1.
Introduction
The explosive development of the internet of things (IoT) has promoted the progress of portable electronic products. Smartphones, notebook computers, and electronic watches are expected to be continuously revolutionized with desirable form factors including miniaturization, flexibility, and lightweight. Meanwhile, with the increasing demand for personalized healthcare and remote diagnostics, wearable bioelectronics such as smart wristbands and intelligent glasses have come into vogue. These devices are connected to the internet and combined with various software applications to enable users to perceive and monitor their health status and surroundings. Research efforts on bioelectronics have been intensively devoted to the field of bio-medicine, IoTs and health monitoring with the rapid progression of electronic technology[1-3].
However, one of the challenges lies in the novel flexible energy storage devices, without which the smooth functionalization of various flexible electronics cannot be guaranteed. Conventional energy storage devices are rigid and robust. When bending and folding, it is easy to cause cracks on collectors, affect electrochemical performance, and even lead to electrical short-circuit, resulting in serious safety problems. Therefore, flexible energy storage devices that can withstand mechanical deformation and retain their electrochemical properties have become a research hotspot. Impressive progress has been achieved in developing energy storage devices in a variety of flexible formats with research efforts in material engineering, device structural design, and system integration[4-7]. The practical requirements of wearable electronics put forward the demands on self-powered integrated systems, which convert clean energy into electricity and support system power supply without external charging. For instance, photovoltaic devices have been adopted to collect solar energy, thermoelectronics for thermal energy, triboelectric and piezoelectric generators for mechanical energy, respectively.
In this review, we mainly focus on the recent research progress of flexible energy storage devices (e.g., batteries and supercapacitors), self-powered systems, and their applications in integrated wearable bioelectronics, as shown in Fig. 1. First, an overview of commonly adopted methods for fabricating flexible energy devices will be provided. They are classified as chemical methods and physical methods, which will be discussed in Section 2. Moreover, representative reports on self-powered systems based on flexible energy devices will be introduced in Section 3, including their working principles and novelties. In Section 4, biosensing devices’ physiological and physical signal detection that can be integrated with flexible energy devices are summarized. Discussion on future perspectives of flexible energy storage devices will be included in Section 5.

class="figure_img" id="Figure1"/>
Download
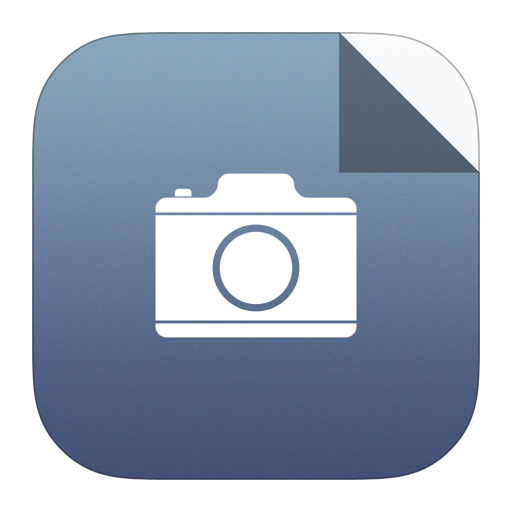
Larger image
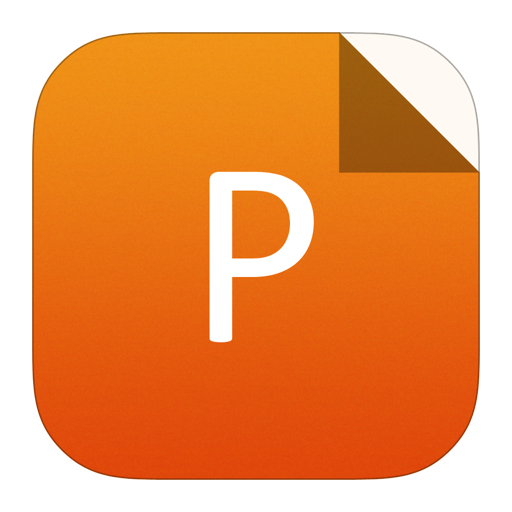
PowerPoint slide
Figure1.
(Color online) The fabrication methods and energy sources for flexible energy storage devices and their applications in wearable biosensing[8–15].
2.
Fabrication methods
The commonly adopted fabrication methods for flexible energy storage devices are summarized in this section. Generally, material synthesis and device fabrication can be categorized into chemical methods and physical methods, respectively. The morphology and properties of active materials with energy storage capability can be modified via various approaches. As for chemical methods, the morphology modification of the active material can be achieved by changing the reaction conditions, leading to optimizing the device performance. Besides, it has higher compatibility with complicated micro-nanostructures. Physical methods, such as coating and sputtering, provide approaches for material loading and device construction on flexible substrates, usually in the form of thin films[16].
2.1
Chemical method
Hydrothermal synthesis: Hydrothermal synthesis refers to a method of preparing materials by dissolving and recrystallizing the powder with water as the solvent in a sealed vessel. In recent years, it is reported that metal-organic frameworks, nanostructures, hybrids of organic polymers, metal oxides, and other substances can all be formed on the substrate by hydrothermal synthesis[17-19]. As shown in Fig. 2(a), Wu and coworkers utilized a liquid phase reaction to primarily grow dodecahedral 2-methylimidazole cobalt (ZIF-67) crystal on carbon cloth. Then the ZIF-67 crystal is gradually transformed into a hollow manganese dioxide (MnO2) polyhedron through a hydrothermal reaction[20]. When tested, the aqueous battery showed a high reversible capacity of 263.9 mA·h/g after 300 cycles, and its performance far exceeded that of the commercial MnO2 electrode. Furthermore, different nanomaterials such as nanospheres, nanocomposite films and nanoneedle arrays are frequently fabricated as anodes and cathodes via hydrothermal synthesis[21-23]. The reaction conditions of the hydrothermal synthesis are relatively facile and are highly controllable to ensure the stability of the synthesis. For instance, Wang’s group summarized controlled hydrothermal synthesis of lithium iron phosphate (LiFePO4) cathode for LIBs, and the LiFePO4 nanorods exhibited a high capacity of 155 mA·h/g at 0.5 C and sustainable capacitance retention of 80%[24].

