1.
Introduction
Integrated photonics is a hopeful way to solve the bottleneck of energy and speed, which mainly focuses on devices like laser, modulator, photodetector at the present stage[1-14]. Microring modulator performing as the basic building block in the photonic integrated circuit (PIC), has received considerable attention in recent years. Features include small footprint, low drive voltage and high extinction ratio, which enable the application possibilities of microring modulators in on-chip optical interconnections. There are colorful studies about microring modulators reported, focusing on high speed, small scale, low voltage, low-power consumption, high order modulation, hybrid integration and novel structures[5, 15-27]. These devices are mainly fabricated on platforms like the silicon on insulator, lithium niobate and polymer[15, 19-22, 28-35]. The basic characteristics of the microring resonator largely decide the performance of the microring modulator, which is hard to change once fabricated on these platforms. However, the attributes like quality factor and coupling coefficient of the microring resonator could be controlled by adjusting the gain in the microring resonance cavity[36, 37]. Based on the tuning of gain, an ultra-low Vpp and high-modulation-depth microring modulator is implemented on an indium phosphide (InP)-based platform, enabling more tunability, designability and flexibility in PIC[38-41]. And compared with the reported InP microdisk modulator, circular microresonators modulation, and slot waveguide microring modulator simulation, the proposed microring modulator shows lower Vpp and higher modulation depth[12-14].
Here, we designed a microring modulator, in which the microring resonator incorporates a 500 μm long semiconductor optical amplifier (SOA) and couples to the bus waveguide through a multimode interference (MMI) coupler. The SOA is implemented in the device to supply the gain and compensate for the propagation loss in the ring, which can adjust the transmission spectra of the resonator to achieve high-modulation depth. And the quantum-confined Stark effect (QCSE) in the microring modulates the refractive index to realize the modulation. Traveling wave electrodes are designed to inject the electrical signal to the SOA to realize the modulation. The free spectral range (FSR) of the device is 19.5 GHz around the resonance wavelength. The extinction ratio (ER) of the resonator is 43.3 dB and the resonance wavelength is 1550.002 nm. The Vpp is as low as 11 mV and the modulation depth rises to a maximum of 42.5 dB. A 137.5 mA bias current is set to the SOA and a 6.6 GHz bandwidth is achieved. Eye diagrams are measured in which pseudo random binary sequence (PRBS) 27 – 1 non-return-to-zero (NRZ) data signals with speed up to 12 Gb/s are applied to the proposed device. The unique designed microring modulator shows a way of realizing the ultra-low Vpp and high modulation depth. And the performance could be improved with further research.
2.
Design and fabrication
Fig. 1 shows the schematic diagram of the proposed microring modulator. The device is fabricated on the InP platform and mainly consists of a racetrack microring, which includes an SOA and is coupled to a bus waveguide through a MMI coupler. The incorporated SOA is 500 μm long and designed to supply 10 dB gain at most. Maximum 20 GHz traveling wave electrodes are employed to SOA to realize the modulation, which are from the standard process design kite (PDK, supplied by HHI, Gemany). To achieve the maximum modulation speed, bias ports of the electrodes could be used bidirectionally, in which one of them should be terminated with a 50 Ω impedance. A 2 × 2 MMI coupler is used to connect the racetrack microring and the bus waveguide due to the good fabrication tolerance of the MMI coupler. Two spot size converters (SSCs) are adopted at the two ports of the bus waveguide, respectively. Distance between the two SSCs is 250 μm, according with the separation of the standard single-mode fiber array, which is convenient for butt-coupling test and package.

class="figure_img" id="Figure1"/>
Download
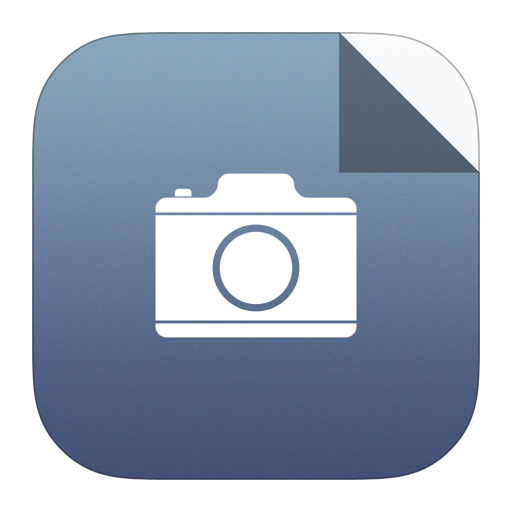
Larger image
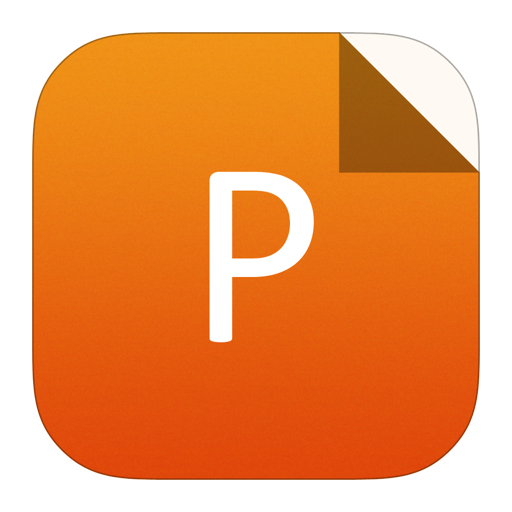
PowerPoint slide
Figure1.
