1.
Introduction
Semiconductor nanomaterials referred to as quantum dots (QDs) have been extensively popular in the recent past due to their distinctive size-dependent optical properties, tailored surface modification and good biocompatibility leading to use as sensor element for many industrial and agricultural applications. As the particle size is reduced to smaller than their exciton Bohr radius, the excitons formed are confined to a potential well. Relatively, changes exist in the fundamental properties of semiconducting materials exist like an increased band gap, reduced melting point, electronic orbitals density and geometries of bonding by simply decreasing particle size. These size-controlling properties affect a semiconductor material instantly restricting to a nanometer scale in all three dimensions, leading to the semiconducting spherical nanocrystals, also referred as quantum dots (QDs)[1]. In addition, quantum dots referred as those whose particle size is less than 100 nm. Nowadays, QDs are fabricated by incorporating elements of the II–VI group that have been acquiring scientific attraction due to their distinct properties.
Among all the semiconducting materials, ZnS is the cutting-edge material that can be preferred as the perfect alternative in gas sensors. It has a large surface to volume (s/v) ratio, high Bohr exciton radius and huge exciton binding energy, wide bandgap and quantum confinement effect. The exceptionality of these materials is tailoring the electronic properties by doping with suitable metals and through the impact of the carrier’s confinement. ZnS, an II–VI semiconductor, non-toxic, has a wide bandgap of 3.68 eV (for bulk) that realizes a good host for the total number of transition and rare earth metal ion dopants contribute to significant optical and magnetic properties. These materials are very useful in designing short-wavelength LEDs, electroluminescence devices, sensors, laser diodes, ?at panel display, biological imaging, solar cells, optoelectronic devices and labelling[2-7]. There are numerous approaches to fabricate nanoparticles (quantum dots), such as the aqueous method[8], chemical bath deposition[9, 10], refluxing technique[11], pulsed laser ablation[12], solid-state reaction method[13], solvothermal technique[14], hydrothermal method[15, 16], co-precipitation and colloidal thermolysis and aerosol assisted chemical vapor deposition[17-22]. Among all these approaches, co-precipitation is the uncomplicated and cost-efficient method. In this technique, various parameters such as pH, temperature and time of reaction, initial solution concentration and material are chosen, which play a crucial role in preparing ceramic powders with the required shape and size.
Nowadays, in the atmosphere, ammonia is being released to a large extent multiplied by human activity. When a large extent of nitrification process is done to the agricultural land in the form of fertilizer (NH3), then due to run-off from the land it leads to acidification of water bodies that endangers aquatic organisms. Similarly, excessive nutrients in lakes or water bodies lead to eutrophication. The total worldwide contribution of nitrogen fixation related to ammonia emission approaches 1.0 Tg/year[23]. Furthermore, many chemical industries produce ammonia for the manufacture of fertilizers and utilize it in refrigeration systems. But this exposure to higher concentrations of ammonia leads to severe health hazards. Near intensive farming also high concentration levels than the allowed exposure limit result in unhealthful conditions inside the stables for farmers and animals, wherever the concentration levels are the maximum[24]. Its odour can detect the high concentration of ammonia, but it is necessary to detect the low gas concentration, yet the human nose fails to detect it. Hence it is essential to design a sensor to detect low concentrations of ammonia vapors.
In the present investigations, ZnS-based quantum dots were synthesized using the co-precipitate technique to detect the low concentration of ammonia. To improve gas sensing properties, divalent cobalt ions with different at.% were doped into the ZnS system. The ionic radius of the cobalt with 0.058 nm is in close proximity to that of the 0.06 nm ionic radius of Zn2+ divalent cation. Hence, divalent Co2+ transition metal ion can be successfully incorporated in the ZnS quantum dots up to a particular absorbance of 0.05–0.25 at.%. Thus, we have synthesized ZnS based quantum dots with cobalt as dopant with 0.05 at.% and 0.25 at.%. Synthesized pure ZnS quantum dots, cobalt doped ZnS quantum dots were systematically studied their structural, morphological and chemical properties. With the aid of these properties, it is demonstrated that the dopant cobalt is embedded in the ZnS quantum dots. Gas sensing properties of these quantum dots were performed towards 50 ppm of ammonia vapors at an operating temperature of 70 °C in static mode and the results were reported.
2.
Materials and methods
2.1
Materials
All the reagents used in the present study are analytical grade (99%) without any further purification procured from Sigma Aldrich, India.