class="figure_img" id="Figure2"/>
Download
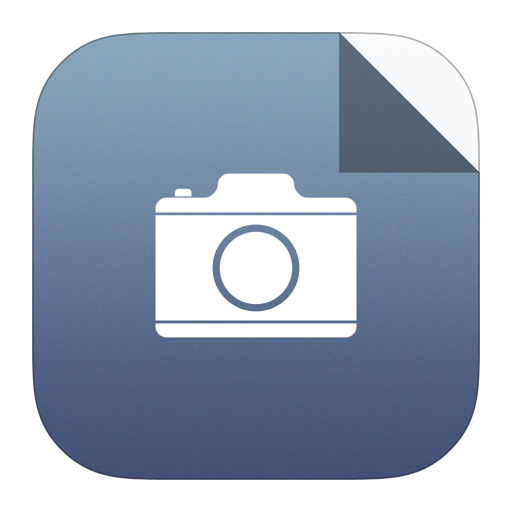
Larger image
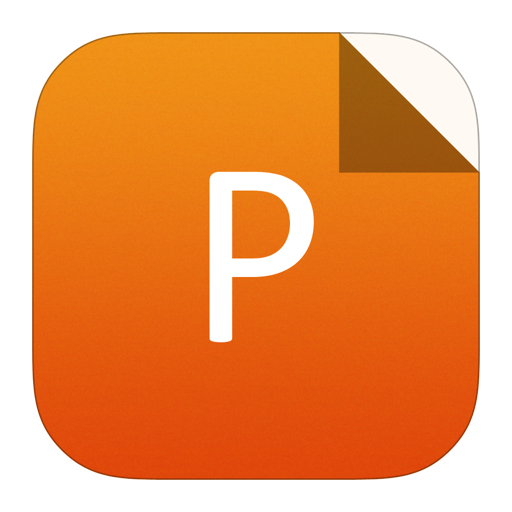
PowerPoint slide
Figure2.
(Color online) Chemical methods for flexible energy storage devices fabrication. (a) Two-step hydrothermal synthesis of MnO2 nanosheet-assembled hollow polyhedrons on carbon cloth[20]. (b) Metal-like conductive paper electrodes based on Au nanoparticle assembly followed by nickel electroplating[10]. (c) A microwave-assisted rapid sysnthesis of nickel-iron-based catalysts for rechargeable zinc-air battery[32]. (d) Synthesis of 3D nanofiber electrode via CVD[36].
Electrochemical deposition: By applying an electric potential in an ionic solution to trigger a reduction or oxidation reaction, a layer of desired materials can be directly deposited onto the conductive substrate. Generally, it relies on a three-electrode system with the working electrode, the counter electrode, and the reference electrode. The unique advantages of electrochemical deposition include: (1) During the metal reduction process, the potential difference, solution concentration, and ambient temperature can be adjusted to control the morphology of the product. (2) The electrolyte can be mixed with various metal salt solvents, which enables composite active materials. (3) The reaction conditions are relatively mild, and the synthesis can be completed at room temperature and atmospheric pressure. Therefore, it is widely used for flexible electrodes functionalization with metals, metal oxides and polymers. For instance, Fig. 2(b) illustrates that metal nanoparticle layers were assembled on insulating paper via layer-by-layer metal electrodeposition to prepare conductive paper, which retained the porous structure of the original paper and delivered an area capacitance of 811 mF/cm2[10]. In Singh’s work, transparent core-shell MnO2 and gold (Au) coated nanofiber network electrodes were fabricated by electrodeposition, which showed mechanical flexibility, high energy density, high transparency, and long-term cycling stability[25]. A flexible and transparent supercapacitor was further developed and delivered a high energy density of 0.14 μW·h/cm2, along with a high areal capacitance of 2.07 mF/cm2.
Microwave-assisted synthesis: Microwave-assisted synthesis achieves material deposition via chemical reactions within the electromagnetically heated electrolytes. It can be applied to the synthesis of porous materials, inorganic complexes, nanocrystalline particles, organic compounds and so on[26-30]. Compared with conventional hydrothermal synthesis, microwave passes through the material and provides energy. Thus, the heating process is rapid and uniform, which enables fast and energy-efficient synthesis. Nonetheless, it is relatively challenging to precisely control the reaction temperature and form factors of the produced materials. Jeon’s group endowed pristine carbon cloth with large surface area, porosity and high specific capacitance via microwave-assisted functionalization. The supercapacitor based on carbon cloth delivered a maximum energy density of 64 μW·h/cm2 at a power density of 1 mW/cm2 with long-term cycling[31]. Fig. 2(c) illustrates the exploration of advanced nickel-iron-based catalysts for rechargeable zinc-air batteries via microwave-assisted synthesis[32]. As a result, four flexible zinc-air batteries were connected in series to power a mobile phone and showed high cyclic stability under different bending states.
Chemical vapor deposition (CVD): When two or more gaseous raw materials are mixed into a reaction chamber, the chemical reaction can be triggered to form materials on the substrate surface. It is the most widely used technology in the semiconductor industry for the synthesis of materials such as Si nanofiber, graphene, and carbon nanotube (CNT)[33-35]. As shown in Fig. 2(d), Zhang’s group fabricated the three-dimensional (3D) nanofiber electrode via CVD. It provided relatively high controllability, and the as-fabricated supercapacitors exhibited high capacitance stability of 96.5%[36]. In addition, Zang and his coworkers manufactured novel 3D CNT-graphene and 3D CNT-CNT network structures as electrodes, which showed enhanced energy density for supercapacitors[37]. Besides, nanotube and nanofiber films prepared by CVD methods have been widely adopted as anodes for lithium-ion batteries (LIBs)[33, 38]. For instance, Yang et al. presented an aprotic lithium-oxygen battery with a high discharge capacity of 11512.4 mA·h/g and long cycle life of 130 cycles[39]. CVD provides an inexpensive and facile method to fabricate functionalized flexible materials for energy storage applications without the use of special or toxic atmosphere, which makes it popular in industry.