(Color online) Architecture of the microring modulator.
The bending radius of the racetrack microring is 300 μm and the perimeter is 4.14

An optical microscope image of the device with a scale bar of 200 μm is shown in Fig. 2(a), in which the SSCs are outside the view because of the minimum magnification times limitation. The way to couple the optical and electrical signal is illustrated in Fig. 2(b) that a two ports standard single-mode fiber array (FA) is coupled to the two ports of the microring modulator through SSCs. Probe 1 carries the electrical signal to one port of the traveling wave electrode on the chip, while probe 2 connects a 50 Ω impedance to the other port of the electrode. There is a copper block under the chip for heat dissipation.

class="figure_img" id="Figure2"/>
Download
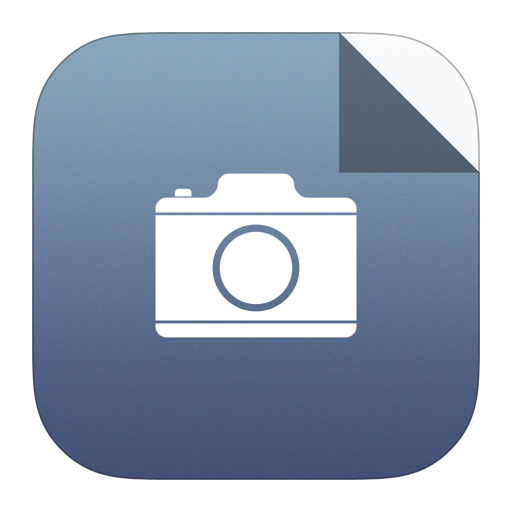
Larger image
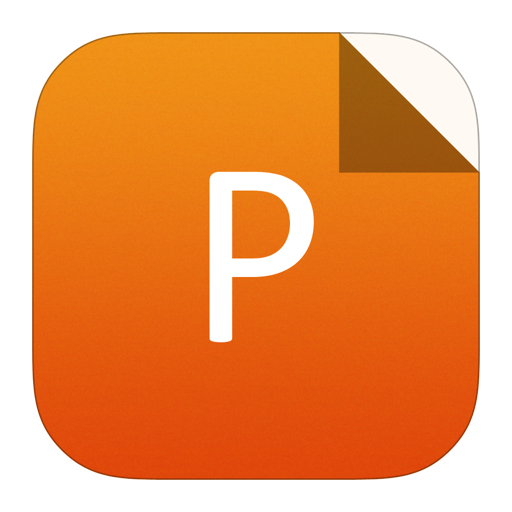
PowerPoint slide
Figure2.
(Color online) (a) A photograph of the microring modulator with a scale bar of 200 μm
3.
Characterization and experimental results
To characterize the modulator, the I–V curve of the SOA is measured when the 50 Ω impedance is disconnected because the SOA is usually driven by injection currents. And the I–V curve could relate currents with the corresponding voltage. A source-measure unit (SMU) and a bias tee are applied to test the I–V curve which is plotted and fitted in Fig. 3. Only a maximum 190 mA current is applied, avoiding the damage of the SOA.