2.2
Experimental studies
Pure and cobalt-doped ZnS (Zn1–xCoxS with x = 0.00, 0.05, and 0.25 at.%) powdered samples were synthesized by the co-precipitation method. Raw materials required for the synthesis of pure ZnS are zinc acetate dihydrate [Zn(CH3COO)2·2H2O] of 1 M dissolved in distilled water (50 mL) and another sodium sulfide [Na2S] of 1 M is dissolved in distilled water (50 mL) separately and then both the solutions were combined after continuous magnetic stirring for 2 h to obtain the pH of 13.5. Precipitate in white color was obtained, which was then separated by using centrifugation. The separation of precipitate is done after washing the prepared sample with distilled water and ethanol several times. It is then dried under vacuum conditions at 600 °C to get powder samples of ZnS quantum dots. Once the pure ZnS sample is obtained, then for the synthesis of cobalt incorporated ZnS quantum dots, zinc acetate and cobalt acetate were taken as starting precursors. These precursors were dissolved in deionized water separately and mixed. Later on, to obtain the pH 13.5, sodium sulfide is added dropwise and stirred for 2 h. The precipitate thus obtained is then drained and then for the removal of all sodium particles, the residue was washed with ethanol several times. At the end of this procedure, the wet precipitate has dried to obtain cobalt-doped quantum dots.
2.3
Characterization
The prepared samples were analyzed by X-ray powder diffraction (XRD) using XPERT-PRO (Model: PW-3710) operated at 45 kV with 40 mA with a Cu-Kα radiation source of wavelength 0.154 nm in the range of 10°–90° of Bragg’s angle to determine the structural parameters. A scanning electron microscope (SEM) (Model: JSM6100) with an image analyzer was used to analyze the morphological features of the pure and cobalt-doped ZnS quantum dots. Transmission electron microscopy (TEM) was performed using a Hitachi (Model: H-7500) with an operating voltage of 200 kV. A Fourier transform infrared spectroscopy study was carried out to determine the chemical composition of the samples using Thermo Nicolet Nexus 670.
2.4
Gas sensing characterization
The gas sensing characterizations have been performed using an indigenous air sealed gas testing chamber in the static method using a Keithley electrometer (6517B). The required Ohmic electrical contacts were established on the sensor element with the help of thin copper wires and highly conducting silver electrodes. This paper ought to report the gas sensing properties of ZnS and cobalt-doped ZnS quantum dots at an operating temperature of 70 °C towards 50 ppm of ammonia.
3.
Results and discussion
3.1
Structural studies
From X-ray diffraction, as shown in Fig. 1, it is noticed that there are three major diffraction peaks for ZnS and cobalt-doped ZnS quantum dots related to (111), (220) and (311) planes which are analyzed to coincide with the standard JCPDS card No. 05-0566 confirming the cubic ZnS structure. For cobalt-doped ZnS, the diminished intensities of diffraction peaks were observed, which imply that Co2+ ions are incorporated effectively in the inner lattice of Zn2+ ions of ZnS lattice. It is also noticed either no extra or any impurity phases evident in cobalt-doped ZnS samples, indicating that the prepared samples are single in phase, thereby clearly indicating that cobalt has been incorporated into the lattice a substitution atom. Applying the subsequent relation, the lattice constant '


class="figure_img" id="Figure1"/>
Download
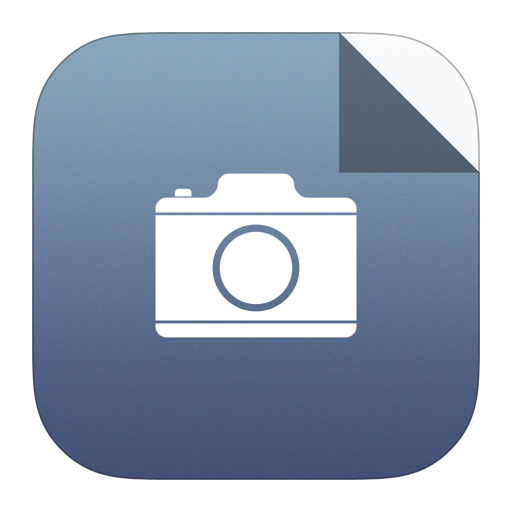
Larger image
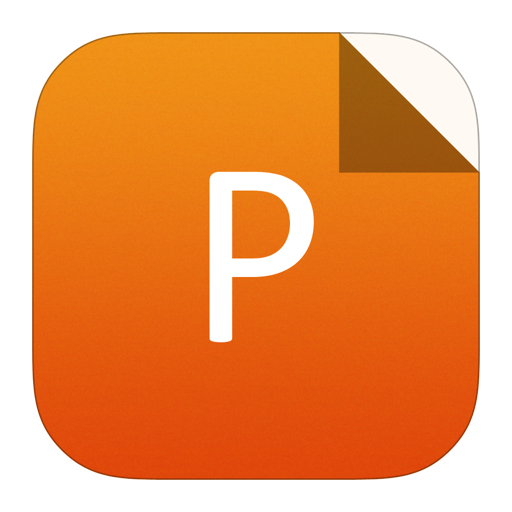
PowerPoint slide
Figure1.