2.2
Physical method
Coating: A slurry consisting of active electrode powder with conductive additives and binders is prepared and smeared onto the substrate. Post-annealing or drying is normal. It is one of the most frequently adopted methods to fabricate flexible electrodes. For instance, Manjakkal and coworkers designed a sweat-activated flexible supercapacitor using the coating method[40]. The valuable device exhibited energy and power densities of 1.36 W·h/kg and 329.7 W/kg, respectively. Besides, Dai's group utilized the coating method to fabricate supercapacitors based on activated carbon. The devices showed significantly improved cyclic stability and rate capability[41]. Fig. 3(a) shows that flexible CNT-based cathodes with controllable thicknesses are successfully fabricated via a facile blade-coating method, and the pouch cell shows impressive cyclic stability under both balanced state and bent state[42].

class="figure_img" id="Figure3"/>
Download
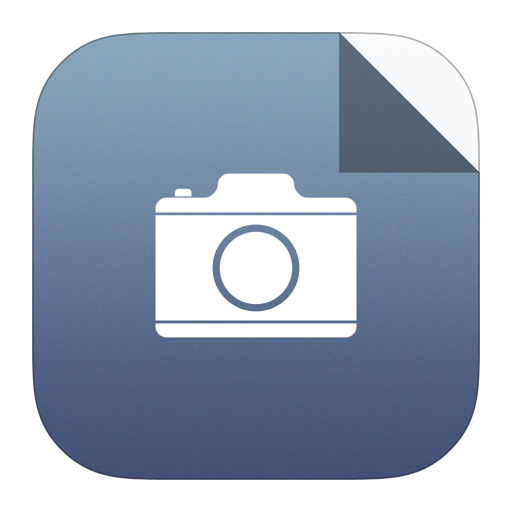
Larger image
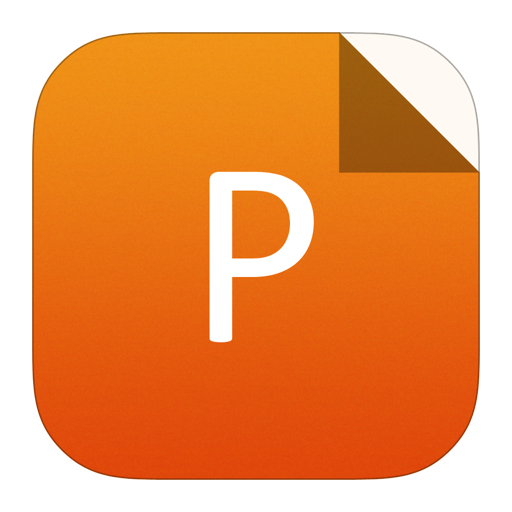
PowerPoint slide
Figure3.
(Color online) Physical methods for flexible energy storage devices fabrication. (a) The coating process to achieve flexible CNT-based cathodes[42]. (b) Infiltration of electrospun porous polyimide nanowires for sulfide solid elelctrolyte membranes[44]. (c) Fabrication of graphite/Si hybrid electrode via sputtering[46]. (d) Schematic of the 3D printed interdigital electrodes for micro-supercapacitors[11].
Infiltration: Infiltration as a mild and low-cost material loading process has been widely employed for porous materials, such as woven cloth, paper, or sponges. Thin films of materials can form on the surface of the substrate. For instance, polyaniline (PANI) has been loaded on the functionalized carbon cloth in the diluted solution via infiltration[20, 30]. It then served as a flexible electrode in a symmetrical supercapacitor, which presented the area capacitance of 350.8 F/g and high capacitance retention of 90.8% for 10 000 cycles. In Song’s work, a lithium-sulfur-infiltrated catholyte was efficiently infiltrated into the carbon cloth during cell fabrication[43]. This carbon cloth cathode was reported with a high areal capacity of 3.2 mA·h/cm2 for 200 cycles with a sulfur loading of 6 mg/cm2, and the battery cells remained functioning even after 300 factitious bending cycles. Moreover, Kim et al. fabricated all-solid-state LIBs using electrolyte-infiltrated polyimide film, which exhibited promising electrochemical performance (146 mA·h/g) as well as excellent thermal stability (up to ~400 °C)[44]. Fig. 3(b) illustrates the fabrication of the sulfide solid electrolyte membrane by infiltrating electrospun polyimide nanowires. Infiltration shows its compatibility and scalability for manufacturing composite electrodes on a large scale.
Sputtering: Sputter deposition involves ejecting material vapor from a target source onto the substrate. Although the process is of high cost and relies on complex equipment, it can well control the thickness and quality of the thin films. For instance, pseudocapacitive materials can be conformally coated on self-supported CNT-aligned films by magnetron sputtering to fabricate fiber-shaped supercapacitors[45]. Such self-supported materials deposited by magnetron sputtering can be widely used for wearable bioelectronics. Besides, it is capable of materials loading on substrates with micro/nanostructures. Fig. 3(c) illustrated the two-step sputtering method to prepare graphite/silicon hybrid electrodes on 3D current collectors for flexible batteries[46]. A remarkable enhancement of 19.7% on specific capacity was obtained, and the overall capacity of 108 mA·h/g at 0.5 C was achieved.
3D printing: 3D printing is an additive manufacturing method to construct 3D objects in a layer-by-layer manner following the designed 3D model. Therefore, it can fabricate 3D objects with attractive geometric characteristics based on a variety of materials, including metals, plastics or composite materials, etc.[11, 47, 48]. Orangi and coworkers adopted 3D printing to construct electrode patterns with conductive ink[11]. Fig. 3(d) shows the schematic of printing interdigital electrodes, and the height of the printed metal carbides and nitrides (Mxene) ink electrodes can be increased by printing additional layers. The printed solid-state micro-supercapacitors exhibited excellent electrochemical performance with a surface capacitance of 1035 mF/cm2. In addition, a variety of high-quality batteries, including LIBs, nickel-iron batteries, iron (III) periodate and zinc batteries, have shown distinct advantages via 3D printing[49-51]. For instance, Lee’s group fabricated yarn-type LIBs by direct ink writing-based 3D printing technology[52]. The technology facilitates its integration into commercial fabrics such as woolen gloves and develops a new strategy for next-generation smart fabrics.
