1.
Introduction
The energy crisis caused by the massive consumption of fossil fuels is a serious problem that must be addressed today. Since the Second World War, many countries have committed to the development of renewable energy, including nuclear energy, wind energy, solar energy and tidal energy; of these, solar energy is favored, being both eco-friendly and economical. In the last decade, significant progress has been made in the field of perovskite solar cells (PSCs), with third-generation photovoltaic materials representing a promising candidate to replace monocrystalline silicon. Given perovskite’s power conversion efficiency (PCE), ranging from 5.8% to 25.5%[1-5], organic–inorganic halide PSCs have become the backbone of perovskite efficiency growth, due to their wide light absorption, excellent carrier mobility, and electron diffusion length. However, volatile cations, such as methylammonium (MA+) and formamidine (FA+), used in organic–inorganic halide solar cells are not stable under light, heat and temperature, making them particularly vulnerable to degradation due to environmental conditions, and as such, unmarketable[6, 7]. These cations degrade readily, and are therefore not suitable for commercial use[8]. In response to this, researchers have attempted to introduce inorganic cesium (Cs+) in place of organic cations, to form all-inorganic perovskites[9-12].
Of the various all-inorganic perovskites, CsPbI3 and CsPbI2Br have relatively narrow bandgaps, (Eg), together with a wide light absorption range, and have achieved efficiencies of about 19%[13-15]. However, when the external temperature changes, both are prone to thermodynamic phase transition into a non-perovskite phase, resulting in extremely low efficiency, which also restricts their development for commercial use. In contrast, CsPbBr3 shows excellent thermal stability under environmental stress[16-18]. Unfortunately, the large band gap (2.3 eV) of CsPbBr3 limits the absorption of light, restricting its further development. Taking into account the issues above, it is widely understood that CsPbBr2I has a more suitable band gap (2.08 eV) as compared with CsPbBr3 (2.3 eV), and demonstrates excellent thermal stability, as compared with CsPbI3 or CsPbI2Br. Since CsPbBr2I forms a high-temperature phase at high temperature, and enters a tetragonal phase when cooled to room temperature, it exhibits relatively superior thermal stability, meaning that CsPbBr2I does not undergo thermodynamic phase transition when the external temperature changes[19-21].
CsPbBr2I was first discussed by Ma et al. in 2016[22, 23]. With process optimization and ion doping, its PCE attained a maximum of 11.08%. However, the PCE of CsPbBr2I still lags far behind other types of perovskite (e.g., CsPbI3 or CsPbI2Br). The main reason for this is the serious phase segregation inside the CsPbBr2I. Under illumination, halide ions migrate to the grain boundary or crystal surface, so that the concentration of halide ions in the grain boundary or crystal surface is too high, which seriously affects ions migration, and leads to ions enrichment, thereby causing phase segregation. Phase segregation changes the absorption range of visible light by changing the band gap of the CsPbBr2I, resulting in lower efficiency. As such, the actual PCE of CsPbBr2I was far lower than the theoretical efficiency.
Phase segregation has always been the most worthwhile area of study in relation to hybrid halide PSCs. For example, I– and Br– in the crystal lattice of MAPb(BrxI1?x)3 and CsPb(I1?xBrx)3 migrate due to the halide ions obtaining sufficient migration energy under light[24, 25]. Due to the high energy of the grain boundary and crystal surface, I and Br ions will eventually agglomerate to form Br-rich and I-rich phases on the grain boundary and crystal surface. This segregation has a serious impact on the photoelectric properties of mixed halides. The consequences of phase segregation are generally considered based on two aspects: (1) It results in severe current density–voltage (J–V) hysteresis, and affects the performance of the device. (2) During the migration of halide ions under light, they will be adsorbed by high-energy grain boundaries and crystal surfaces, and agglomerate on the grain boundaries and crystal surfaces. As such, grain boundaries, grains and crystal surfaces have high energy, and play an important role in ion migration. The accumulation of ions at the grain boundaries will lead to the formation of I-rich and Br-rich phases resulting in phase segregation. However, the I-rich phase (tending to 1.55 eV) and the Br-rich phase (tending to 2.3 eV) produced by phase segregation deviate from the appropriate bandgap respectively, resulting in the red shift and blue shift of absorption, affecting absorption and reducing efficiency. This is a significant reason for the PCE of CsPbBr2I being lower than that of other inorganic PSCs. However, due to the limitations of microscopic observation, the mechanism of phase segregation cannot currently be fully confirmed [26, 27].
In this review, we first introduce the crystal structure and photoelectric properties of CsPbBr2I. Secondly, by analyzing the structure of CsPbBr2I, the reasons for its high thermal stability and phase transition are revealed. Next, we discuss possible strategies to suppress light-induced phase transition, such as reducing the numbers of grain boundaries by improving the spin-coating of the membrane, so as to prepare a large-grain membrane, reducing the grain boundary’s surface energy by modifying the interface, and changing the bonding length via ion doping. Finally, we discuss the problems in this field, and provide an outlook for the future of CsPbBr2I.
2.
The crystal/electronic structure and optical properties of CsPbBr2I
2.1
Crystal structure
Like other CsPbX3 (X = I–, Br–), the crystal structure of CsPbBr2I can be described as the Pb site, and X site ions form a [MX6]4– corner-shared octahedron; Cs+ is located in the center of the octahedron, as shown in Fig. 1(a)[28, 29]. Perovskite follows the Goldshmidt tolerance factor, t, as given in Eq. (1):

class="figure_img" id="Figure1"/>
Download
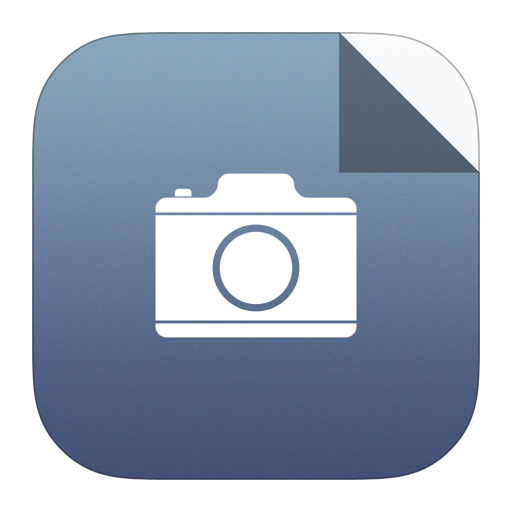
Larger image
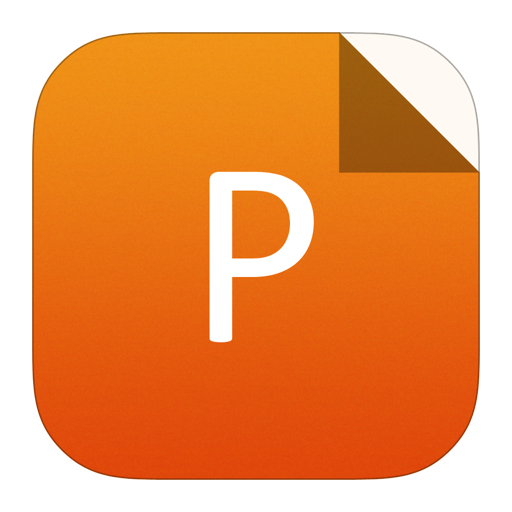
PowerPoint slide
Figure1.