class="figure_img" id="Figure3"/>
Download
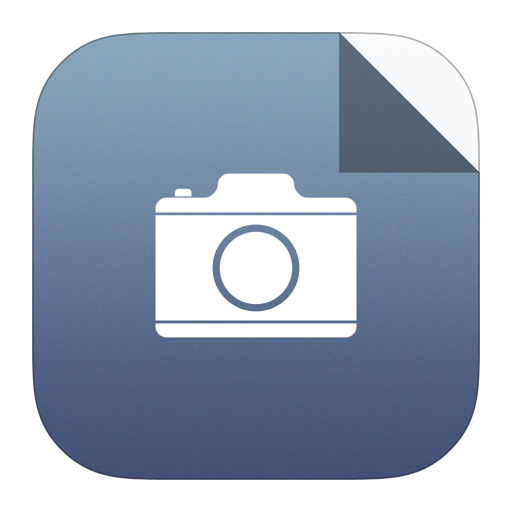
Larger image
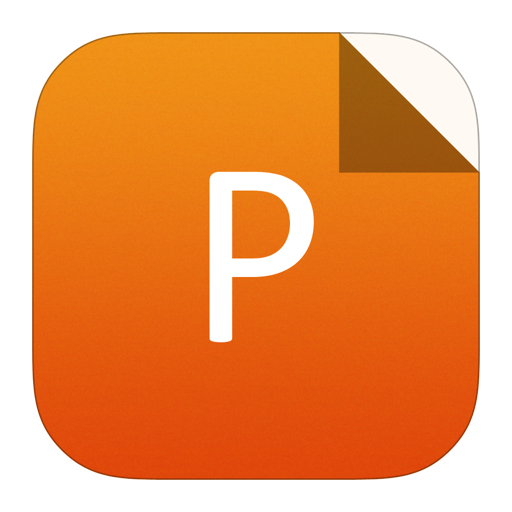
PowerPoint slide
Figure3.
The I–V curve of the active resonator when the 50 Ω resistance is disconnected.
Then a high-resolution optical vector network analyzer (OVNA) is established to measure the static transmission and phase spectra of the designed microring modulator. An optical carrier generated by the laser (Yenista, Tunics T1005-HP) and modulated by radio frequency (RF) signal from the electrical vector network analyzer (ZVA 40) through the phase modulator (EOSPACE, 40G). And an optical double-sideband (ODSB) signal is produced and one sideband is filtered out by the tunable filter to generate an optical single-sideband (OSSB). The OSSB signal is amplified by an erbium-doped fiber amplifier (EDFA) and coupled to the chip via the input port SSC. After the transmission through the device, the OSSB signal is injected to one photodetector (FINISAR XPDV2120R) to beat and generate RF signal. The RF signal is fed back to the electrical vector network analyzer (EVNA) after the amplification of an electrical amplifier (EA). The S21 curve exhibits the transmission spectra of the device and the resolution is decided by the EVNA, which could achieve tens of Hz resolution. The characterization of the device could be more precisely measured by this OVNA[24, 42].
The transmission spectra of the modulator at four different injection currents are displayed in Fig. 4(a). There are four lines representing the transmission spectra of the device for 136.74, 137.24, 137.74, and 138.24 mA injection currents. When the current of SOA is set to 138.24 mA, the microring resonator operates around the ideal critical coupling point, in which the ER is over 43 dB. As improving the injection current, the gain of SOA could compensate for the loss in the microring. The current is delicately tuned to adjust the relation between the loss coefficient and coupling coefficient, while the transmission spectra could be observed in real time through OVNA. Once the coupling coefficient between the bus waveguide and the microring equals the loss coefficient in the microring, the active microring resonator will be under critical coupling condition, wherein the ER is theoretically infinite at the resonance wavelength. To estimate the coupling state of the microring resonator, the phase data recorded by the EVNA is processed with the formula:
m{Phase}}left(pi
ight)= $



class="figure_img" id="Figure4"/>
Download
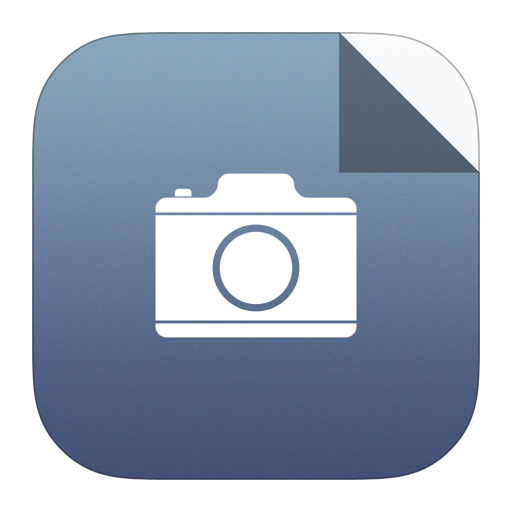
Larger image
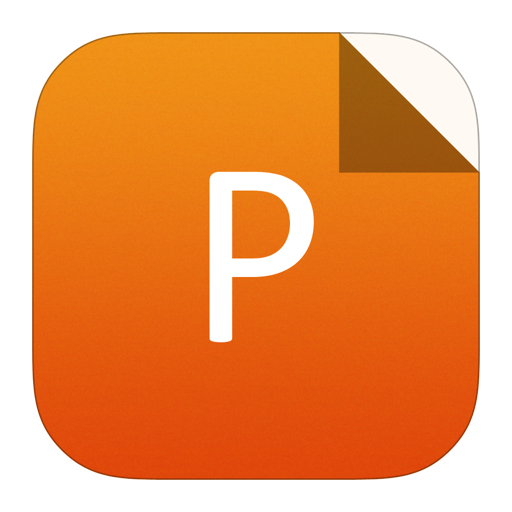
PowerPoint slide
Figure4.