3.
Self-powered systems
A self-powered system is defined as a system that operates by utilizing the ambient energy presenting in the system environment without external charging[53]. Especially for wearable electronics, the self-power capability can elongate the device duration time and reduce the recharging frequency[54, 55]. However, energy harvesting and conversion from the environment might result in fluctuations in power output, which might fail to fulfill the requirements of sustainable power supply for electronic devices. To tackle this challenge, the integration of flexible energy storage devices, such as batteries or supercapacitors, can serve as energy buffers and release the stored energy when required. Here, the advances of flexible energy storage devices integrated with energy harvesting components, including solar cells, thermal cells and mechanical nanogenerators will be summarized.
3.1
Solar energy
Solar energy is an ancient energy source with universal harmlessness and long-term sustainability. The design of flexible power supplies that integrate batteries and amorphous silicon solar modules have been frequently reported. Among these batteries, LIBs, zinc-ion batteries and aluminum-air batteries are commonly used to power health-monitoring devices[56-59]. Ostfled et al. designed a self-powered system that combines a photovoltaic (PV) module and a LIB composed of a printed graphite-based anode and a lithium oxide cathode[56]. By selecting a load duty cycle appropriately, the system could balance the current between the battery and the PV module to maintain a constant charge. Finally, the battery was used in a pulse oximeter after 600 mechanical bending cycles, and its capacity remained at least 90%, proving its effectiveness as a power source for wearable sensing systems. The system can be integrated into wearable devices such as jacket sleeves, bags and travel mugs with excellent flexibility.
Similarly, solar energy can also be stored in supercapacitors as shown in Fig. 4(a)[60]. Fan’s group successfully realized a printable and flexible self-powered system utilizing solar energy to drive a gas sensor. The solar energy can also be stored in supercapacitors and released to ensure continuous operation when interruption from illumination variation occurs. Dong et al. also manufactured and described a printable dye-sensitized solar cell (DSC) integrated with a supercapacitor[61]. Under sunlight exposure, the supercapacitors were charged by the current generated in DSC and then discharged with a capacitance of 0.14 mF/cm2. The electrical performance was tested outdoors under different extreme bending conditions. The stable data highlights the device’s performance under various extreme mechanical load conditions and paves the way for future highly flexible integrated energy systems.

class="figure_img" id="Figure4"/>
Download
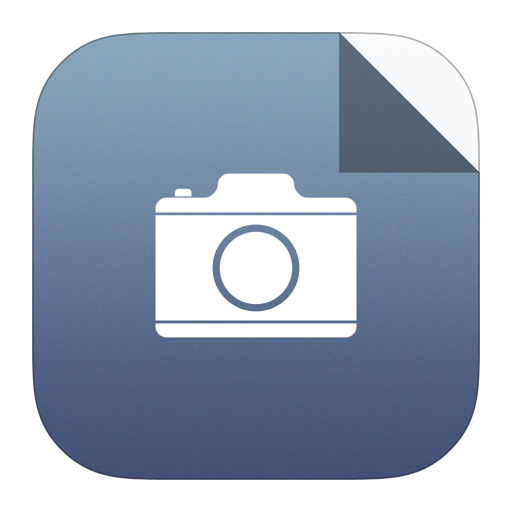
Larger image
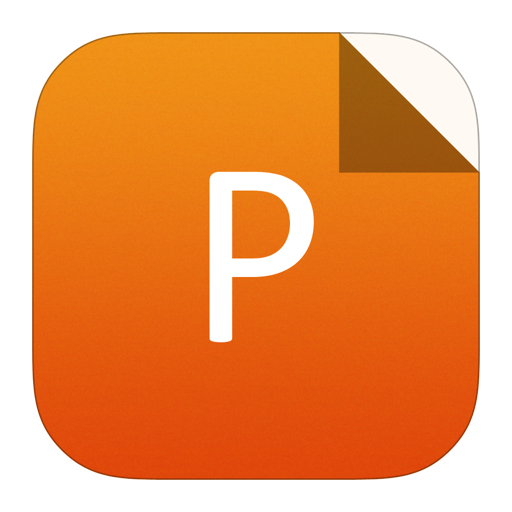
PowerPoint slide
Figure4.
(Color online) Self-powered systems consists of flexible energy storage devices and energy harvesting components. (a) Schematic of a printable self-powered system consists of solar cells, supercapacitors and gas sensor[60]. (b) Design of a thermocell for harvesting body heat and charging supercapacitors[63]. (c) Self-powered cloth consists of TENG, supercapacitor and wearable sensor[69]. (d) An all-solid-state self-powered system with high performance PENG using a particular mesoporous film[73].
3.2
Thermal energy
Thermal energy can be collected around our environment and from human bodies as well. By setting a temperature difference between the edges of two semiconductors of different properties, it generates a direct-current voltage at both ends. Thermoelectric modules can be used as nanogenerators based on the Seebeck coefficient, which are electrically in series and thermally in parallel to function uninterruptedly 24 h a day[62].
Body thermal energy is one of the easily accessed energy sources for wearable bioelectronics and has attracted increasing research interest. Fig. 4(b) illustrates a thermocell for harvesting body heat and can provide constant power for supercapacitors reported by Liu and coworkers[63]. It utilized the temperature gradient between the two surfaces of an object, namely, the thermoelectric effect (TE), to create a typical TE module. Connected with multiple p–n cells in series, sandwich structures of n-type and p-type semiconductors were successfully matched in these thermocells, and an incremental potential to 0.34 V at ΔT = 10 K was obtained. Furthermore, a supercapacitor was charged by collecting body heat and then was applied to illuminate green light-emitting-diodes (LEDs). Wang’s group fabricated a LIB for simultaneously stored the harvested thermal, mechanical and solar energies[64]. Therefore, stable electrical energy at comparable output power can be achieved compared with self-powered systems consisting of only active harvesters such as triboelectric or piezoelectric generators.
