

Corresponding authors: ? E-mail:hebing@gxnu.edu.cn? E-mail:oceanwen@gxnu.edu.cn
Received:2019-06-6Online:2019-12-1
Fund supported: |

Abstract
Keywords:
PDF (11218KB)MetadataMetricsRelated articlesExportEndNote|Ris|BibtexFavorite
Cite this article
Si-Hao Zhou, Wen Qiu, Yong Ye, Bing He, Bing-Hai Wen. The Effects of Wettability on Primary Vortex and Secondary Flow in Three-Dimensional Rotating Fluid*. [J], 2019, 71(12): 1480-1484 doi:10.1088/0253-6102/71/12/1480
1 Introduction
Stirring a cup of tea, the liquid is made to rotate and the leaves will soon collect in the center of the bottom, instead of the brim. The common and interesting phenomenon is called as "tea leaf paradox" and was used by Albert Einstein to explain the formation of meanders in the courses of rivers, in which the secondary flows play a key role.[1] The stirring makes a fluid system mixing or separation evolving from one state to another. The resulting secondary flow, which follows the primary flow or vortex, is fundamental for understanding atmospheric pressure systems and river course erosions, separating red blood cells from plasma or coagulated trub from beer in the brewing technique.[2] Jakubowski et al. studied the secondary flow in a whirlpool cycling vat, which is employed to remove hot trub in the process of wort boiling.[3] Taylor et al. used the dynamic filter made by the secondary flow principle in the coaxial double cylinder to implement the liquid extraction function.[4] Najjari et al. investigated a steady flow through a curved pipe using perturbation theory and found that there are two counter-rotating vortices in the cross section of the pipe.[5] Kefayati et al. studied the effects of secondary flow and eddy current on cerebral venous blood bank by utilizing imaging techniques.[6] Westra et al. analyzed the secondary flow in a centrifugal pump impeller by performing the CFD simulations.[7] On the other hand, the secondary flow has become an important technology in inertial microfluidics.[8] Di Carlo et al. realized cross-stream inertial migration in a micrometer winding tube, in which the secondary flow is used to accelerate the particle focusing.[9] Xiang et al. applied spiral microfluidic channels, in which the steady secondary flow promotes the particle focusing all the time.[10] Liu et al. analyzed the role of the secondary flow in tubes with different shapes.[11] Chung et al. created a sheathless and high-throughput microparticle focusing platform through geometry-induced secondary flows.[12] Sun et al. studied the inertial secondary flow in curved channel by the lattice Boltzmann method (LBM).[13] As the central equation in kinetic theory, the Boltzmann equation offers the possibility to simulate complex flows with a wide range of spatiotemporal scales in an effective and accurate way.[14] Over the past three decades, the LBM has been developed and modified with great enhancements in terms of precision and/or efficiency.[15]2 Model and Method
In this work, the flow in a rotating fluid with free interface is investigated. We focus on the effects of the surface wettability on the primary flow and the secondary vortex and hope to extend its applications in micro scale. The fluid system is modeled by the multiphase LBM driven by a chemical potential. The single-relaxation-time lattice Boltzmann equation can be concisely expressed as[16]where $\tau$ is the relaxation time, $F$ is the external force term, and $f_i^{(\rm eq)}$ is the equilibrium distribution function, $f_i(\textbf{x},t)$ is the particle distribution function at lattice site $\textbf{x}$ and time $t$, $\textbf{e}_i$ is the discrete speeds with $i = 0, ... , N.$
where $\omega_i$ is the weighting coefficient and $\textbf{u}$ is the fluid velocity. The nonideal force in a van der Waals fluid can be evaluated from the free energy, which determines the general expression of equation of state (EOS)[17]
The full pressure tensor can be written as
where $\delta_{\alpha\beta}$ is the Kronecker delta. The chemical potential can also be defined from the free energy as[18-20]
where
Then, the chemical potential can be calculated by the free-energy density
Thus, the nonideal force can be easily evaluated by the chemical potential avoiding the pressure tensor [21]
