermi level locates below Ec even at low temperature, but not 0 K, because the electrons can transport by variable-range hopping.
However, when the interaction of electrons is taken into consideration, another metal-insulator transition called Mott transition arises because of the formation of lower and upper Hubbard band, which results from the average repulsive energy between two electrons in the same atom. The density of the dopant is dominant for the Mott transition at low temperature, which is probed by means of quantum transport by Enrico Prati et al. taking advantage of the decrease in densities of implanted As atoms among the samples[54]. They also found that temperature can contribute to Mott transition to some degree because of variable-range hopping.
5.2
Transition from variable-range hopping to nearest-neighbor hopping
As we know, single electron tunneling through multiple quantum dots can feature a series of current peaks. It was found that the peak amplitude is stable below certain low temperatures in an extremely narrow channel MOSFET[55]. However, the conductance peak height at higher temperature varies exponentially with the activation energies. Thermally activated electrons can overcome not only the energy difference between two non-degenerate states with the assistance of phonons, but also the barrier induced by gate even for subthreshold voltage.
Mott believed that the most frequent electron hopping among localized states is the variable range hopping instead of the nearest neighbor hopping. Mott’s model further points out that the conductance depends on temperature as
$$G propto exp (-B/{T^v}),$$ ![]() | (6) |
with v = 1/4 for a three dimensional system while Efros’s model gives a v = 1/2[56]. Indeed, there is a critical temperature Tc, above which the conductivity follows Mott’s model while below which Efros’s model holds[56]. Meanwhile, at the critical temperature Tc, the hopping distance equals to the average distance of neighbor local states[51].
We have studied the conductance transition from variable range hopping to nearest neighbor hopping in the junctionless nanowire transistors[57, 58]. In order to understand the electron transport behaviors, the Arrhenius plot of the conductance as the function of the inverse temperatures was given to show a distinct conductance transition from single electron tunneling to thermally activated transport, separated by the transition temperature Tc of 30 K in low- and high-temperature regimes[57]. Below the critical temperature Tc, the conductance G depends weakly on the temperature because the variable range hopping holds at low activation energy. Above the critical temperature Tc, the activation energies were subsequently extracted by conductance linear fitting in the neighbor hopping region of the Arrhenius plot. According to the balance between the activation energy and the Coulomb interaction energy, the critical temperature Tc has been derived from
m c}} = frac{{2.4, {e^4}a{g_0}}}{{{k_{
m B}}{{(4pi {varepsilon _{
m r}}{varepsilon _0})}^2}}}$

ight|_{T = 30{,
m K}} = 0$

m B}}T}}}
ight)^{1/4}}$

As we increase the gate voltages and enlarge the temperature range up to 250 K, three temperature regions can be clearly observed, as shown in Fig. 3. At low temperature region (T < 30 K), the electrons can only hop among the equivalent energy levels (i.e. the variable range hopping) without phonon-assistant mechanism, resulting in the weak temperature dependence of the conductance. At intermediate temperature region (30 K < T < 100 K), the thermal electrons can be excited near the Femi energy and hop from the upper Hubbard band to conduction band (i.e. the nearest neighbor hopping) with the help of phonons. Therefore, the conductance is significantly affected by the temperatures. At high temperature (100 K < T <250 K), the thermal electrons can directly hop from the lower Hubbard band of donor states into the conduction band [58].