3.3
Mechanical energy
Triboelectric nanogenerator (TENG): The triboelectric nanogenerators convert mechanical energy based on the combination of the triboelectric effect and electrostatic induction. Various micro/nanostructured materials have been adopted to realize TENG-supercapacitor systems such as thin films, foams, soft rubbers and fabrics[8, 65-69]. Fig. 4(c) shows a self-charging power textile in one piece of cloth with excellent mechanical flexibility reported by Pu et al.[69]. It consists of three functional units: the TENG fabric for energy harvesting, the supercapacitor fabric for energy storage and wearable electronics for sensing. The self-charged power textile achieved high capacitance (72.1 mF/cm2) and stable cycling performances (96% for 10 000 cycles). However, the system showed a limitation of unstable energy output and a short operational time. Chun et al. proposed an all-in-one self-powered system for touch sensing with flexible and transparent electrodes[70]. The TENG component could harvest high electric power (1.45 mW/cm2 with 17 kPa) when touched. The supercapacitor exhibits a high capacitance of 3.83 μF/cm2 and stable performance without degrading the ultrahigh transparency (77.4%). Meanwhile, the tactile sensor could detect non-contact/contact touches by measuring the capacitance change.
Piezoelectric nanogenerator (PENG): Piezoelectric components can convert slight vibration or strain differences into electrical energy and then power downstream circuits. Nanostructured and flexible materials have been frequently used to fabricate PENG in wearable applications[71, 72]. For instance, He et al. fabricated an all-solid-state self-powered system using mesoporous films to generate piezoelectric effect as shown in Fig. 4(d). The self-powered system was charged by compressive deformation, and the overall capacity is 0.118 μA·h within 240 s. Such self-powered module based on PENG successfully powered up a smartwatch, sports wristband and array of LEDs, respectively, indicating its potential applications for self-sustainable wearable electronics[73].
In addition, the development of hybrid nanogenerators with high electrochemical stability has received much attention in recent years[3]. For instance, a self-arched nanogenerator (SANG) with a combination of triboelectric and piezoelectric effects is designed for real-time monitoring of pulse waveform by Li’s group[74]. This unique device structure and mixed effect of triboelectric and piezoelectric contribute effectively to SANG's stability and sensitivity. Moreover, Jiang et al. demonstrated a free-rotating hybrid nanogenerator constituted by a triboelectric and an electromagnetic generator. It can provide power for calculators, wireless temperature sensors and even charging mobile phones[15]. The integration of energy storage devices with hybrid nanogenerators significantly contributes to the development of bioelectronics with desirable self-power capability.
4.
Flexible energy storage module for sensing applications
A variety of flexible energy storage devices charged by different self-powered systems were reviewed, and they could be further integrated for sensing applications. In addition, wireless wearable bioelectronics has gained tremendous attraction due to its potential for non-invasive health monitoring[75]. The integration of the wireless data transmission module in wearable biosensing systems enables real-time analysis of target analytes levels and remote monitoring[76-78]. While it poses higher requirements for the power supply module in wearable electronics. This section will introduce the sensing applications for flexible energy storage devices, including physiological and physical signal detection. And Table 1 has summarized recent flexible energy storage devices integrated with sensing systems, and their superior performance is also involved.
Category | Material | Cycle | Capacitance/Capacity | Novelty | Biosensing application | Ref. |
Supercapacitor | Graphene-silver-3D foam | 25 000 | 38 mF/cm2 | Excellent cycling stability | pH sensor | [79] |
The sheath-core yarn | 10 000 | 761.2 mF/cm2 | Highly stretchable | Strain sensor | [80] | |
Nanosheets of CoSe2 on CNT | 4000 | 593.5 mF/cm2 | Superior mechanical stability | Opto-sensor | [81] | |
Textile | 10 000 | 644 mF/cm2 | Excellent flexible stability | Glucose sensor | [82] | |
Boron-carbon nanosheets | 10 000 | 534.5 F/cm3 | Large interlayer conductivity | Pulse sensor | [83] | |
Sweat as the electrolyte | 4000 | 10 mF/cm2 | Sustainable and safe | Sweat sensor | [40] | |
Lithium-air battery | 1000 | 680 mA·h/g | High energy density | Physiological sensor | [84] | |
Battery | Zinc-air battery | 6000 | 2.6 mA·h/cm2 | High safety and high force-resistance | Gesture sensor | [85] |
Zinc-MnO2 battery | 1000 | 277.5 mA·h/g | Highly compressible | Pressure sensor | [86] | |
Aqueous zinc-ion fiber | 5000 | 371 mA·h/g | High specific capacity | Strain sensor | [87] |
Table1.
Summary of recent flexible energy storage devices integrated with sensing systems.