where $\stackrel{\leftrightarrow }{\textbf{p}}_0 = c_s^2\rho\stackrel{\leftrightarrow}{\textbf{\emph{I}}}$ is the ideal-gas equation of state. The nonideal force acts on the collision operator by simply increasing the particle momentum in $f_i^{(\rm eq)}$ in terms of the momentum theorem. The velocity in Eq. (2) is replaced by an equilibrium velocity[22]
Correspondingly, the macroscopic fluid velocity is redefined by the averaged momentum before and after the collision
It is verified that the multiphase model satisfies thermodynamics and Galilean invariance.[21] The water/vapor system is constrained by the famous Peng-Robinson (PR) EOS,
where the temperature function is $\alpha(T)=[1+(0.37464+1.54226\omega-0.26992\omega^2)\cdot(1-\sqrt{T/T_c})]^2$ with the acentric factor $\omega=0.344$ for water. The parameters take $a = {2}/{49}, b = {2}/{21}$ and $R = 1$. Correspondingly, the chemical potential is written as[21]
Figure 1 illustrates a schematic diagram of three-dimensional rotating fluid in a cylinder. We selected the three-dimensional LBM with 19 discrete velocities. A relaxation time is 1.3 and the temperature is $T_r = 0.8$. Both the length and width of the computational domain are 60 lattice units and the height is 120 lattice units. The cylinder radius is set as $R=30$ lattice units, $R^*$ denotes radial position. The fluid is driven to rotate by a stirring force, which is exerted at $r$=20 lattice units away from the central axis of the cylinder. The chemical-potential boundary condition is used on the surface of the cylinder, and the chemical potential of the cylinder surface is specified to determine the wettability of the cylinder.[20]
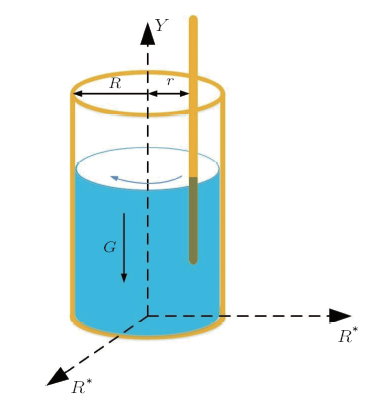
Fig. 1(Color online) Three-dimensional rotating fluid in a cylinder.
In the simulation experiment, we set the upper part of the cylinder to the gas phase and the lower part to the liquid phase with higher density. By changing the chemical potential of the wall and the bottom in our model, we can obtain the different contact angle and observe the effects of the wettability on the primary flow and the secondary vortex. The stirring force in the cylinder is set as $F=0.0018$ and the contact angle of the wall and the bottom is set as $30^{\circ}$, $60^{\circ}$, $90^{\circ}$, and $150^{\circ}$, respectively. From Fig. 2, we can observe the formation of the secondary flow in the vertical cross section. When the contact angle is $30^{\circ}$ that the wall is hydrophilic, the liquid is easily attracted by the surface and spreads on the wall, the shape of the primary vortex appears as a steep valley as shown in Fig. 2(a). As the contact angle increases, the wettability of the wall surface becomes hydrophobic, the liquid leaves the wall of the cylinder and the shape of primary vortex gradually becomes a saddle with two raised parts. Meantime, as the increase of contact angle, the concave liquid level rises gradually. Figure 3 draws a series of shapes of the primary vortexes when the surface wettability of the channel side is changing from hydrophilic into superhydrophobic. Interestingly, a small secondary vortex is discovered near the three-phase contact when the surface has a moderate wettability; it gradually weakens and disappears when the surface becomes strong hydrophilic or hydrophobic. This indicates that the formation of the small vortex is affected by the contact angle of wall and is result of the interaction between the secondary flow and curved gas/liquid interface.
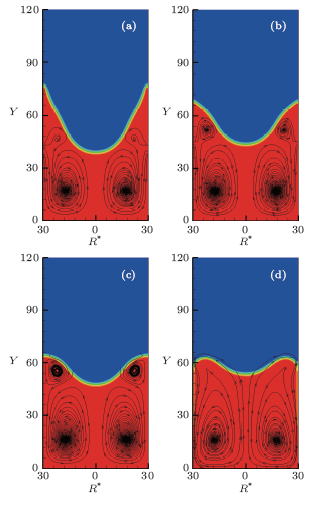
Fig. 2(Color online) The primary vortex and the secondary flow at different contact angles, (a) $\theta = 30^{\circ}$, (b) $\theta = 60^{\circ}$, (c) $\theta = 90^{\circ}$, (d) $\theta = 150^{\circ}$.
The big secondary vortex can always exist; its position and vorticity are influenced by the surface wettability of the cylinder. In order to investigate the influence of surface wettability on the vortex position and vorticity, the flows in the cylinders with three different volumes are simulated and analyzed. Namely, the heights of the liquid level in three different volumes are 50, 60, and 80 lattice unites, respectively. The effects of different surface wettability of side wall and bottom are investigated separately. Firstly, the surface wettability of the cylinder bottom remains neutral, namely the contact angel $90^{\circ}$, whereas the contact angle of the side wall changes from $30^{\circ}$ to $160^{\circ}$ with the increment $10^{\circ}$ every time.