class="figure_img" id="Figure3"/>
Download
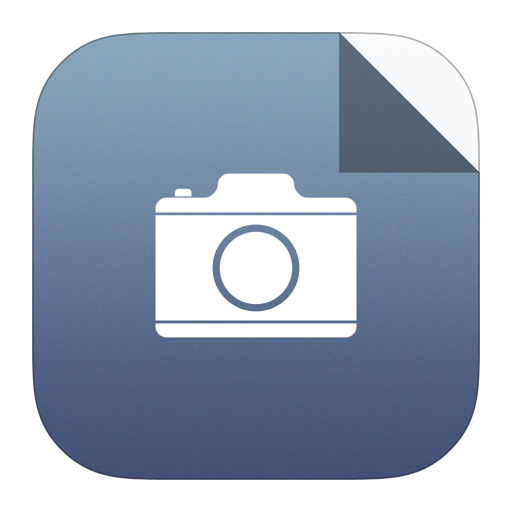
Larger image
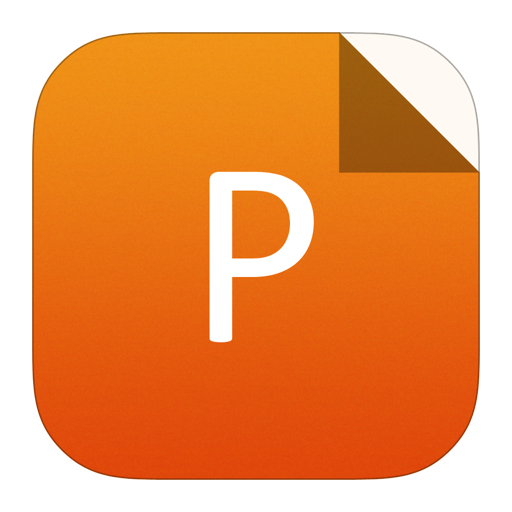
PowerPoint slide
Figure3.
(a) Arrhenius plot of the conductance at Vds = 5 mV for various gate voltages. (b) The activation energy as a function of gate voltages in different temperature regions[58].
The energy spacing between Ea2 and Ea3 (which are the activation energy in intermediate temperature region and high temperature region respectively) at the same gate voltage is considered as the energy needed to hop from the lower Hubbard band to the upper Hubbard band. The Hubbard band will broaden with the increase of gate voltage, which results in a smaller energy gap between the lower and upper Hubbard band. Therefore, the electrons at the band edge of localized states hop easily between two bands. Meanwhile, the activation energy Ea2 and Ea3 gradually fall and tend to be stable when we enlarged the gate voltages because the confinement potential of the conductance channel decrease and the conduction level moves close to the Fermi energy level of source.
6.
Characteristics features of 1-D transport
6.1
Junctionless nanowire transistors
The junctionless nanowire transistor (JNT)[59], which is indeed a gated resistor without junction, was firstly proposed and demonstrated by Colinge et al. in 2010. Their JNTs were fabricated with a cross section of 10 × 30.5 nm2 and a doping concentration ranging from 2 × 1019 to 5 × 1019 cm?3. A small cross section is required to allow for the full depletion of the channel, and a high doping concentration is needed to ensure a large current flow and a small contact resistance. The on/off current ratio of larger than 1 × 106 and a subthreshold slope (SS) of the order of 80 mV/dec were reached, indicating a good performance of the device. Compared with the traditional transistor, the JNT has a different conduction mechanism[60, 61] which contributes to the stability of the threshold voltage and the suppression of short channel effect[62]. The features of operation[63] and various methods for extraction parameters[64] of junctionless MOSFET have been reviewed for a better understanding.
6.2
One dimensional transport properties in JNTs
The nanowire MOSFETs are structures in which the channel is replaced with a nanowire. The silicon nanowire with diameters as small as 1 nm has been demonstrated[65]. Importantly, the nanowires exhibit one-dimensional (1-D) ballistic conduction at low diameters[66] and match well with the gate-all-around structure which is used to suppress the short channel effects. In 2008, Kim et al. theoretically investigated the 1-D ballistic transport characteristics by I–V characteristics of planar MOSFETs and of 1-D MOSFETs in two cases, one subband and two subbands at 0 K[67]. Their results show plateau-shaped features in ID–VG curve and gm peaks in gm–VG curves at low bias VDS.
The conduction path of JNT is clearly located at the center of the nanowire rather than the surface like the inversion channel[59], which allows the electrons to transport with bulk mobility without being scattered by the surface roughness (i.e. the bulk transport). Meanwhile, the electric field in the center of JNT is very small[68], which contributes to the marked conductance oscillations[69], i.e. 1-D ballistic conduction. Walter Hu et al. fabricated p-type back-gated nanowire FETs with cross sections of channel of 3–5 nm[70] and showed the 1D transport prevailing at 300 K[49]. The quantum confinement effects observed at room temperature may be a key to the new applications of Si devices.
We experimentally investigated the 1-D transport in single n-channel JNT at different temperatures[71, 72]. We experimentally investigated the 1-D transport in single n-channel JNT at low temperatures. The current plateaus are clearly observed at the temperature of 6 K above the threshold voltage, resulting from the electron transport through 1-D subbands. A noteworthy result is that the height of the second and third current step is about twice that of the first and fourth ones. We conclude that the six equivalent valleys in the conduction band are anisotropic in the different directions and will split into twofold degenerate and fourfold degenerate valleys due to the effective mass.