Table options
-->
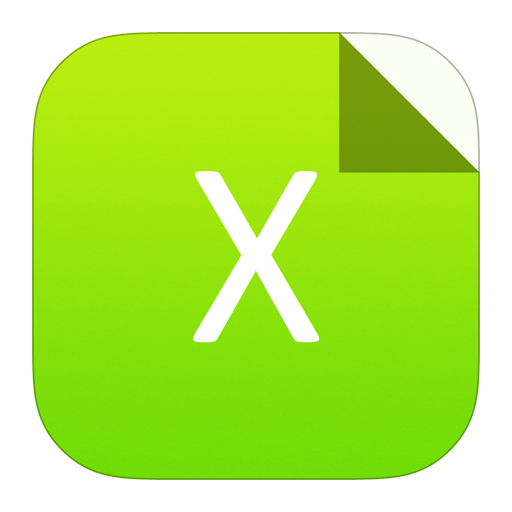
Download as CSV
Category | Material | Cycle | Capacitance/Capacity | Novelty | Biosensing application | Ref. |
Supercapacitor | Graphene-silver-3D foam | 25 000 | 38 mF/cm2 | Excellent cycling stability | pH sensor | [79] |
The sheath-core yarn | 10 000 | 761.2 mF/cm2 | Highly stretchable | Strain sensor | [80] | |
Nanosheets of CoSe2 on CNT | 4000 | 593.5 mF/cm2 | Superior mechanical stability | Opto-sensor | [81] | |
Textile | 10 000 | 644 mF/cm2 | Excellent flexible stability | Glucose sensor | [82] | |
Boron-carbon nanosheets | 10 000 | 534.5 F/cm3 | Large interlayer conductivity | Pulse sensor | [83] | |
Sweat as the electrolyte | 4000 | 10 mF/cm2 | Sustainable and safe | Sweat sensor | [40] | |
Lithium-air battery | 1000 | 680 mA·h/g | High energy density | Physiological sensor | [84] | |
Battery | Zinc-air battery | 6000 | 2.6 mA·h/cm2 | High safety and high force-resistance | Gesture sensor | [85] |
Zinc-MnO2 battery | 1000 | 277.5 mA·h/g | Highly compressible | Pressure sensor | [86] | |
Aqueous zinc-ion fiber | 5000 | 371 mA·h/g | High specific capacity | Strain sensor | [87] |
4.1
Physiological signal detection
Sodium sensors: The sodium ion is indispensable in our daily life, and it is essential for human healthcare. Typically, clinical sodium monitoring relies on instruments like inductively coupled plasma mass spectrometry. With the advancement of sensor manufacturing methods, sweat sodium can be detected with high sensitive ion sensors. Sodium sensors can be embedded in a flexible system and integrated with signal processing circuits. Fig. 5(a) shows a flexible sodium sensing patch that can be self-powered[78]. In this system, Dahiya’s group carried out a stable sodium measurement from perspiration on the skin. The perspiration also served as a biofuel for energy supply. The measured sensitivity of Na+ is 55.5 ± 0.3 mV per decade when the entire device is under continuous movement, which indicates a safe and sustainable perspective for wearable bioelectronics. Nevertheless, other power supply methods, such as human movement, are also fulfilled in practical applications[88, 89]. Since high energy consumption is a critical problem when biosensors are attached to the human body, efficient energy supply from human motion represents a fascinating approach to sustainably driving wearable bioelectronics.

class="figure_img" id="Figure5"/>
Download
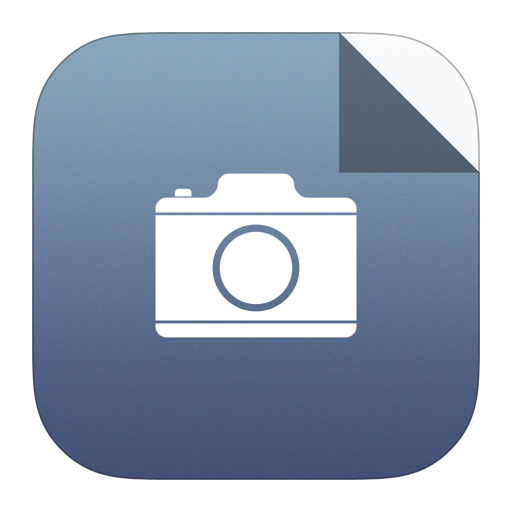
Larger image
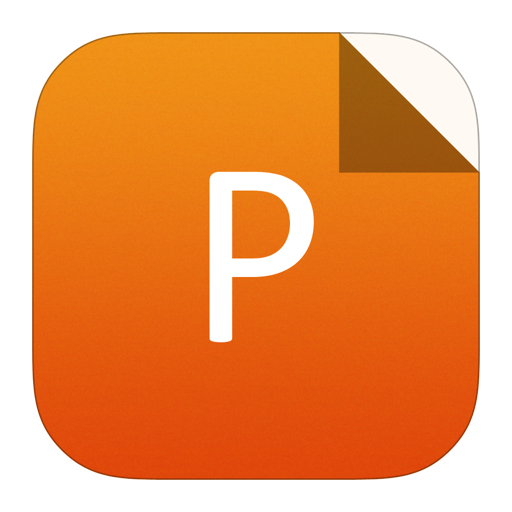
PowerPoint slide
Figure5.
(Color online) Physiological sensing systems integrated with flexible energy storage devices. (a) An all-in-one, and flexible self-powered sodium sensing patch with wireless data transmission[78]. (b) A self-powered smartwatch for non-invasive sweat glucose monitoring[92]. (c) Schematic of a self-powered system with pH sensor and its performance under dynamic bending conditions[79]. (d) A self-powered wristband that can power up LED as an indicator of gas detection[60].
Glucose sensors: Glucose levels in human fluids are significant health state indicator, especially for diabetes diagnostics. Therefore, wearable devices for glucose monitoring have long been a research focus. Interestingly, some materials can be simultaneously utilized for excellent glucose-sensing performance as well as energy devices[90, 91]. Ngo and coworkers adopted nickel oxide and graphitic carbon nitride hybrid nanostructure for electrode fabrication for both the glucose sensor and symmetrical supercapacitor. A high glucose sensitivity of 5.387 mA/(mM·cm2) was obtained, and an energy density of 1.06 kW/kg was also observed. However, it is relatively challenging to monitor the sweat glucose because it is less concentrated and other composites in sweat could affect the sensing accuracy. Fig. 5(b) illustrates a self-powered smartwatch for specifically non-invasive glucose monitoring[92]. In this work, Zhao et al. proposed a Zinc-MnO2 battery that served as a flexible energy storage device. It retained 91.86% of the initial capacitance after 1000 cycles of charging/discharging at 1.8 A/g while the self-powered system was charged up to 6.0 V within 1 h under bright sunlight. Apart from batteries, flexible supercapacitors can also provide energy for glucose sensors. Sun et al. reported that a flexible supercapacitor could power enzyme-free biosensors, and it also had a high sensitivity for glucose detection (592 μA/mM)[82]. However, long-term reliable glucose sensors remain a challenge for real-time biosensing applications.
pH sensors: As the pH values inside human body are relatively stable, pH sensors are more commonly adopted in sweat sensing. For instance, Manjakkal’s group invented a sweat-activated battery that simultaneously monitored heart rate, sweat chloride and sweat pH[79]. Also, a single pH sensor was proposed with a flexible graphene foam supercapacitor. Fig. 5(c) shows the 3D schematic of a self-powered system with a pH sensor and its performance under dynamic bending conditions. The self-charged supercapacitor exhibited a capacitance of 38 mF/cm2 and an energy density of 3.4 μW·h/cm2. The electrochemical and supercapacitive performance indicated its further applications, such as multi-sensing e-skin for human healthcare monitoring.