(Color online) X-ray diffraction pattern of ZnS and cobalt-doped ZnS quantum dots.
$${d_{hkl}} = frac{a}{{sqrt {{h^2} + {k^2} + {l^2}} }},$$ ![]() |
where d is interatomic distance,

Sample | Lattice constant ‘$a $’ (nm) | Volume of unit cell (10–30) m3 | Crystallite size D (nm) | Strain (ε) (10–4) |
ZnS | 0.529 | 148.03 | 4.84 | 2.76 |
0.05 at.% cobalt doped ZnS quantum dots | 0.527 | 146.36 | 2.43 | 4.23 |
0.25 at.% cobalt doped ZnS quantum dots | 0.524 | 143.88 | 2.81 | 4.87 |
Table1.
Structural properties of pure and cobalt-doped ZnS quantum dots.
Table options
-->
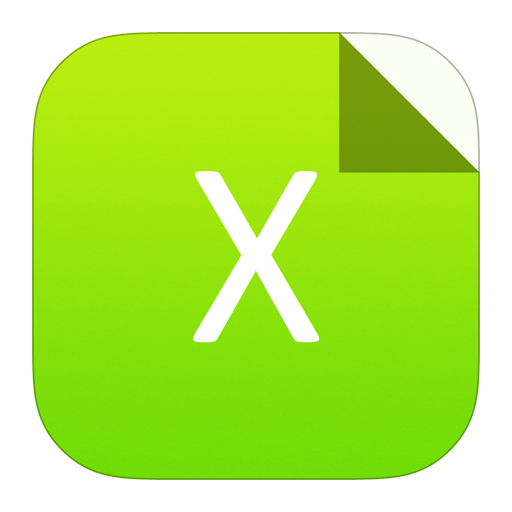
Download as CSV
Sample | Lattice constant ‘ ![]() ![]() | Volume of unit cell (10–30) m3 | Crystallite size D (nm) | Strain (ε) (10–4) |
ZnS | 0.529 | 148.03 | 4.84 | 2.76 |
0.05 at.% cobalt doped ZnS quantum dots | 0.527 | 146.36 | 2.43 | 4.23 |
0.25 at.% cobalt doped ZnS quantum dots | 0.524 | 143.88 | 2.81 | 4.87 |
$$D = frac{{0.9lambda }}{{beta costheta }},$$ ![]() |
where λ is the wavelength of X-rays, θ is diffraction angle and β is full-width half maxima (FWHM). With the increasing of cobalt doping concentration in the ZnS matrix, the diminishing values of D is observed, which is in the range of 2 to 5 nm, which are also called quantum dots since the synthesized nanoparticles are in the quantum regime as its size is less than exciton Bohr radius[32]. Compared with the pure ZnS quantum dots, the crystallite size of the 0.05 at% cobalt doped ZnS quantum dots is reduced due to distortion and micro-strain induced in the lattice due to the incorporation of cobalt ions in the ZnS lattice[33]. The slight increase in the crystallite size in the case of 0.25 at.% of cobalt-doped ZnS quantum dots is due to the enhancement of grain surface growth due to the excess concentration of cobalt. The calculated average crystallite size values are tabulated in Table 1.
The micro strain was determined using the following equation[34].
$$varepsilon = frac{beta }{{4 {{tan}} theta }}.$$ ![]() |
In strain values as depicted in Table 1, we have noticed a sudden rise in the strain from pure ZnS to cobalt-doped ZnS quantum dots due to a large amount of cobalt doping and this consequently has given rise to a significant distortion resulting in the broadening of the peak in the lattice and thus degrade the crystallinity of the sample, similar studies were reported by Singhal et al.[27]. In comparison with the pure ZnS, certain modifications observed in structural parameters, such as decrement in size, increment in strain and decrement in lattice parameters, illustrate the existence of Co2+ ions in the ZnS lattice.
3.2
Morphology studies
3.2.1
SEM analysis
Scanning electron microscopy (SEM) is an effective instrument to analyze surface morphology. Fig. 2 displays the typical SEM micrographs of pure and cobalt-doped ZnS quantum dots. These images have shown that the particles distributions are almost spherical and these quantum dots are agglomerated. The agglomeration and roughness of these particles slightly increase with an increasing dopant concentration. The rough surface is beneficial for gas detection since it can provide more active sites and a high specific surface area required for excellent gas sensing properties.