(Color online) (a) Crystal structure of cubic CsPbX3 perovskite. Reproduced with permission[35]. Copyright 2019, Springier Publications. (b) The mechanism of bonding/antibonding orbitals of ABX3. Reproduced with permission[96]. Copyright 2016, American Chemical Society Publications. (c) The phase transition causes the angle of the M–X–M to decrease from 180°, leading to a change in the conduction band minimum and valence band maximum, thereby affecting the bandgap[30]. Copyright 2015, American Chemical Society Publications. (d) Absorbance spectra for inorganic perovskite films with different compositions of CsPb(I1–xBrx)3. Reproduced with permission[35]. Copyright 2018, Elsevier Inc Publications. (e) Absorption coefficient and steady state PL spectrum of CsPbBr2I. Reproduced with permission[22]. Copyright 2016, Wiley-VCH Publications.
$$t =frac{{r}_{mathrm{A}}+{r}_{mathrm{X}}}{sqrt{2}({r}_{mathrm{B}}+{r}_{mathrm{X}})},$$ ![]() | (1) |
where rA, rB, and rX are the ionic radii of their respective ions in the ABX3. With respect to CsPbX3, the halide anion has a smaller negative charge than the oxide anion, and the halide ion has a large radius. This requires a large cation ion radius, and low valence at the A site, so Cs+ is the appropriate choice for inorganic ions, and is sufficient to maintain the perovskite structure[30-33].
According to Eq. (1), when 0.8 < t < 1, the perovskite phase structure has a relatively high PCE. When t < 0.8, the efficiency of the resulting non-perovskite phase is very low. In theory, by introducing other ions to tune the t value to between 0.9 and 1, we could increase the stability of CsPbBr2I.
2.2
Electronic structure
The electronic structures of different components of CsPbX3 share great commonalities. The valence band maximum (VBM) of CsPbBr2I is primarily composed of anti-bonding hybrid Pb 6s, and X np (X = I?, Br?) orbitals, where the main contribution is X np. The conduction band minimum (CBM) is determined by the anti-bonding mixture of Pb 6p and X np orbitals, and the main contribution is Pb 6p, as shown in Fig. 1(b)[34]. In the ideal undistorted perovskite crystal structure, the axial orbital overlap between the halide p-orbital and the metal s- and p-orbitals is optimal, and this overlap causes the s-type valence band to be significantly widened, thereby significantly increasing the bandwidth and reducing the band gap. When the phase changes from α-, to β-, to γ-, the octahedral structure begins to tilt, and the axial orbital coincidence decreases, resulting in a decrease in the bandwidth, an increase in the bandgap, and an increase in the density-of-states (DOS) at the top of the valence band, as shown in Fig. 1(c)[30].
2.3
Optical properties
The optical properties of CsPbBr2I are described in terms of absorption and quantum yield (QY).
(1) Fig. 1(d) shows that as the iodine concentration increases, the wavelength becomes longer, and the absorption begins to shift[35, 36]. As Fig. 1(e) clearly shows, the absorption of the film starts at about 605 nm, corresponding to a bandgap of about 2.08 eV[37, 38].
(2) QY is a measure of the capacity of optoelectronic devices to absorb light-emitting electrons. For a QY of CsPbX3 between 10%–90%, Samanta et al. measured the fluorescence QY of CsPbBr3 and CsPbBr2I to be ~40% and ~10%. This indicates the presence of many inherent trap states in CsPbBr2I, which affect the low-emission intensity. The main trapping states result from iodine-induced distortion of the corner sharing [Pb(Br/I)6] octahedral of the cubic CsPb(Br/I)3 nanocrystals, leading to the formation of a twisted crystal structure[39].
3.
Phase transition of all-inorganic halide perovskite
Stability is a major challenge for all inorganic CsPb(I1?xBrx)3 (0 ≤ x ≤ 1); this includes both thermal stability and optical stability. The stability of CsPb(I1?xBrx)3 is dependent on the ratio of Br/I content. Fig. 2(a) shows that when x > 0.2, CsPb(I1?xBrx)3 has a stable orthorhombic perovskite structure at room temperature. When x < 0.2, the perovskite structure tends to transform to the delta phase at room temperature, i.e., a thermally-induced phase transition, including for CsPbI3[40]. This is due to the larger radius of I ions, which makes the chemical bond between I? and Cs+ longer, resulting in an unstable lattice with a tendency to transform into the delta phase. As such, Br, with its small radius, is an ideal ion. It matches the radius of Cs+, leading to the formation of a more stable crystal structure[41].

class="figure_img" id="Figure2"/>
Download
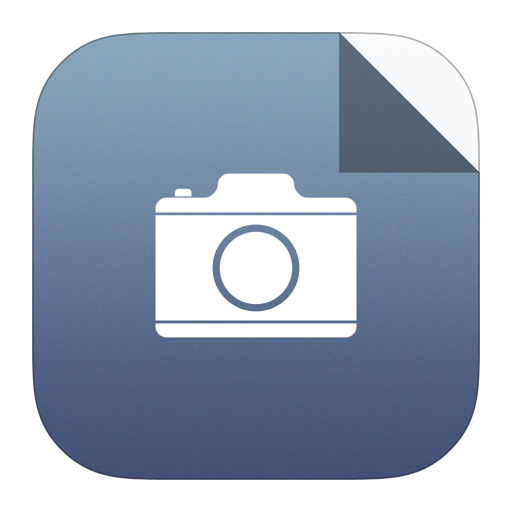
Larger image
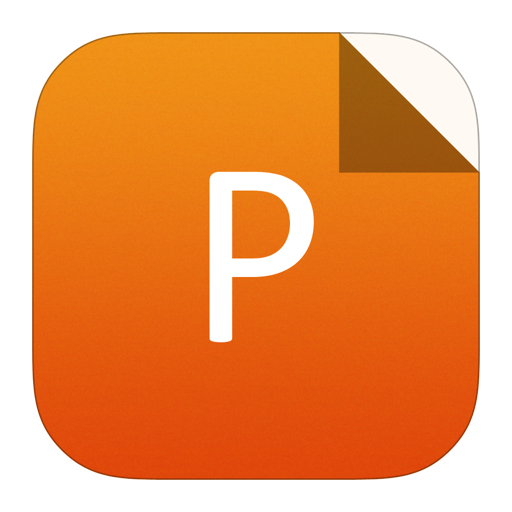
PowerPoint slide
Figure2.