Gas sensors: A gas sensor can convert a certain gas volume fraction such as the composition and concentration into a corresponding electrical signal that can be further analyzed. Gas sensors are generally classified according to the detection of different chemicals, such as hydrogen, oxygen, ammonia gas, nitrogen dioxide, sulfur dioxide, etc.[93-96]. They have wide applications from personalized healthcare to environmental monitoring. A variety of strategies for scalable device manufacturing and facile system integration have shown great potential for wearable devices. Lin et al. fabricated a fully integrated sensing system powered by supercapacitors on plastic substrates[60]. Fig. 5(d) demonstrates that the as-fabricated self-powered wristband can power LEDs as an indicator of gas detection. In particular, the flexible supercapacitors provided an area capacitance of 12.9 mF/cm2, which could power the high-sensitivity tin oxide gas sensor at room temperature. Besides, micro-electromechanical sensing systems with significantly reduced device volume could enable a higher sensor array density. It also allows the integration with rechargeable and flexible energy devices to achieve reliable and repeatable device performance. Benedict et al. proposed a gas mapping system with a wireless communication module to track the global pollution levels by continuous and remote-controllable gas sensing[97]. It is believed with flexible energy devices, the sensing system will have practical applications for wearable biosensing and environment protection as well.
Humidity sensor: Humidity sensors have been investigated for industrial applications while attracting increasing research interest for biosensing. Various sensing materials are proposed for high-performance humidity sensing systems, including metal oxides, carbon materials, polymers, cellulose, etc.[98-102]. Pereira and coworkers fabricated a polymer humidity sensor and a printed battery made of lithium iron phosphate to form an all-printed smart label[103]. The printed humidity sensor had a linear response with a sensitivity of 0.004% relative humidity. However, the printed battery cannot support long-term power supply at this stage. It was recently reported that an all-solid-state flexible capacitor provided a higher capacitance to drive a humidity sensor[104]. This textile supercapacitor exhibited a specific capacitance of 8.01 F/g and a high cyclability of 5000 cycles. Without additional charging, it can drive commercial high-power sensors for 47 min.
4.2
Physical signal detection
Pulse sensors: Pulse sensors can detect the pressure change generated during arterial pulsation and convert it into an electrical signal that can be observed in a straightforward manner. Self-sustaining power packs have been demonstrated to power the pressure sensor and monitor human physiological signals[105]. In Xu’s work, photovaltaic devices and supercapacitors were integrated into an assembled power pack. It provided stable power output for pulse sensing regardless of the sunlight fluctuation, demonstrating its potential applications in future wearable electronics. In addition, Rajendran et al. proposed a screen-printed flexible solid-state supercapacitor for self-powered pulse sensing systems as shown in Fig. 6(a). The system demonstrated excellent mechanical stability with and serpentine independent interconnections[106]. The supercapacitor based on cured CNT electrodes showed an areal capacitance of 62 mF/cm2 at an applied current density of 0.19 mA/cm2, and only a decrement of 10% during stretching was observed. The pulse sensor was successfully powered even at low intensity in outdoor sports activities, proving its feasibility in personalized health monitoring. Yu and coworkers designed graphene-based electrodes for both supercapacitors and pressure sensors in a physiological signal sensing system[107]. Apart from supercapacitors, flexible batteries also have been demonstrated to power a wearable pulse sensor[108]. Li et al. reported a flexible solid-state zinc ion battery with desirable operational safety. It delivered a power density of 148.2 mW/cm2 and excellent cycle stability (maintained at 97% after 1000 cycles). It successfully replaced the commercial battery pack for a smartwatch and supported pulse sensing.

class="figure_img" id="Figure6"/>
Download
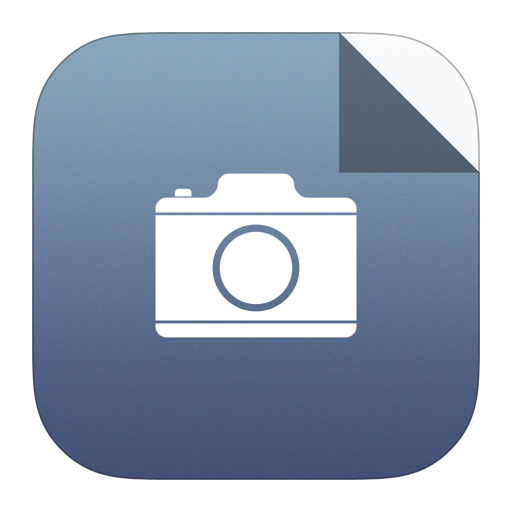
Larger image
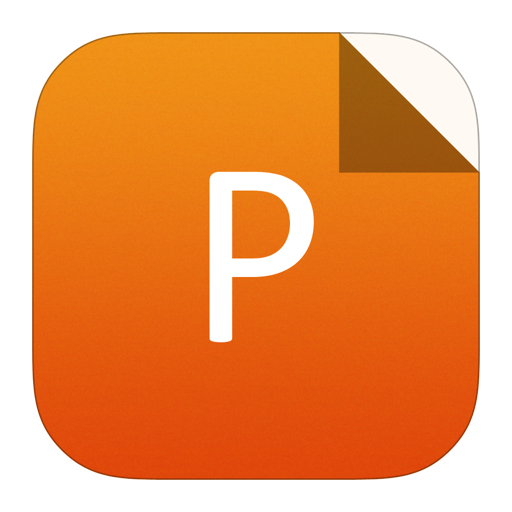
PowerPoint slide
Figure6.