class="figure_img" id="Figure2"/>
Download
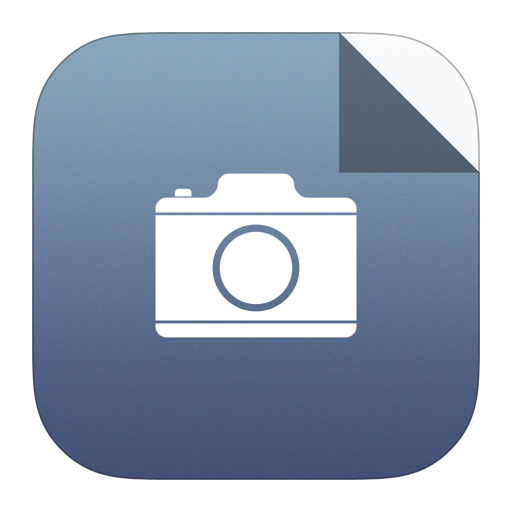
Larger image
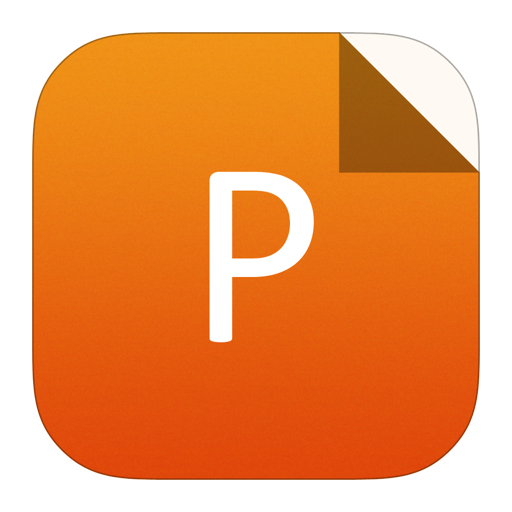
PowerPoint slide
Figure2.
SEM images of (a) ZnS, (b) 0.05 at.% cobalt doped ZnS quantum dots, (c) 0.25 at.% cobalt doped ZnS quantum dots.
3.2.2
TEM analysis
The microstructure of pure and cobalt-doped ZnS quantum dots was also examined in detail with the help of TEM. Fig. 3 displays TEM images of pure and cobalt-doped ZnS quantum dots. From these images, uniform size distribution is observed and the particle average size is in the range of 3–6 nm. The crystallite size calculated from XRD is in good correlation with the average crystallite size values obtained from TEM micrographs.

class="figure_img" id="Figure3"/>
Download
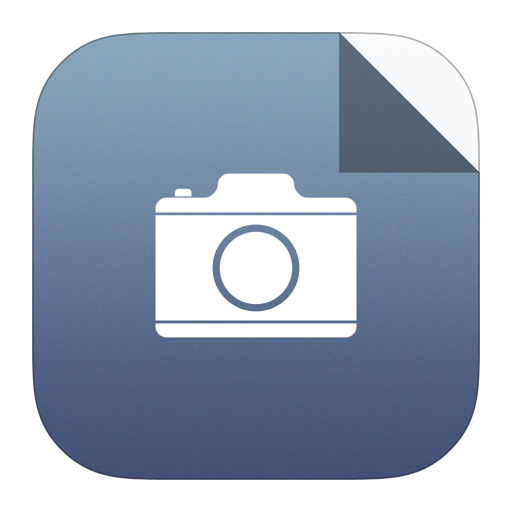
Larger image
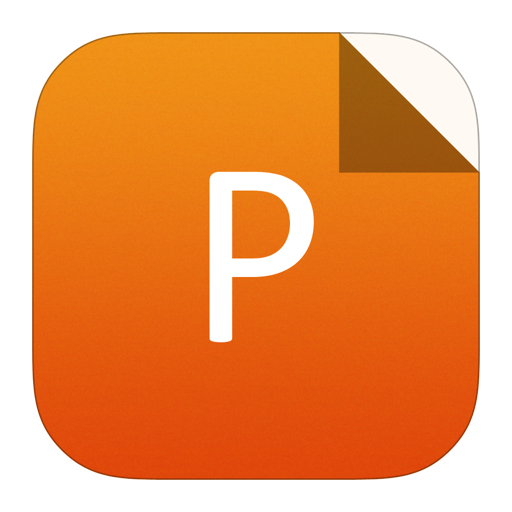
PowerPoint slide
Figure3.
TEM images of (a) ZnS, (b) 0.05 at.% cobalt-doped ZnS quantum dots (c) 0.25 at.% cobalt-doped ZnS quantum dots.
3.3
Chemical analysis using EDX
EDX is a perfect tool to analyze material chemical composition. Fig. 4 displays the EDX spectrum of pure and cobalt-doped ZnS quantum dots existence of Zn, Co and S are confirmed and no additional element traces are observed in the spectrum. This illustrates that high purity Co-doped ZnS quantum dots can be synthesized by the chemical precipitation process. A precise observation of a low-intensity peak for cobalt-doped ZnS shows the proper incorporation of cobalt in the structure.