Figure 4 shows the influence of the surface wettability of side wall on the vortex position and intensity. The trends of the three different liquid heights are consistent, especially the vorticities of them are almost the same. The more hydrophobic the cylinder side is, the stronger the vortex intensity is, and the nearer to the centerline and bottom the vortex core is. When the contact angle increases, the wetting property of the side surface changes from hydrophilic into hydrophobic. These bring down the friction of the side surface,[23] and then enhance the secondary flow. Similarly, the side friction decreases, the rotation speed increases, the secondary flow vortex moves down and the bottom friction increases.
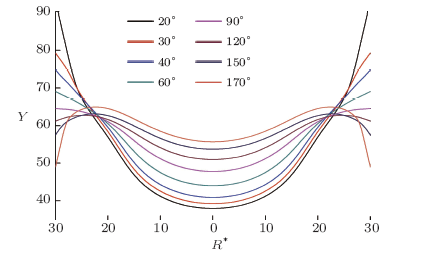
Fig. 3(Color online) The shapes of the primary vortexes are changing with the different wettability of the side surface.
Secondly, the surface wettability of the cylinder side remains $90^{\circ}$, while the contact angle of the bottom changes from $30^{\circ}$ to $160^{\circ}$ with the increment $10^{\circ}$ every time. As shown in Fig. 5, although the more hydrophobic bottom makes the secondary vortex stronger and pulls the vortex core nearer to the centerline and bottom, the heights of the vortex core for different liquid volumes are clearly different. Furthermore, both the decrease of the vortex core and the increase of the vortex intensity caused by the growth of the bottom contact angle are slow than those by the side surface. On the whole, the wetting effect of the cylinder side is more intensive than the bottom.
At last, the influence of the stirring force on the secondary flow is studied. The contact angles of the side and bottom surface remain $90^{\circ}$, while the stirring force changes from 0.0014 to 0.0024. As shown in Fig. 6, the influences of stirring force on the secondary flow are highly consistent for three different liquid level heights. Although the horizontal position of the secondary vortex only moves a little, its height is raised evidently and the vortex intensity has a substantial increased too. With the increase of the stirring force, the angular velocity is larger and larger, and then the bigger centrifugal force pushes the liquid to the cylinder side. The center of the primary vortex drops and the edge rises notably. Consequently, the vortex core of the secondary flow is pushed up and enhanced greatly.
3 Conclusion
In this work, the multiphase LBM driven by a chemical potential is used to study the flow of rotating fluid in a three-dimensional cylinder. Through a series of numerical simulations, the effects of stirring force and surface wettability on primary vortex and secondary flow are presented clearly. It can be concluded that when the stirring force is constant, the contact angle has a positive correlation with the position of secondary flow and the vortex intensity, and the surface wettability affects the shape of the primary vortex. The increase of the stirring force also enhances the secondary flow and pushes the vortex up. Remarkably, we find there is a small secondary vortex near the three-phase contact line when the surface has a moderate wettability, owing to the interaction between the secondary flow and the curved gas/liquid interface. The research on secondary flow not only helps us to explain the natural phenomena, but also has important significance for the related performance improvement in industrial production and medical research, especially in inertial microfluidics.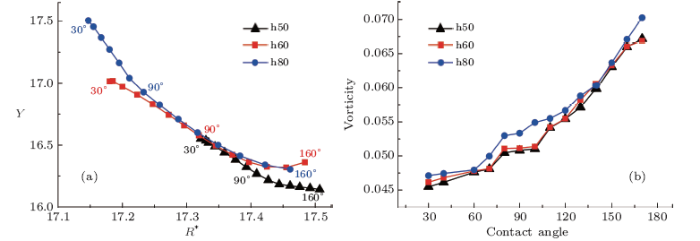
Fig. 4(Color online) The impacts of the wettability of side wall on (a) vortex core and (b) the intensity of the vortex. The curve in black, red, and blue represent liquid level at 50, 60, 80 lattice unites, respectively.
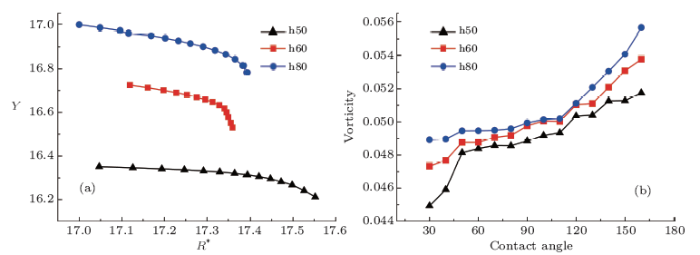
Fig. 5(Color online) (a) The impacts of the bottom wettability on the vortex position of the cylinder and (b) on the strength of the vortex.
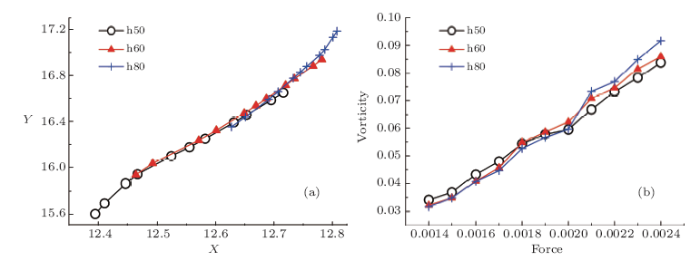
Fig. 6(Color online) (a) The influence of stirring force on the position of the vortex core and (b) on the strength of the vortex.
Reference By original order
By published year
By cited within times
By Impact factor
[Cited within: 1]
[Cited within: 1]
[Cited within: 1]
[Cited within: 1]
[Cited within: 1]
DOI:10.1016/j.jbiomech.2016.11.041URLPMID:27894675 [Cited within: 1]