(Color online) Physical sensing systems integrated with flexible energy storage devices. (a) A screen-printed flexible solid-state supercapacitor for self-powered pulse sensing[106]. (b) Schematic illustration of an integrated self-powered tactile sensor[114]. (c) The structure design of the FBG sensor for in-situ temperature measurement[117]. (d) Integration of the dual-mode strain sensor and supercapacitor on a deformable substrate[126].
Tactile sensors: With the development of microelectronic technology and the emergence of various organic materials, a variety of tactile sensors have been proposed for strain and pressure sensing. They play an increasingly important role in wearable devices for artificial intelligent body monitoring[109]. Typical substrates for tactile sensors fabrication include hydrogels, papers and textiles[110-112]. Dai’s group reported the fabrication of unique sheath-core yarn to construct devices with high energy storage capacity and a highly multifunctional sensing system[80]. The integrated electronic effectively monitored human body motions including different ranges of stress deformations. It exhibited an ultrahigh strain sensing range (0–350%) as well as an excellent capacitance of 761.2 mF/cm2 at the scanning rate of 1 mV/s. Shit et al. reported a self-powered capacitive sensor could be used on the skin to sense the human heartbeat, pulse and voice[113]. Besides, a physical stimulus that may cause a change in physical size or dielectric constant will introduce a measurable change in capacitance. Fig. 6(b) shows a self-powered flex sensor integrated with and its sensing responses to different bending angles proposed by Xu’s group[114]. The system used Mxene nanosheets to fabricate flexible supercapacitors. The sensing response current increased with the bending degrees. Such self-powered and wearable systems can be widely adopted in human-machine interfaces and biological monitoring.
Temperature sensors: According to the measurement method, temperature sensors can be divided into contact and non-contact sensing. While temperature sensors are now widely used in industrial and agricultural life, reports on the combination of flexible temperature sensors and energy storage devices for wearable applications are rare[115]. Hong's group reported a smart skin temperature patch that could fit the skin conformally and continuously monitor biological data for assessing physical condition[116]. This work shows the potential for smart patches to monitor non-febrile conditions in the community as well as non-invasively monitor individuals’ body temperature, even in real-life situations. In addition, Seema and coworkers designed a fiber Bragg grating (FBG) sensor for in-situ temperature measurement, integrated with flexible planar supercapacitors into a self-powered device[117]. Fig. 6(c) shows the structure design of the FBG sensor. The bending angle changes according to the measured temperature in the surrounding environment.
Opto-sensors: Photodetectors can detect the conductivity of the irradiated material changes due to radiation, and it is widely used in the range of visible spectrum, infrared spectrum and UV spectrum[118-120]. Applications and the powerful functions of fibrous photodetectors have been reported. Yildirim et al. reported a self-charging photodetector based on nanowires[121]. The nanowire can receive light energy and light information simultaneously. In other words, the nanowire serves as a nanogenerator to power itself and a photodetector to sense light signals. Recently, Zong’s group invented a high-performance flexible supercapacitor based on cobalt selenide (CoSe2) and CNT film[122]. It generated a stable output voltage of 1.8 V and a high energy density of 0.25 mW·h/cm2. In addition, a CoSe2/CNT-based photodetector was powered up, and fast response was observed under different wavelengths.
As discussed above, wearable sensors play an essential role in physiological and physical signal-detecting fields, and they can record continuous signals integrated with self-powered systems. Multifunctional sensing systems, including chemical and physical sensing, have also become a popular research area[123-125]. Park and coworkers fabricated an integrated system of physical sensors and a supercapacitor on a wearable substrate using liquid-metal interconnections. As Fig. 6(d) shows, dual-mode and a strain sensor are integrated with a flexible supercapacitor and attached to the hand’s skin[126]. This study used microporous polypyrrole-coated graphene foam as the single functional material for the fabrication of active multifunctional sensors. Overall, such multifunctional sensing systems with sustainable power supplies have made exciting progress. It provides promising strategies to design a self-powered sensing system with considerations on safety, stability and biological conformality to fulfill the requirements for health monitoring applications.
5.
Challenges and prospects
In recent years, rapid research advances have been achieved in flexible electrochemical energy storage, and many of them are adopted in commercially available products. The latest development on the integration of flexible energy storage devices into wearable bioelectronics is introduced in this review. The technology on material engineering and flexible device fabrication attract tremendous research interests, reflecting the urgent demand for flexible energy devices with desirable characteristics to power wearable sensing systems.
However, there still exist challenges on flexible energy storage devices for practical applications. Firstly, one of the critical issues for flexible devices lies in mechanical stability. While energy devices can be successfully constructed on various flexible platforms, most conductive current collectors and active materials for energy storage are intrinsically rigid. Therefore, mechanical interference such as bending, twisting and stretching could possibly introduce cracks within layers and device delamination. It will undoubtedly lead to poor device performance during charging and discharging and even raise safety concerns, such as organic electrolyte leakage. To tackle this challenge, innovation on flexible materials, structural device designs, and reliable approaches for back-end packaging are expected. Secondly, as the volume of the energy device keeps reducing to fulfill the requirements on device miniaturization, especially for wearable applications, its energy storage capacity decreases significantly. It could result in inadequate power supply during long-term operation. Especially for wearable biosensing applications that aim at real-time and long-term monitoring, its practical applications could be limited. In order to achieve the competitive energy capacity of flexible energy storage devices compared with their counterparts in rigid formats, several strategies have been proposed. For instance, the electrodes can be texturized with micro/nanostructures to increase charge storage and facilitate ion transfer to improve the energy density. Thirdly, for wearable biosensing applications, biocompatibility is one of the crucial considerations. The materials utilized for energy devices fabrication should be nontoxic and nonirritating to human skin. The flexible substrates and packing materials are also expected to provide attractive form factors such as air permeability and moisture conductivity, so as to improve the comfortability for wearing. In summary, it is believed that flexible energy storage would be developed and revolutionized with high mechanical and electrochemical stability, which is essential for their further integration with wearable bioelectronics for applications in personalized healthcare and robotics.
Acknowledgements
This work was supported by the Engineering Research Center of Integrated Circuits for Next-Generation Communications Grant (Y01796303) and Southern University of Science and Technology Grant (Y01796108, Y01796208).