(Color online) (a) The transmission spectra of the microring modulator under four injection currents applied to the SOA. (b) Modulation depth and insertion loss with currents from 136.74 to 138.24 mA.
To measure the modulation bandwidth, a 50 Ω impedance is applied to the chip to reduce the reflection of the microwave signal. The experiment link is shown in Fig. 5. A 1550.005 nm carrier wave is coupled to the modulator to enable the microring modulator operate at the linear operating point. And a 186.6 mA bias current is applied, in which a 137.5 mA will be set to SOA according to the I–V curve in Fig. 3(a) and the tested static transmission spectra in Fig. 4(a). Using the EVNA to measure the S21 of this modulator from 0 to 20 GHz with the power of 9 dBm, which is presented in Fig. 6. The 3 dB bandwidth of the microring modulator is about 6.6 GHz. Because the traveling electrodes are from the standard PDK, the bandwidth is mainly limited by the QCSE. What is more, optimizing the electrode and waveguide design, improving the growth of InP quantum wells, and reducing the radius of the microring could improve the 3 dB bandwidth of the microring modulator.

class="figure_img" id="Figure5"/>
Download
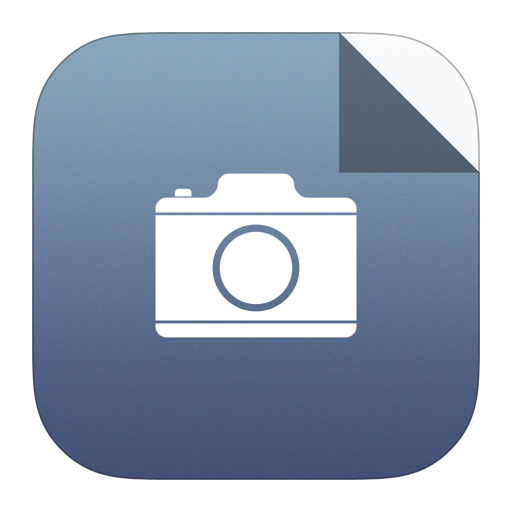
Larger image
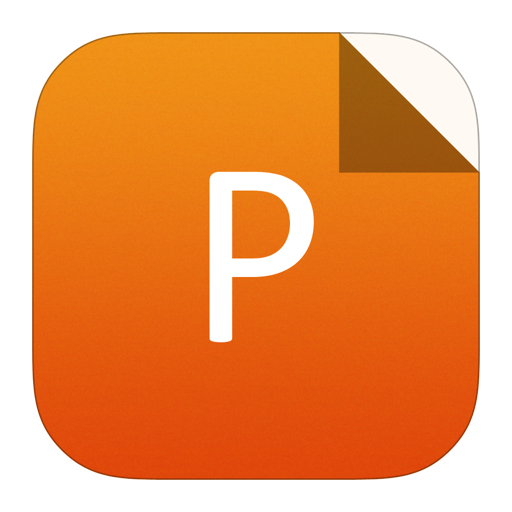
PowerPoint slide
Figure5.
(Color online) The experiment schematic of the modulation bandwidth measurement.