(Color online) (a) Illustration depicting the perovskite crystal structure as a function of the iodine/bromine ratio. Reproduced with permission[40]. Copyright 2018, Wiley-VCH Publications. (b) The phase transition causes the angle of the M-X-M to decrease from 180° due to the influence of temperature. Reproduced with permission[31]. Copyright 2016, American Chemical Society Publications. (c) Photograph of the low-T phase (non-coloured) and high-T phase (orange-red-coloured) thin films. Reproduced with permission[45]. Copyright 2018, Nature Publications Group. (d) The low-T phase is represented by one-dimensional chains of edge-sharing lead-halide octahedra, whereas in the high-T phase, the octahedra share corners. Reproduced with permission[45]. Copyright 2018, Nature Publications Group.
However, with an increase in the Br/I content, the thermal stability of the material is generally overcome, while the optical stability of the material gradually weakens, i.e., light-induced phase segregation. When x > 0.2, phase segregation occurs under light, which specifically manifests as a large number of I ions gathering at grain boundary or grain surface to form a compound, resulting in lower cell efficiency. This is primarily because mixed halide anions cannot exist uniformly in the lattice, due to ionic radius and electronegativity problems. When the external light provides energy, the two kinds of ions tend to segregate[42].
Taking into account the above two issues, the Br/I ratio in CsPbBr2I determines its characteristic of strong thermal stability, but also causes phase segregation under illumination. In the following section, we will first analyze the thermal stability mechanism of CsPbBr2I, then discuss the internal causes of severe phase segregation in CsPbBr2I, and finally provide some strategies to prevent phase segregation.
3.1
Thermally induced phase transition
For the all-inorganic version, a substantial change in the crystal structure, namely phase transition, will occur at different temperatures. Phase transition is the transition between different crystal lattices and it is accompanied by the absorption or release of heat. In this part, we first discuss the mechanism of thermally induced phase transition, and then elaborate the phase transition of CsPbBr2I.
3.1.1
Mechanism of thermally induced phase transition
A phase transition is a change in the crystalline structure of the same substance at different temperatures. Each crystal structure at different temperetures is known as a phase. Since different crystal structures have different optical properties and cell parameters, perovskite materials have different light absorption rates at different temperatures, with the result that different efficiencies are produced under different phases[43, 44]. The root cause of perovskite phase transition is that the perovskite skeleton is a double bridge connecting halide ions (M–X–M moiety), as shown in Fig. 2(b)[30]. Under the influence of external temperature or pressure, the perovskite reduces the symmetry by changing the angle of M–X–M to adapt to the corresponding external conditions.
3.1.2
CsPbBr2I thermally-induced phase transition
The crystal structure of CsPbBr2I is mainly high-temperature (α-perovskite), inculding the space group Pm-3m, and the low-temperature non-perovskite phase (δ-perovskite), Pmnb[45]. The high-temperature phase has a deep orange color, and the low-temperature phase is nearly lucent, with a low PCE, as shown in Fig. 2(c). In the process of preparing CsPbBr2I, the halide is heated above the temperature at which the low-temperature phase changes to the high-temperature phase, resulting in the formation of a high-temperature phase.
In an inert environment, with a decrease in temperature, the high-temperature phase forms a metastable state, and remains at room temperature, thereby maintaining extremely high efficiency. As shown in Fig. 2(d), the transition from the high-temperature to the low-temperature phase is reversible. However, for CsPbI3, after about 320 °C heating and cooling, a high-temperature metastable phase forms at room temperature, and heating the metastable phase again causes it to transform extremely easily into a low-temperature non-perovskite phase. As the I– on the CsPb(I1?xBrx)3 (0 ≤ x ≤ 1) lattice is partially replaced with Br–, the bromine-rich crystal structure has a more suitable t, and is not prone to thermodynamic phase transition[46, 47]. For example, once the formation of CsPbBr2I forms a high-temperature metastable phase, heating within the melting point range does not readily transform into the non-perovskite phase.
3.2
Light-induced phase transition
Unlike monocrystalline silicon solar cells, CsPbBr2I halide perovskite is composed of different anions, and the migration of these anions requires relatively low energy. When there is ambient light to provide energy, ion migration will occur, and anions will migrate. In this section, we discuss the phase-segregation phenomenon of CsPbBr2I under light.
3.2.1
The origin of photo-induced phase segregation
Although newly-prepared CsPb(I1?xBrx)3 (0 < x < 1) halide is evenly mixed, with an increase in Br content, it will display a red-shifted photoluminescence peak, which splits into two smaller peaks with different absorption wavelengths under illumination, as shown in Fig. 3(a)[48, 49]. Under light, the CsPb(I1?xBrx)3 (0 < x < 1) mixed with halide changes from a regular arrangement to a segregation of I– and Br–, where the bandgap with a previously uniform halide mixture increases in the Br-rich region, and decreases in the I-rich region, respectively. In the presence of an I-rich phase, the carrier is captured and thermalized rapidly on encountering an I-rich region with low bandgap, when it is diffused by light. The I-rich phase and the homogeneous phase can also form a built-in electric field, which accelerates the sweep of carriers into the I-rich phase, leading to the formation of new defects at the grain boundary and crystal surface, which results in a decrease in fill factor, affecting the voltage and current density, and thus the overall PCE[50, 51]. This segregation exists in the grain boundary, and also in the crystal interior; however it is more prevalent at grain boundaries than inside the crystal, as shown in Fig. 3(b).

class="figure_img" id="Figure3"/>
Download
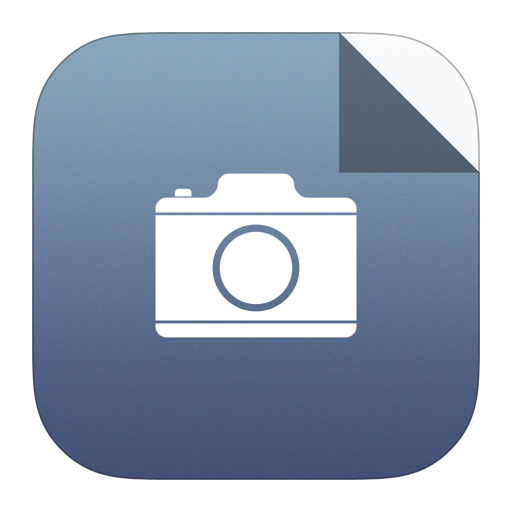
Larger image
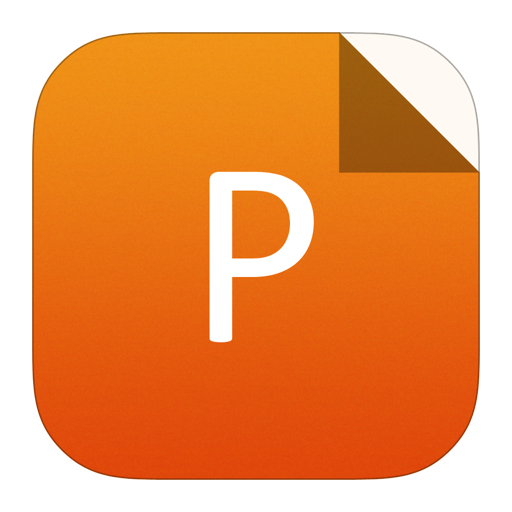
PowerPoint slide
Figure3.