class="figure_img" id="Figure4"/>
Download
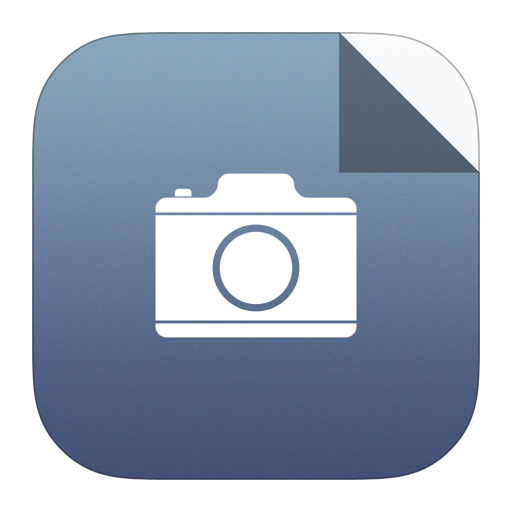
Larger image
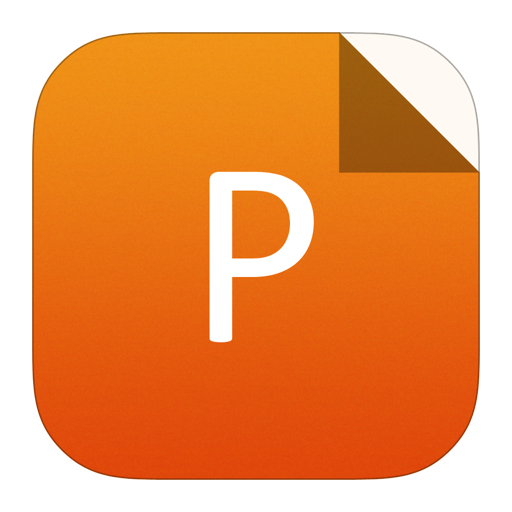
PowerPoint slide
Figure4.
(Color online) EDX spectra of (a) ZnS quantum dots, (b) 0.05 at.% cobalt doped ZnS quantum dots, and (c) 0.25 at.% cobalt-doped ZnS quantum dots.
3.4
Fourier-transform infrared spectroscopy (FTIR)
FTIR spectra of pure and cobalt-doped ZnS quantum dots are depicted in Fig. 5. This study will give a qualitative analysis of the adsorbed molecules constrained to the surface of ZnS: Co2+ quantum dots. It is clearly observed a broad peak in the range of 3200–3500 cm–1 due to the O–H stretching band due to the absorbed moisture in all the samples. The characteristic peaks appeared at 671.32, 667.54 cm–1 in the prepared samples due to the presence of sulphide in the ZnS samples. These observations are inconsistent with the previously reported work[35]. The 1399, 1427.51, 1441.52 cm–1 bands are characteristic of hydroxyl groups in the pure ZnS sample. The band at 487.72, 439.21 cm–1 is ascribed to NH2 symmetric stretching vibration[36]. The weak bands at frequencies 928.61, 904.91, and 888.54 cm?1 indicate the vibrational modes of interaction between sulfide ions in the crystal.

class="figure_img" id="Figure5"/>
Download
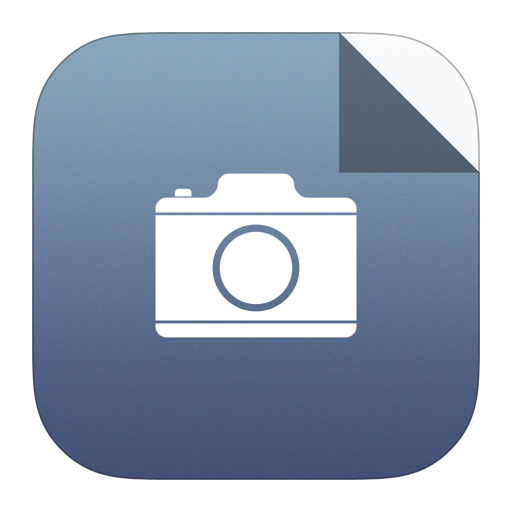
Larger image
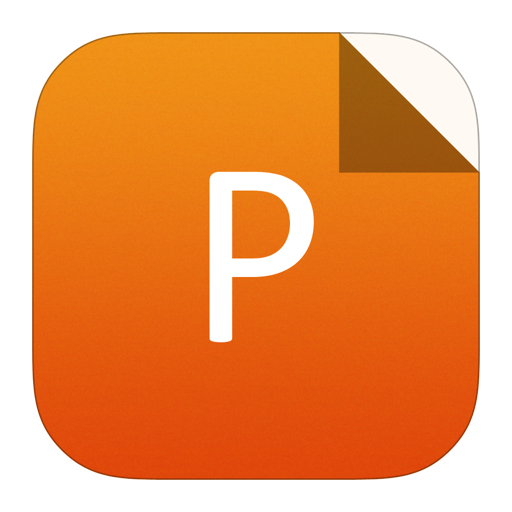
PowerPoint slide
Figure5.