class="figure_img" id="Figure6"/>
Download
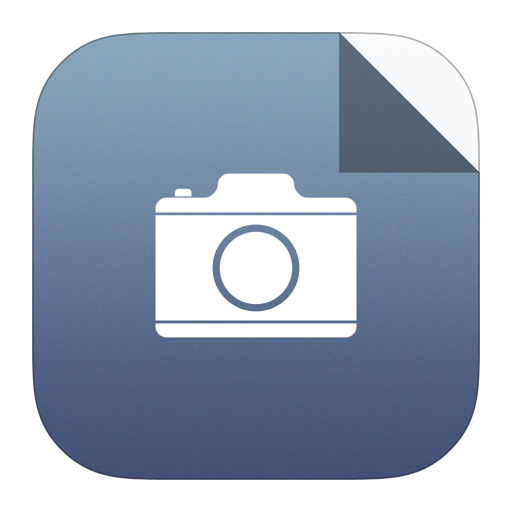
Larger image
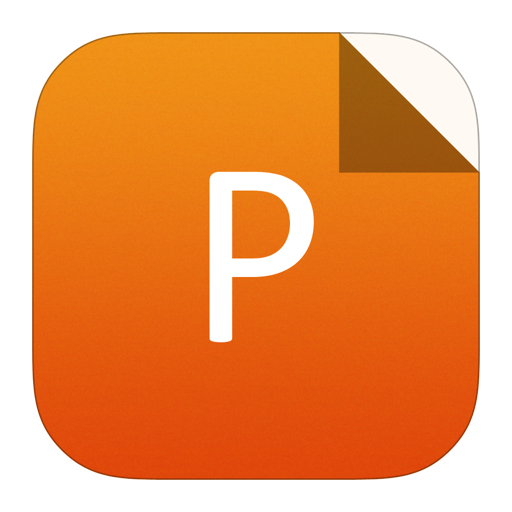
PowerPoint slide
Figure6.
The bandwidth of the device when it works as an electro–optic modulator.
Applied in the back-to-back configuration, eye diagrams are measured. Figs. 7(a)–7(c) show measured eye diagrams under the transmission of 4, 8, and 12 Gb/s PRBS 27–1 NRZ data signals, respectively. As can be seen in Fig. 7, the eye could open clear when the modulating signal reaches 8 Gb/s. Nevertheless, when the data speed exceeds 10 Gb/s, the eye diagrams are hard to open because of the noise and the nonlinearity in the test link. The proposed microring modulator may be employed in the optical communication link. To improve the modulation speed, the fabrication process and electrodes design could be modified.

class="figure_img" id="Figure7"/>
Download
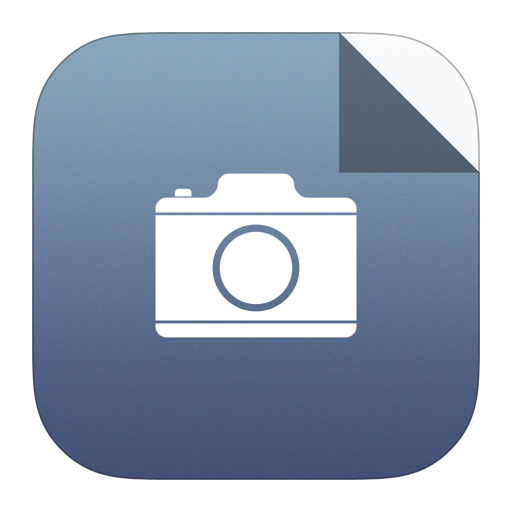
Larger image
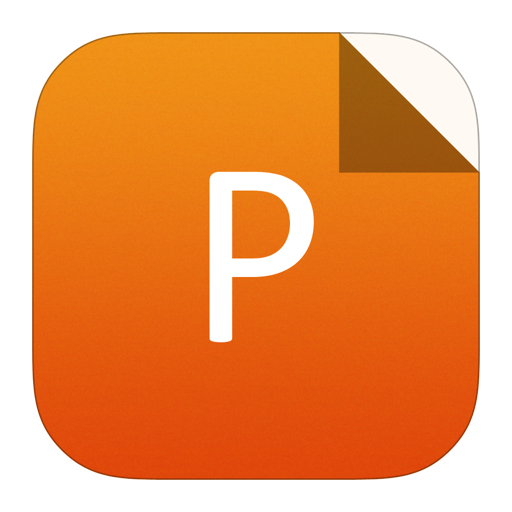
PowerPoint slide
Figure7.
(Color online) Measured eye diagrams of the device under 4, 8, and 12 Gb/s bit rates.
4.
Conclusion
In conclusion, an ultra-low Vpp and high-modulation-depth InP-based electro–optic microring modulator is designed, fabricated, and characterized. The Vpp reaches as low as 11 mV and the modulation depth is 42.5 dB in maximum. The microring modulator shows a 6.6 GHz bandwidth and measured eye diagrams up to 12 Gb/s bit rate demonstrate the ability of the proposed device in the optical communication link. The realization of the microring modulator broadens the applications and promotes the designability of the basic building blocks in PIC.
Acknowledgements
This work was supported by the National Natural Science Foundation of China (61535012, 61925505) and the National Key R&D Program of China (2018YFB2201900). The authors acknowledge VLC, Spain, for their help in designing this chip. The authors acknowledge HHI, Germany, for the chip fabrication.