(Color online) (a) The photoluminescence peak of these mixed-halide perovskites shifted exclusively to 1.87 eV after continuous illumination. The solid lines denote the spectra taken from freshly made samples, and the dashed lines dentote the measurements following 10 min illumination at an intensity of 0.3 W/cm2. Reproduced with permission[56]. Copyright 2019, Nature Publications Group. (b) Ion migration diagram of CsPb(I1–xBrx)3 in the grain interior and at the grain boundary under light. Reproduced with permission[53]. Copyright 2018, American Chemical Society Publications. (c) Secondary electron SEM image of CsPbBr2I film surface. (d) CL mapping of the CsPbBr2I film. Reproduced with permission[52]. Copyright 2017, Wiley-VCH Publications. (e) The calculated ΔGdark per volume (solid line) is negative, regardless of the Br content. (f) Under illumination, as the grain size exceeds X0, ΔGlight > 0. Reproduced with permission[56]. Copyright 2019, Nature Publications Group.
Like most CsPb(I1?xBrx)3 (0 < x < 1) perovskites, regardless of the CsPbBr2I spin-coating methods, there is a large difference between the forward sweep and the reverse sweep voltage, resulting in a very low average voltage. This is due to I– and Br– agglomeration at the grain boundary and crystal surface, respectively, under light, which causes phase segregation of CsPbBr2I, resulting in severe hysteresis and low efficiency. Li et al. first used scanning electron microscopy (SEM) and cathodoluminescence (CL) to conduct microscopic analyses of CsPbBr2I[52]. Figs. 3(c) and 3(d) show the microscopic grain morphology of CsPbBr2I under SEM and CL, respectively. The team also reported the phenomenon of "clusters" of halide I segregation at the grain boundaries and on the surface of the film[53].
3.2.2
The mechanism of photo-induced phase segregation
The study of hybrid halide perovskite ion migration has a history of more than 30 years. As for how the halide phase is segregated, although it still cannot be observed with specific equipment, some studies have found that ion migration triggers phase segregation[54, 55]. The segregation of halide ions is generally related to crystal defects, the bonding method between halides, and the formation energy required for ion migration. A great deal of research has been devoted to an understanding of the basic electronic processes of the halide hybrid system, establishing thermodynamic models, and simulating the occurrence of this phenomenon by means of theoretical calculations. Here, we introduce two typical theoretical explanations of phase separation:
(1) Nucleation theory. In this model, whether the phases of the mixed halide are uniform or segregated depends on the Gibbs free energy, ΔG. When ΔG < 0, the phase of the CsPbBr2I mixed halide is stable. When ΔG > 0, the mixed halide will be unstable inside the lattice, creating a driving force that segregates the halide components, and the I– and Br– inside the lattice will tend to separate. The Gibbs free energy in the dark (ΔGdark) is a function of the average grain size, r, the temperature, T, and the relative content of bromine in the mixed halide phase, XBr, as shown in Eq. (2)[56]:
$$begin{array}{l}Delta {G_{{ m{dark}}}}left( {{X_{{ m{Br}}}},T} ight) = dfrac{4}{3}pi {r^3}left[ {Delta {h_{{ m{mix}}}}left( {{X_{{ m{Br}},}}T} ight) - T cdot Delta {s_{{ m{mix}}}}left( {{X_{{ m{Br}},}}T} ight)} ight] qquadqquadqquadquad-mathop sum _i {c_i}{W_i}{r^2}.end{array}$$ ![]() | (2) |
In the above equation, Δhmix is the volumetric enthalpy, T·Δsmix is the volumetric entropy, and ΣiciWir2 denotes the cohesive energies.
Using density functional theory (DFT) calculations, we find that, regardless of the size of the crystal grains, the ΔG is negative for any content of XBr in the dark, which proves that the phase of the mixed halide is stable under dark conditions, as shown in Fig. 3(e). While light-induced polarons are introduced under illumination, polarons can generate lattice strain energy by lengthening or shortening the chemical bond between the Pb-site and the halogens, and the Gibbs free energy (ΔGlight) of the mixed phase under illumination depends on the lattice strain energy (Δgs > 0) originating from the light-induced polaron and ΔGdark. Because Δgs > 0, according to Eq. (3), when the grain size, r, is large enough, the ΔGlight will be greater than zero under light, meaning that a driving force of phase separation will be generated, so that the mixed halide will exhibit phase separation under light. Br–- and I–-rich domains are formed by dispersing and mixing the halide phase, as shown in Fig. 3(f):
$$ Delta {G_{{ m{light}}}}left( {{X_{{ m{Br}}}},T} ight) = Delta {G_{{ m{dark}}}}left( {{X_{{ m{Br}}}},T} ight) + frac{4}{3}pi {r^3} cdot Delta {g_{ m{s}}}left( {{X_{{ m{Br}}}}} ight).$$ ![]() | (3) |
However, the assumption above assumes that the temperature in the dark is the same as the temperature under light. In fact, the presence of light will inevitably cause the temperature to rise.
(2) Alloy lattice distortion theory. The serious segregation of mixed halides can be explained in the context of two mixed halide anions, which can be regarded as Al–xBx alloys[57]. The two alloy components are inherently less stable, due to compatibility issues, and the ions exhibit lattice distortion due to their different radii. Crystal defects, together with the instability of the composition, make it easier for the relatively small ion-radius I– to migrate under light excitation; compared to the interior of the crystal, the crystal surface and grain boundary have higher energy, due to lattice mismatch, and the grain boundary will adsorb a lot. The halide ions gather around the grain boundaries to form "clusters," in a process referred to as phase segregation. Phase segregation and ion migration primarily affect the hysteresis of current density and voltage. We can use the hysteresis index (HI) to describe the hysteresis of CsPb(I1?xBrx)3 (0 < x < 1). The specific formulas are as given in Eq. (4):
$$mathrm{Hysteresis; index}left(mathrm{HI} ight)mathrm{ }=frac{{mathrm{PCE}}_{mathrm{reverse}}-{mathrm{PCE}}_{mathrm{forward}}}{{mathrm{PCE}}_{mathrm{reverse}}}.$$ ![]() | (4) |
Li et al. pointed out that the hysteresis index of CsPbBr2I could be as high as 43.8%, far higher than for CsPbI3 or CsPbI2Br. In order to reduce the hysteresis of CsPbBr2I, and improve the efficiency of devices in which it is utilized, in this work, we focus primarily on methods of suppressing phase segregation, thereby reducing the hysteresis.