FTIR spectra of (a) ZnS quantum dots, (b) 0.05 at.% cobalt doped ZnS quantum dots, and (c) 0.25 at.% cobalt-doped ZnS quantum dots.
4.
Gas sensing studies
Ammonia is used by fertilizer factories, refrigeration systems and chemical industries. A small leakage in the system can be a critical situation in the industry. Industries using ammonia should have a proper sensor that can immediately alarm to caution when the limit level of ammonia concentration crosses the maximum limit of 50 ppm[22]. This study successfully synthesized pure ZnS and cobalt-doped ZnS quantum dot sensors to detect a low concentration of ammonia. The response of the gas is calculated by the following equation[37].
$${ m{Response% }} = frac{{{R_{ m a}} - {R_{ m g}}}}{{{R_{ m g}}}} times 100,$$ ![]() |
where Ra is the resistance of the sensor in air ambience, and Rg is the resistance in exposed gas.
In present day-to-day life, the optimum temperature is essential to minimize the use of power required for operating practical devices. The ZnS-based quantum dots' response is attributed to the improved surface free energy at an optimum temperature. The increase in native surface states density also leads to the growth of sensors threshold sensitivity, which agrees with the calculated structural parameters. The sensing mechanism in the semiconductor-based materials can be ascribed to the transformation taking place in electrical conductivity/resistance due to the chemical interaction between the surface complexes of O–, O2–, reactive chemical species (S2–) and gaseous molecules[38, 39]. Adsorption of gas, transfer of charges, and desorption processes are the key factors in this mechanism.
Quantum dots are well thought out in the gas sensors to be a good candidate as the target gas molecules can be easily absorbed as they acquire a high surface area, and by means of Debye length and their grain size is compared. Thus, when the gas molecules are absorbed, these quantum dots trigger the charge transfer to improve the gas response[40]. At an optimum temperature of 70 °C when the ZnS material is exposed to air, oxygen molecules on the ZnS surface are adsorbed due to the trapping electrons from the conduction band of ZnS, ionized oxygen species such as O–, O2– are formed relatively[41–43]. Then due to the development of the space-charge region, a high resistance state in the air is observed for ZnS. On the surface of the sensing element, the chemisorbed O2 species interacted with the molecules of ammonia. In the case of ammonia, which is reducing gas, from the baseline, there is a decrease in the surface resistance of the film during the period of interaction with the target gas molecules and the reaction mechanism is shown below:
$$2{ m{N}}{{ m{H}}_3}left( {{ m{ads}}} ight) + 3{{ m{O}}^ - }left( {{ m{ads}}} ight) to {{ m{N}}_2}left( {{ m{gas}}} ight) + 3{{ m{H}}_2}{ m{O}}left( {{ m{gas}}} ight) + 3{{ m{e}}^ - }.$$ ![]() |
In cobalt-doped ZnS quantum dots, cobalt nanoparticles activate the target gas by dissociation and subsequent spill-over[44, 45] of dissociation fragments onto the gas sensing material, which causes the target gas response. In the cobalt-doped ZnS gas sensor, cobalt particles increase the molecule to ion conversion rate and the quantity of adsorbed oxygen; as a result, a deeper depletion layer is formed compared to the pure ZnS. When ammonia gas is introduced, the layer would decrease or disappear rapidly, resulting in a dramatic change in the resistance of the semiconductor materials. In a word, cobalt particles increase both the number of oxygen species and the molecule-ion conversion rate, thus significantly enhancing the ammonia sensing properties. Fig. 6 shows the gas sensing equipment in which the sensing measurement is carried out successfully:

class="figure_img" id="Figure6"/>
Download
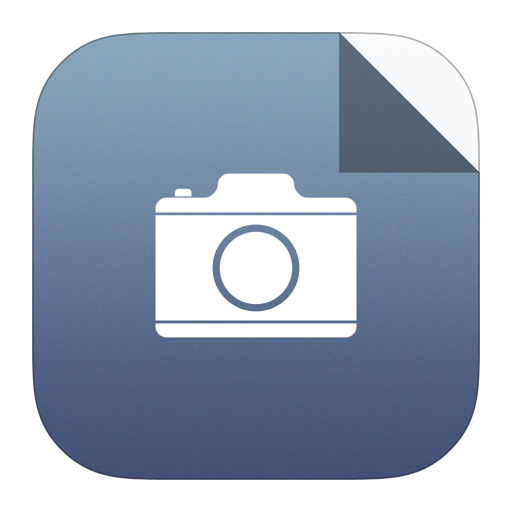
Larger image
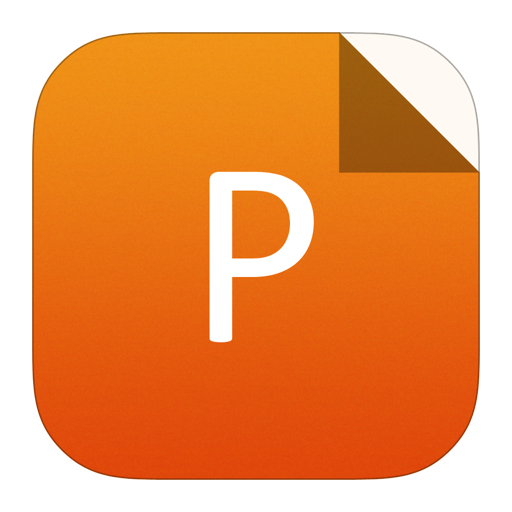
PowerPoint slide
Figure6.