class="figure_img" id="Figure4"/>
Download
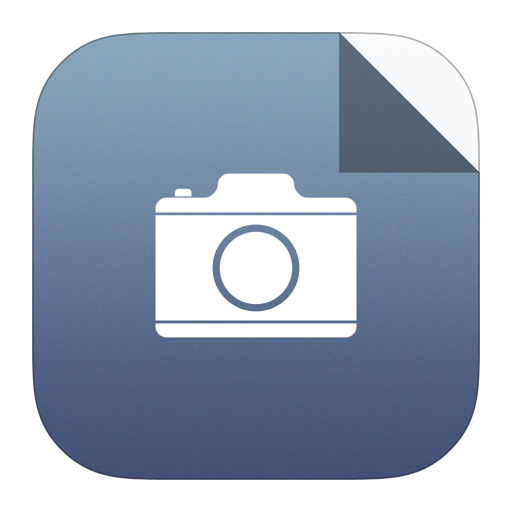
Larger image
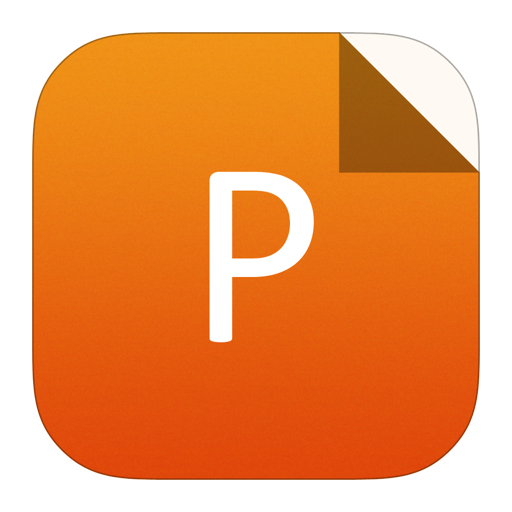
PowerPoint slide
Figure4.
Conductance and the number of subbands as a function of gate voltage for VDS = 10 mV at T = 6 K[72]. Inset: degenerate valleys in silicon nanowire.
7.
Conclusions
This review focuses on the study of few dopants as quantum components in silicon nanoscale devices. The JNT as an adequate platform of investigating dopant atoms has exhibited some interesting quantum features. Continuing miniaturization of semiconductor devices faces the basic physical limitations, such as quantum confinement, quantum tunneling and the atomistic nature of matter. The single atom transistor based on the dopant-induced QD stands for ultimate size of the solid device. Some quantum computing needs dopant atoms to realize the control of electron spins in silicon. The discrete or coupling dopant atoms determine the electron transport mechanism in nanoscale, thus the key technique for improving device performance is to realize the exercisable and fine control of dopant atom states. Meanwhile, a proper quantum confinement can significantly elevate the operating temperature, which has potential for the implementation of the single atom device at room temperature. Applications based on the dopant-induced QDs are very promising candidates for development of the semiconductor device.