Aberrations in flow in the cerebral venous outflow tract (CVOT) have been implicated as the cause of several pathologic conditions including idiopathic intracranial hypertension (IIH), multiple sclerosis (MS), and pulsatile tinnitus (PT). The advent of 4D flow magnetic resonance imaging (4D-flow MRI) has recently allowed researchers to evaluate blood flow patterns in the arterial structures with great success. We utilized similar imaging techniques and found several distinct flow characteristics in the CVOT of subjects with and without lumenal irregularities. We present the flow patterns of 8 out of 38 subjects who have varying heights of the internal jugular bulb and varying lumenal irregularities including stenosis and diverticulum. In the internal jugular vein (IJV) with an elevated jugular bulb (JB), 4Dflow MRI revealed a characteristic spiral flow that was dependent on the level of JB elevation. Vortical flow was also observed in the diverticula of the venous sinuses and IJV. The diversity of flow complexity in the CVOT illustrates the potential importance of hemodynamic investigations in elucidating venous pathologies.
[Cited within: 1]
DOI:10.1103/PhysRevLett.102.094503URLPMID:19392526 [Cited within: 1]

Nonlinearity in finite-Reynolds-number flow results in particle migration transverse to fluid streamlines, producing the well-known "tubular pinch effect" in cylindrical pipes. Here we investigate these nonlinear effects in highly confined systems where the particle size approaches the channel dimensions. Experimental and numerical results reveal distinctive dynamics, including complex scaling of lift forces with channel and particle geometry. The unique behavior described in this Letter has broad implications for confined particulate flows.
DOI:10.1073/pnas.0704958104URLPMID:18025477 [Cited within: 1]