Gas sensing setup.
In addition to this, due to the electron-donating reaction, there is an increase in the conductivity of metal oxide for the samples that are doped with transition metal (cobalt). Fig. 7 shows the variation of response % of different sensor elements towards 50 ppm of ammonia gas at an optimum operating temperature of 70 °C. Response at a substantially low temperature is attributed to the increased surface free energy of the nanocrystalline ZnS. It is very clear that the sensor, which is fabricated with 0.25 at.% of cobalt-doped ZnS quantum dot, has shown the maximum response. It might be due to the morphology and the surface state of this sensor being pretty good compared to the other quantum dots.

class="figure_img" id="Figure7"/>
Download
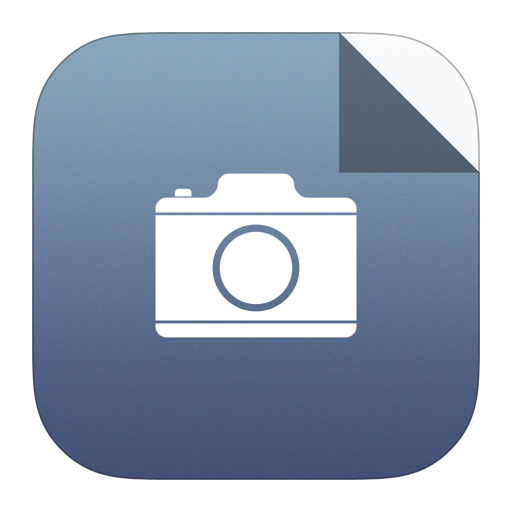
Larger image
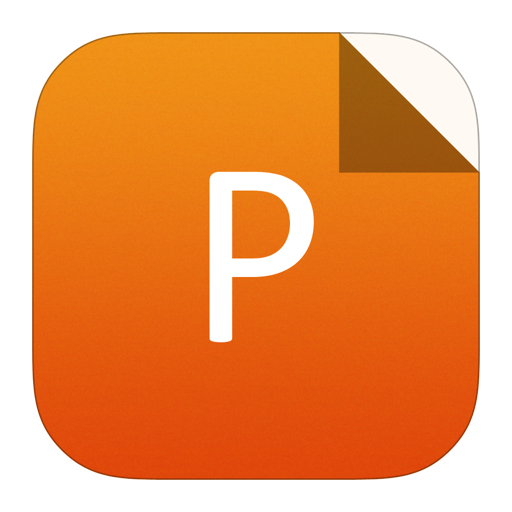
PowerPoint slide
Figure7.
(Color online) Response of pure and cobalt-doped ZnS quantum dots towards 50 ppm ammonia.
Fig. 8 shows the transient response curve of pure and cobalt-doped ZnS quantum dots. From the transient curve, response and recovery time are determined. The response time of the sensor elements towards the ammonia gas varies from 48, 35, 26 s and recovery rate as 20, 12, 28 s in the case of pure ZnS, 0.05 at.%, 0.25 at.% cobalt-doped ZnS quantum dots respectively.