4.
Suppressed phase segregation of CsPbBr2I
In summary, the light stability of halide materials is the main obstacle to the development of CsPb(I1?xBrx)3 (0 < x < 1) PSCs, represented here by CsPbBr2I. Based on studies of the phase-segregation mechanism, we believe that phase segregation must be suppressed at the grain boundary, the film surface, and the lattice interior.
(1) For grain boundaries, a film with large grains and few grain boundaries can be prepared by improving the preparation method.
(2) For the surface of the film, serial passivation can be used to reduce the surface energy and suppress ions’ bias polymerization.
(3) For the inside of the grain boundary, ion doping can be used to enhance bonding, which effectively inhibits the ion migration caused by lattice expansion due to photo-generated carriers.
These three strategies for phase segregation suppression are described in detail below.
4.1
Preparation of large grain film
The defects on the perovskite film and numerous grain boundaries possess high energy; when halide ions migrate under light, these defects will adsorb halide ions, causing halide ions to agglomerate, and phase segregation to occur. As such, the preparation of perovskite films with large grains, no pinholes, and no cracks is key to suppressing phase segregation and obtaining high PCE for perovskite devices[58, 59]. In order to prepare a more ideal perovskite film, researchers generally optimize the film’s morphology using one of two approaches:
(1) The continuous exploration and improvement of film preparation methods has led to developments in spin-coating-based methods, and includes other film preparation methods such as the doctor blade, inkjet-printing, and slot-die coating for film preparation[60].
(2) Optimization of the technology under different preparation methods, such as annealing temperature and time, the composition of the solvent, and the modification of some intermediates.
Combining the two approaches above, we have improved the traditional film preparation methods and preparation processes, and developed new CsPbBr2I spin-coating methods, which have continuously improved solar cell efficiency. In the next section, we investigate traditional spin-coating methods, and examine the improved processes for each type of film[61].
4.1.1
Dual source thermal evaporation
The first CsPbBr2I film was first prepared using the dual source thermal evaporation method; as shown in Fig. 4(a), its efficiency reached 4.7%. This method can be used for large-scale production and coating in industry. The process involves loading CsI and PbBr2 into the device with a 1 : 1 ratio, heating of the two substances to a predetermined temperature, and evaporating to the preheated TiO2 substrate to prepare the CsPbIBr2 sample.

class="figure_img" id="Figure4"/>
Download
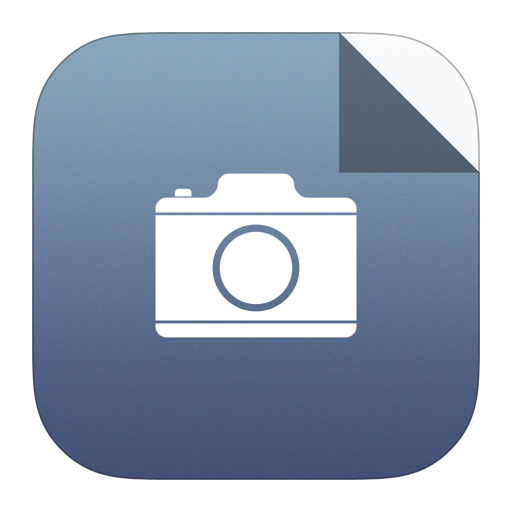
Larger image
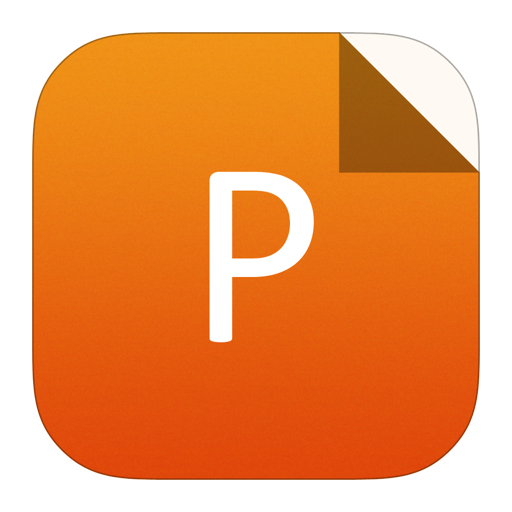
PowerPoint slide
Figure4.
(Color online) (a) Schematic diagram of dual-source thermal evaporation. Reproduced with permission[97]. Copyright 2018, Wiley-VCH Publications. (b) Illustration of CsPbBr2I perovskite film, fabricated using a preheating-assisted spin-coating process. Reproduced with permission[62]. Copyright 2019, The Royal Society of Chemistry. (c) Illustration of intermolecular exchange strategy. Reproduced with permission[59]. Copyright 2018, Wiley-VCH Publications. (d) Schematic illustration of conventional pathway growth (CPG), and seed-assisted growth (SAG) methods. Reproduced with permission[63]. Copyright 2020, Wiley-VCH Publications. (e) Illustration of CsPbBr2I film with spray-assisted deposition. Reproduced with permission[65]. Copyright 2016, American Chemical Society Publications.
Since the entire spin-coating process is carried out in steam, there is no residual liquid on the substrate, and the thickness of the film can be controlled by controlling the spraying rate[22]. However, the main issue of dual-source thermal evaporation is that CsI and PbBr2 will sublime on the TiO2 substrate at high temperatures, resulting in an uneven film. Furthermore, the annealing temperature and the substrate temperature will also affect the quality of the film. Therefore, the production of high-efficiency CsPbBr2I films is not possible using this method.
4.1.2
One-step method
The traditional one-step method is to dissolve CsI and PbBr2 dimethyl sulfoxide (DMSO) in a 1 : 1 ratio, spin-coating them onto a TiO2/SnO2 substrate, and annealing to obtain a CsPbIBr2 film. Although the one-step method is extremely easy to operate, it has many shortcomings, such as poor solubility of Br– in the solution, resulting in uneven film composition, lattice defects, and a reduction in the quality of the film. In addition, DMSO combines with PbBr2, forming a complex PbBr2-DMSO intermediate, which prevents the crystallization of CsPbBr2I film, and results in isolated holes on the film[58].
In order to overcome these problems, Que et al. preheated the substrate prior to spin-coating, which resulted in improved film coverage, as shown in Fig. 4(b), where the resulting grain size is 0.2–2 μm. However, the preheating temperature of the substrate is not easy to control. If the preheating temperature is too high, disorder in the [Cs-PbBr2]+ arrays in the initial formation will cause frost on the film’s surface, and have an impact on overall device performance. Zhu et al. used the method of intermolecular exchange to prepare their CsPbBr2I film, as shown in Fig. 4(c). The method involves a precursor CsPbBr2I film, prepared according to the one-step method, which is then coated onto the TiO2 substrate. For the intermolecular exchange, a methanol solution containing CsI is spin-coated onto the CsPbBr2I precursor film, then washed with anhydrous IPA (isopropanol). After annealing, the resulting CsPbBr2I film exhibited long-term stability and a greatly improved efficiency as compared to the traditional one-step method[62].