Under laminar flow conditions, when no external forces are applied, particles are generally thought to follow fluid streamlines. Contrary to this perspective, we observe that flowing particles migrate across streamlines in a continuous, predictable, and accurate manner in microchannels experiencing laminar flows. The migration is attributed to lift forces on particles that are observed when inertial aspects of the flow become significant. We identified symmetric and asymmetric channel geometries that provide additional inertial forces that bias particular equilibrium positions to create continuous streams of ordered particles precisely positioned in three spatial dimensions. We were able to order particles laterally, within the transverse plane of the channel, with >80-nm accuracy, and longitudinally, in regular chains along the direction of flow. A fourth dimension of rotational alignment was observed for discoidal red blood cells. Unexpectedly, ordering appears to be independent of particle buoyant direction, suggesting only minor centrifugal contributions. Theoretical analysis indicates the physical principles are operational over a range of channel and particle length scales. The ability to differentially order particles of different sizes, continuously, at high rates, and without external forces in microchannels is expected to have a broad range of applications in continuous bioparticle separation, high-throughput cytometry, and large-scale filtration systems.
DOI:10.1021/acsami.9b17936URLPMID:31794661 [Cited within: 1]

Stimuli-responsive anisotropic slippery surfaces (ASSs) have demonstrated intriguing performance in manipulating the behaviors of some liquids. However, most present methods have been limited to conductive droplets, certain specific conductive platforms and higher manipulation temperature that greatly hinder its practical applications. Here, an electric-responsive paraffin-infused ASS (ER-PIASS) composed of paraffin, micro-grooved PDMS, and flexible embedded silver nanowire heater is reported. Owing to the fast electric-response of ER-PIASS, smart control between anisotropic sliding and pinning for diverse liquids can be realized by remotely loading and discharging electric-stimuli. The underlying mechanism is that the generated Joule heat melts the solidified paraffin to slide a pinning droplet once an electric-trigger is loaded due to the formation of a slippery air/liquid/liquid/solid system. Once the voltage is discharged, the liquefied paraffin would rapidly solidify to stick a slipping droplet because of the recovery of a frictional air/liquid/solid system. Additionally, the effect of groove's height (h), spacing between two adjacent grooves (d) and thickness of paraffin layer on the anisotropic degree was quantitatively studied and an optimized value of 75o is thus harvested. Through tuning the recipe of the hybrid lubricant, the responsive voltage and temperature for ER-PIASS can be dramatically decreased to ultra-low figures of 2.0 V and 34.2 oC. By taking advantage of this ultralow-voltage-driven biocompatible ER-PIASS, we enable the anisotropic smart control of cell culture medium and yeast droplets for their directional coalesce, growth and fission. We believe that such stimuli-responsive surfaces will be promising candidates for manipulating droplets directional sliding behavior and further bloom the studies of flexible microfluidics devices.
DOI:10.1039/c5lc01522gURLPMID:26794086 [Cited within: 1]

Inertial microfluidics has been widely used in high-throughput manipulation of particles and cells by hydrodynamic forces, without the aid of externally applied fields. The performance of inertial microfluidic devices largely relies on precise prediction of particle trajectories that are determined by inertial lift acting on particles. The only way to accurately obtain lift forces is by direct numerical simulation (DNS); however, it is burdensome when applied to practical microchannels with complex geometries. Here, we propose a fitting formula for inertial lift on a sphere drawn from DNS data obtained in straight channels. The formula consists of four terms that represent the shear-gradient-induced lift, the wall-induced lift, the slip-shear lift, and the correction of the shear-gradient-induced lift, respectively. Notably, as a function of the parameters of a local flow field, it possesses good adaptability to complex channel geometries. This generalized formula is further implemented in the Lagrangian particle tracking method to realize fast prediction of particle trajectories in two types of widely used microchannels: a long serpentine and a double spiral microchannel, demonstrating its ability to efficiently design and optimize inertial microfluidic devices.
DOI:10.1002/smll.v9.5URL [Cited within: 1]
[Cited within: 1]
DOI:10.1103/PhysRevE.99.012142URLPMID:30780360 [Cited within: 1]