class="figure_img" id="Figure8"/>
Download
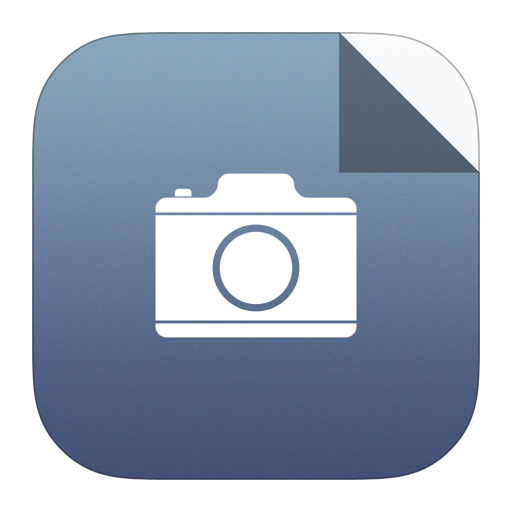
Larger image
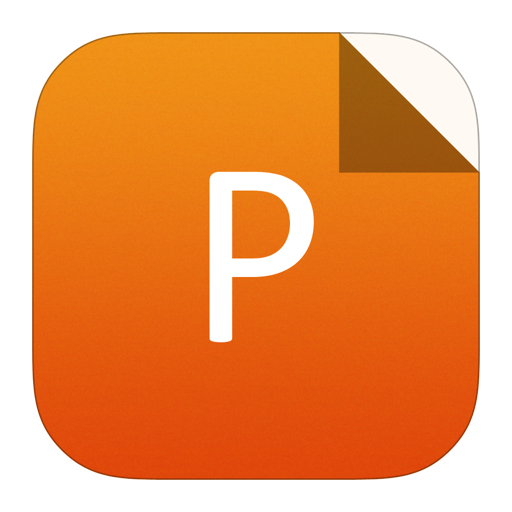
PowerPoint slide
Figure8.
(Color online) Transient response curves (a) pure ZnS (b) 0.05 at.% of cobalt-doped ZnS quantum dots (c) 0.25 at.% of cobalt-doped ZnS quantum dots.
The sensor element which is prepared 0.25 at.% of cobalt-doped ZnS exhibits stable response and recovery behaviors. We have also compared the response of this sensor element towards various concentrations of ammonia vapors with available literature. As shown in Table 2, our sensor exhibits better gas sensing properties in terms of response time and ammonia vapour concentration.
S.No | Material | Concentration (ppm) | Response time (s) | Reference |
1 | SnO2 thin film | 50 | 175 | [46] |
2 | CuO-MnO2 composite | 100 | 120 | [47] |
3 | NiO nanowire | 50 | 36 | [48] |
4 | WS2-TiO2 nanohybrids | 200 | 250 | [49] |
5 | Ni-ZnO | 750 | 46 | [50] |
6 | TiO2 modified ZnO thick film | 100 | – | [51] |
7 | Cobalt doped ZnS quantum dot | 50 | 26 | Present work |
Table2.
Comparison table of gas sensing properties different materials towards ammonia.
Table options
-->
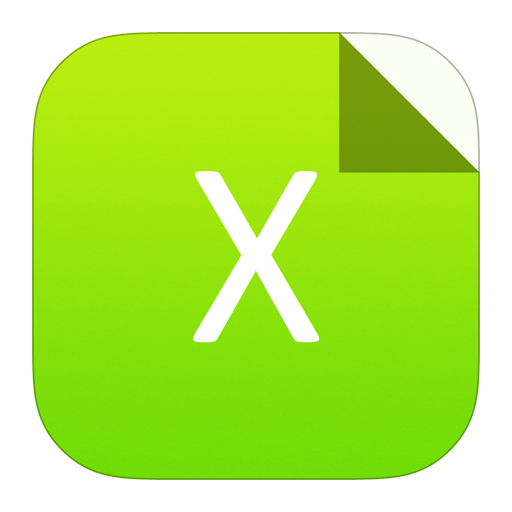
Download as CSV
S.No | Material | Concentration (ppm) | Response time (s) | Reference |
1 | SnO2 thin film | 50 | 175 | [46] |
2 | CuO-MnO2 composite | 100 | 120 | [47] |
3 | NiO nanowire | 50 | 36 | [48] |
4 | WS2-TiO2 nanohybrids | 200 | 250 | [49] |
5 | Ni-ZnO | 750 | 46 | [50] |
6 | TiO2 modified ZnO thick film | 100 | – | [51] |
7 | Cobalt doped ZnS quantum dot | 50 | 26 | Present work |
5.
Conclusions
We have successfully synthesized pure and cobalt-doped ZnS (Zn1–xCoxS, x = 0.05, 0.25 at.%) quantum dot sensors with zinc blende structure using a cost-effective co-precipitation method. The structural studies from XRD reveal that the formation of well-crystalline quantum dots with a crystallite size less than 5 nm. EDX analysis revealed Zn and S elements in the pure sample Zn, S, Co, in cobalt-doped samples. SEM images reveal the smaller grain size and spherical shape of cobalt-doped samples, while TEM confirms the particle size is in the range of 6 nm. The morphology and surface state is increased with increasing cobalt concentration. Spectroscopic investigation using FTIR analysis shows the changes observed at different vibrational modes. The gas sensing characterization of pure and cobalt-doped ZnS quantum dots were investigated towards 50 ppm of ammonia. Among these samples, 0.25 at.% cobalt doped ZnS quantum dots were shown the maximum response of 82% towards 50 ppm of ammonia vapors at an operating temperature of 70 °C with a response time of 26 s and recovery time of 28 s. We firmly believe that this sensor can be suitable to detect the low concentration of ammonia vapours.