Whether employing a one-step method, preheating assisted deposition, or intermolecular exchange, since the formation of CsPbBr2I crystals requires high formation energy, high-temperature annealing can result in the growth of large grains, which increases production costs, and limits its application in flexible devices. In order to reduce the formation energy of CsPbBr2I crystals to lower the annealing temperature, Zhang et al. treated their CsPbBr2I film with methylammonium halides (MAX, X = I, Br) prior to annealing, so that the MA+ was at the center of the CsPbBr2I crystal nucleation, thereby greatly reducing its formation energy, meaning that the final annealing temperature could be reduced to 150 °C[63]. As shown in Fig. 4(d), compared with a CsPbBr2I film synthesized by the conventional route, the color of the film after MAX treatment becomes noticeably darker, and its absorbance is improved.
4.1.3
Two steps method
Given that, in the one-step method, the solubility of Br– in the precursor solution is relatively small, and ion doping is difficult, a two-step method has been proposed to resolve this difficulty. This primarily consists of dissolving PbBr2 in N, N-dimethylformamide (DMF) and DMSO as a precursor solution, and spinning it on the substrate, followed by drying on the heating stage for a period of time. The prepared film is then immersed in a methanol solution of CsI for a period of time, before being annealed to obtain a CsPbBr2I film with an efficiency of 8.25%[64]. However, the disadvantage of this method is that it results in the presence of residual methanol solution on the substrate, which affects the crystallization of the film. In order to further improve efficiency and film production speed, Lau et al. initially spin-coated a precursor solution of PbBr2 onto a mesoporous TiO2 substrate. The difference from the two-step method is that the methanol CsI solution forms steam at high temperatures, which is then sprayed onto the precursor film, and annealing for a period of time causes the film to crystallize in air, as shown in Fig. 4(e)[65]. For better control of the annealing temperature and in order to grow large-grain films, Wang et al. developed a two-step temperature-control annealing process: having prepared their CsPbBr2I film via the two-step method, the substrates were first placed onto a hotplate at a temperature of 150 °C for about 10 min. The substrates were then placed onto the hotplate at a temperature of 280 °C for 10 min. Using this method, the highest PCE achieved was 8.31%, as compared with the one-step PCE of 4.98%, an efficiency increase of 66.9%[66].
Having discussed the basic spin coating method and its improvements above, we find that each method has certain drawbacks, and that some process parameters are not ideal, such as the annealing temperature, substrate temperature, annealing time, spray rate, etc. All in all, making perovskite films with few defects and large grains helps reduce hysteresis and improve efficiency.
4.2
Passivation of film surface
Halide phase segregation will generate a large number of mobile ions near the grain boundaries or inside the crystal grains of the CsPbBr2I film. These mobile ions accumulate near the CsPbBr2I/ETL interface to form an incident electron injection barrier, which is not conducive to small extraction[60, 67, 68]. Therefore, the selection of appropriate charge transfer materials or materials to modify the CsPbBr2I/ETL interface could effectively reduce hysteresis and improve efficiency. At the same time, the choice of modifier to modify the interface may result in a better match in the band between CsPbBr2I and ETL, further reducing the energy loss[69]. With respect to charge transport materials, TiO2, SnO2, In2S3, etc., are generally selected[70].
(1) TiO2 is the most widely-used charge transport material, but its disadvantages include higher sintering temperature, narrow band gap, and relatively low electron mobility[71]. In order to improve the charge extraction rate of the CsPbBr2I/ETL interface, thereby improving efficiency, Hayase et al. used Xanthate to coat the surface of the CsPbBr2I; this then decomposed into S2– following high-temperature annealing, as shown in Fig. 5(a). Since S2– has a strong chemical bond with perovskite, which interacts with the perovskite phase, the perovskite film surface is passivated and stablised for a long time[72]. Zhu et al. used CsBr clusters to modify the TiO2 interface. Their study found that CsBr changed the CBM of TiO2, increasing it from –4.00 to –3.81 eV, while at the same time reducing the TiO2 power function from 4.11 to 3.86 eV[73]; Fig. 5(b) shows the band alignment diagram following this modification. CsBr clusters can also play a role in nucleation, promoting interface nucleation to improve efficiency. Liu et al. used SmBr3 to suppress phase separation[74]. The mechanism here is that Sm and TiO2, in combination with SmBr3, form Ti–O–Sm, thereby enhancing the interface. Moreover, Sm can replace Pb to permit gradient band doping, as shown in Fig. 5(c). The results show a reduction in HI, from 15.1% to 6.6%, while efficiency remained stable at about 10%.

class="figure_img" id="Figure5"/>
Download
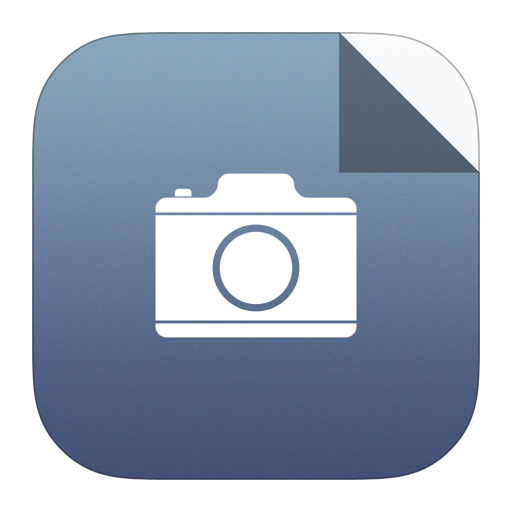
Larger image
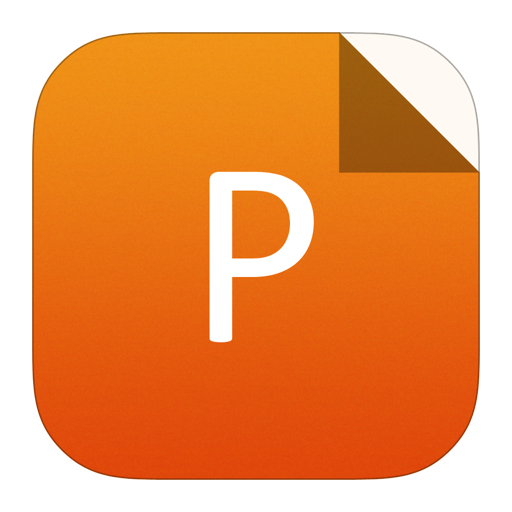
PowerPoint slide
Figure5.