A multiple-relaxation-time discrete Boltzmann model (DBM) is developed for compressible thermal reactive flows. A unified Boltzmann equation set is solved for hydrodynamic and thermodynamic quantities as well as higher order kinetic moments. The collision, reaction, and force terms are uniformly calculated with a matrix inversion method, which is physically accurate, numerically efficient, and convenient for coding. Via the Chapman-Enskog analysis, the DBM is demonstrated to recover reactive Navier-Stokes (NS) equations in the hydrodynamic limit. Both specific heat ratio and Prandtl number are adjustable. Moreover, it provides quantification of hydrodynamic and thermodynamic nonequilibrium effects beyond the NS equations. The capability of the DBM is demonstrated through simulations of chemical reactions in the free falling process, sound wave, thermal Couette flow, and steady and unsteady detonation cases. Moreover, nonequilibrium effects on the predicted physical quantities in unsteady combustion are quantified via the DBM. It is demonstrated that nonequilibrium effects suppress detonation instability and dissipate small oscillations of fluid flows.
DOI:10.1103/PhysRevE.96.053305URLPMID:29347713 [Cited within: 1]

A discrete Boltzmann model (DBM) is proposed to probe the Rayleigh-Taylor instability (RTI) in two-component compressible flows. Each species has a flexible specific-heat ratio and is described by one discrete Boltzmann equation (DBE). Independent discrete velocities are adopted for the two DBEs. The collision and force terms in the DBE account for the molecular collision and external force, respectively. Two types of force terms are exploited. In addition to recovering the modified Navier-Stokes equations in the hydrodynamic limit, the DBM has the capability of capturing detailed nonequilibrium effects. Furthermore, we use the DBM to investigate the dynamic process of the RTI. The invariants of tensors for nonequilibrium effects are presented and studied. For low Reynolds numbers, both global nonequilibrium manifestations and the growth rate of the entropy of mixing show three stages (i.e., the reducing, increasing, and then decreasing trends) in the evolution of the RTI. On the other hand, the early reducing tendency is suppressed and even eliminated for high Reynolds numbers. Relevant physical mechanisms are analyzed and discussed.
DOI:10.1146/annurev.fluid.30.1.329URL [Cited within: 1]
DOI:10.1017/S0003356100010229URL [Cited within: 1]
[Cited within: 1]
[Cited within: 2]
DOI:10.1103/PhysRevE.95.063305URLPMID:28709336 [Cited within: 3]

Chemical potential, as an important thermodynamic quantity, has been popularly used in thermodynamic modeling for complex systems, especially for those involving the phase transitions and chemical reactions. Here we present a chemical-potential-based multiphase lattice Boltzmann model, in which the nonideal force is directly evaluated by a chemical potential. The numerical computation is more efficient than the pressure-tensor-based model [Wen et al. Europhys. Lett. 112, 44002 (2015)10.1209/0295-5075/112/44002] because the calculations of the pressure tensor and its divergence are avoided. We have derived several chemical potentials of the popular equations of state from the free-energy density function. The theoretical analyses and numerical results support that the present model satisfies thermodynamics and Galilean invariance. An effective chemical-potential boundary condition is also implemented to investigate the wettability of a solid surface, and the contact angle can be linearly tuned by the surface chemical potential.
DOI:10.1103/PhysRevE.74.046703URLPMID:17155208 [Cited within: 1]

We present a further theoretical extension to the kinetic-theory-based formulation of the lattice Boltzmann method of Shan [J. Fluid Mech. 550, 413 (2006)]. In addition to the higher-order projection of the equilibrium distribution function and a sufficiently accurate Gauss-Hermite quadrature in the original formulation, a regularization procedure is introduced in this paper. This procedure ensures a consistent order of accuracy control over the nonequilibrium contributions in the Galerkin sense. Using this formulation, we construct a specific lattice Boltzmann model that accurately incorporates up to third-order hydrodynamic moments. Numerical evidence demonstrates that the extended model overcomes some major defects existing in conventionally known lattice Boltzmann models, so that fluid flows at finite Knudsen number Kn can be more quantitatively simulated. Results from force-driven Poiseuille flow simulations predict the Knudsen's minimum and the asymptotic behavior of flow flux at large Kn.
[Cited within: 1]