(Color online) (a) Illustration of CsXth decomposition, and sulfur doping in perovskite. Reproduced with permission[72]. Copyright 2019, Elsevier Inc Publications. (b) Schematic architecture of the cell, together with the corresponding energy band diagrams for TiO2 and TiO2/CsBr ETLs. Reproduced with permission[73]. Copyright 2019, Wiley-VCH Publications. (c) SmBr3 doping normalizes the gap between CsPbBr2I and TiO2. Reproduced with permission[74]. Copyright 2019, Wiley-VCH Publications.
(2) CsPbBr2I film produced with SnO2 as the transport layer has high crystallinity and extremely uniform crystal grains, but the opening voltage is relatively low, while TiO2 is conducive to the separation and extraction of charges[75]. Exploiting the advantages of both methods, Cao et al. found that depositing a TiO2 atomic layer onto SnO2 can significantly improve the PCE of carbon-based all-inorganic perovskites. A TiO2 layer on an SnO2 electron transport layer simultaneously boosts the crystallization and increases grain size. The charge extraction and transportation are also effectively improved[76].
(3) In addition to the above two methods, ZnO and In2S3 can also be used as ETL transport layers for CsPbBr2I[77]. For both are stable and non-toxic to In2S3, and both possess a wide energy band, with greater carrier recombination than TiO2[78]. Yang et al. found that the efficiency of In2S3 is significantly greater than that of TiO2 under the same conditions, making it an ideal transport material[79].
4.3
Doping increases bond strength
Doping could introduce other ions into the crystal lattice, altering the crystal structure to suppress phase segregation and improve the performance of the material[80–82]. Doping has three main effects in terms of suppressing phase segregation.
(1) Increasing bonding strength. Since light can cause the crystal lattice of CsPbBr2I to stretch which in turn causes phase segregation, we can introduce ions with strong bonding close to the Cs+ and Pb2+ sites to partially replace the A site or the B site, thereby altering the bonding strength and distance of A–X and B–X, as shown in Fig. 6(a). If the bonding between A–X and B–X is enhanced, the photo striction can be reduced, thereby suppressing phase segregation[83].

class="figure_img" id="Figure6"/>
Download
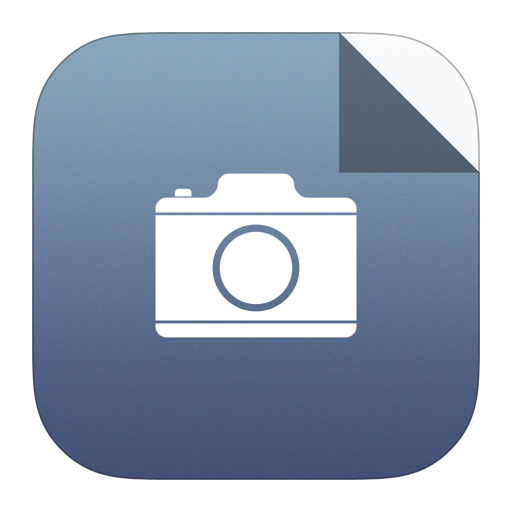
Larger image
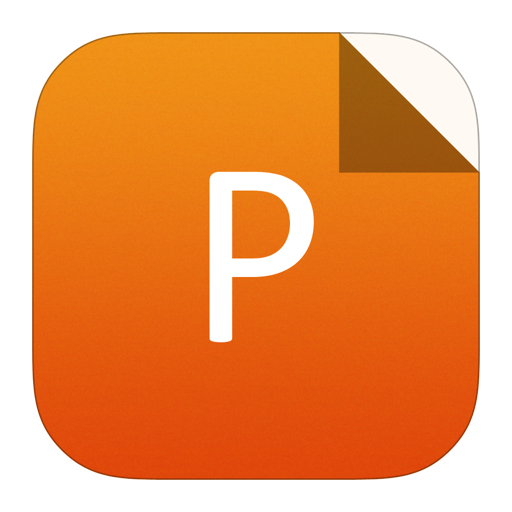
PowerPoint slide
Figure6.
(Color online) (a) Relation of the tolerance factor (τ) with a B–X–B bond angle (θ) of the ABX3 perovskite structure. Reproduced with permission[88]. Copyright 2018, American Chemical Society Publications. (b) Rotational distortion of [BX6]4?([PbX6]4?) octahedra can be restricted by reducing the B–X bond length when Pb2+ is partially substituted with smaller B-site cations. Smaller B-site cations reduce the size of the [BX6]4? octahedron, which in turn decreases the size of the cuboctahedral void for the A-site cation. Reproduced with permission[88]. Copyright 2018, American Chemical Society Publications. (c) Typical SEM images of CsPbBr2I, and CsPb0.995Mn0.005Br1.99I1.01 films, respectively. Reproduced with permission[91]. Copyright 2018, Wiley-VCH Publications. (d) SEM images of (above) CsPbBr2I and (below) CsPb(Ba)Br2I. Reproduced with permission[93]. Copyright 2017, Elsevier Inc Publications.
(2) Improving the crystallinity of the material. Pinhole defects in CsPbBr2I films are the main sites of ion migration induced by phase segregation[84, 85]. Doping can participate in the crystallization process, increase the crystallinity of the film, and shorten the crystallization time, thereby resulting in a more regular film, in order to suppress phase segregation.
(3) Changing the optical and electronic performance. Because phase separation occurs under light, doping can change the band gap by changing the CBM and VBM of CsPbBr2I, thereby affecting the light absorption of CsPbBr2I, which essentially inhibits phase separation.
By summarizing the effects of doping, we can then analyze the effect of doping on CsPbBr2I performance from A site and B site, respectively.
4.3.1
A-site doping
In CsPbBr2I, the Cs+ at the A site determines the space size of the corner-sharing [PbBr6]4- octahedra, and the introduction of ions with an A radius greater than Cs, such as (FA or MA), or ions less than Cs+, can inhibit phase segregation. Its main effect is to reduce lattice distortion caused by excited carriers through the substitution of other A-site ions, which promotes the thermodynamic stability of the uniform phase, and inhibits segregation[86]. Tan et al. incorporated Li into the A site of CsPbBr2I; compared with non-doped devices, the efficiency was increased by 1.84%[87]. This is because the introduction of Li+ into the crystal lattice of CsPbBr2I resulted in an increase in bond strength between the Cs, Pb, and halides, which helped to inhibit phase separation, and in addition, the average grain size of the crystals obtained via Li+ doping was significantly larger than that of undoped crystals.
4.3.2
B-site doping
With regard to B-site doping, the optical characteristics are mainly affected by CBM and VBM. Therefore, a partially-doped metal site (B-site cation) is a more direct and effective way to change the effect of photo striction on light illumination, and may eliminate the phase segregation problem[88]. The choice of B-site dopant and its main mechanism is illustrated in Fig. 6(b). In addition, compared with undoped CsPbBr2I, doped CsPbBr2I film has a better coverage.
Given that the energy level of B-site Pb is relatively deep, B-site doping with other metal ions may cause new deep defects, which will serve as scattering centers to further affect the original photoelectric properties of CsPbBr2I[78]. Therefore, it is very important to choose appropriate elements to replace Pb. Below are three different types of B-site doping:
(1) Among other kinds of perovskite doping, Mn2+ doping has proved to be an effective method to adjust the electronic and optical properties of semiconductor nanocrystals[89, 90]. As such, replacing Pb2+ with part of Mn2+ is considered to generally improve the efficiency of CsPbBr2I. Liang et al. synthesized a new Mn-doped all-inorganic perovskite, CsPb1?xMnxBr2?2xI1+2x. When the Mn2+ doping concentration x = 0.005, Compared with the CsPbBr2I film without Mn2+, the CsPb0.995Mn0.005Br1.99 I1.01 film displays vertical branches on its surface[91]. These branches expand the contact area between the perovskite and the electrode, and promotes holes from the perovskite layer, transferred to the electrode, as shown in Fig. 6(c).
(2) Sn doping in organic–inorganic lead-halide perovskites can narrow the bandgap and achieve high cell efficiency. Li et al. used the one-step antisolvent method to prepare CsPb1?xSnxBr2I (x = 0.00, 0.25, 0.5, 0.75, and 1.00) films with adjustable Eg between 2.04 and 1.64 eV[92]. In contrast to the above synthesis method, Liang et al. successfully prepared a new hybrid Pb/Sn inorganic perovskite CsPb0.9Sn0.1Br2I via a simple liquid phase process, whereby the doping band of Sn2+ adjusted the bandgap to 1.79 eV, and achieved an efficiency of 11.33%[64].
(3) Ba2+ doping results in a CsPbBr2I film with a good crystalline morphology. Liu et al. partially replaced Pb in CsPbBr2I with Ba (II, IR = 135 pm), which resulted in an undesirable t of less than 0.8. Ba(II) doping improves the device efficiency to 10.51%, as compared with an efficiency of 8.4% for a film not doped with Ba2+[93]. Due to the participation of Ba2+ doping, in the crystallization process, the crystallinity of the film is increased, resulting in many small and grains, as shown in Fig. 6(d).
Doping has achieved good results in terms of suppressing phase segregation. However, there are a series of scientific problems still to be resolved regarding doping. Issues such as whether dopants should be introduced into the crystal lattice, or just isolated on the surface, and how doping ions adjust the properties of the perovskite remain a mystery. In addition, the uniformity of the doped phase needs to be investigated by means of a high-resolution and high-sensitivity characterization method.
5.
Challenges and prospects
To summarize this article, we find that the biggest advantage of CsPbBr2I is that it will not fail due to thermodynamic phase change during external temperature change; i.e., it exhibits thermal stability. Its biggest shortcoming is that phase segregation can occur on exposure to light, causing hysteresis, which seriously affects efficiency. From the perspective of film preparation and interface modification, the efficiency of CsPbBr2I still shows room for improvement. In addition, the process of transferring CsPbBr2I from laboratory to commercial use represents a significant challenge. Below, we provide a brief summary of the challenges we face in the further development of CsPbBr2I, and look forward to its application prospects.
5.1
Challenges
Anti-hygroscopic. Studies have shown that unencapsulated CsPbBr2I can maintain its initial performance for up to seven days at 100 °C. CsPbBr2I has excellent thermal stability, but like other halide perovskites, CsPbBr2I is particularly hygroscopic. Although it can operate normally in an environment with normal humidity, when humidity increases, water molecules will induce a non-perovskite phase in the CsPbBr2I, resulting in device failure. Currently, the main protection measures to ensure moisture resistance in perovskites consist of mineral oil with hydrophobic and sealed packaging. However, organic mineral oil is an environmental pollutant, and is not convenient to use; we therefore need to conduct in-depth research into battery packaging materials.
Light stability. The critical issue to be addressed for CsPbBr2I is the phenomenon of light-induced segregation. Three approaches have been proposed above. It is commonly accepted that structure determines performance, given that the theoretical analysis of photo-induced segregation is based only on computer simulation calculations, and the effect of light-induced polaron on the lattice is only assumed. Therefore, the mechanism of phase segregation must be studied energetically in order to positively inhibit it.
Low PCE. Although the PCE of CsPbBr2I can be as high as 11%, compared with other Cs-based all-inorganic perovskite, its PCE is not very high. Although the bandgap determines the limited optical absorption range of CsPbBr2I, maximizing its efficiency within the limited optical absorption range is an issue requiring to be addressed. At present, there is not much room for film preparation and energy band alignment, and the main focus of future research should be to introduce interface passivators or ion doping to improve efficiency.
5.2
Outlook
Third-generation perovskite photovoltaic devices are primarily being developed with an eye to energy conservation and environmental protection. In the current experimental development stage, the selection of materials used to make devices is slowly shifting towards low cost and environmental protection considerations.
Development of inexpensive electrodes. Precious metal electrodes such as Au, Ag, etc. were previously selected for use in electrodes, while for the band connection between these metal electrodes and the perovskite layer, Spiro-MeOTAD, PCBM, etc. were used. On the one hand, these materials have poor stability, and on the other hand, these materials are extremely expensive to use, and cannot be converted for commercial use. In this regard, the replacement of the above-mentioned precious metal electrodes with carbon electrodes and hole-free transport materials is imperative in the long run. However, although current perovskite batteries made with carbon electrodes exhibit stable efficiency, they are generally relatively rare. It is to be expected that in the next few years, the number of perovskite batteries utilizing carbon electrodes will increase significantly.
Study nontoxic perovskites. Since the use of toxic Pb metal in perovskite materials causes serious environmental pollution after degradation, it is necessary to develop a pollution-free alternative to the use of lead. Several less toxic or non-toxic metals (Bi, Sn, Mn, Sb and Ge) represent possible substitutes for Pb. For the purpose of future research into the replacement of lead, in theory, attention should be paid to the following two points: (1) For any candidate material to replace lead, the ionic radius should follow an ABX3 formation of t (0.8 < t < 1); if the alternate radius is too large or too small, this will lead to t deviating from the ideal range, and produce poor results. (2) Pb is the main contributor to CBM and VBM, which decides the light absorption performance of perovskite materials; as such, replacement metal ions should share the same basic electronic structure as Pb. Sn is considered to be the most likely alternative to Pb metal, but the instability of Sn is also a problem worthy of our consideration. There is still a long way to go in the study of lead-free perovskites.
Explore the recyclability of ETL. TiO2 transmission materials are widely used for ETL materials, due to their stability and efficiency. Zhu et al. recycled their TiO2 glass substrate after use, then soaked and dried it in DMF for recycling[94]. In the future, we may seek out more economical and pollution-free ETL materials, or continue to strengthen the exploration of recovery processes for recoverable TiO2 substrates. This area shows great promise.
Improve the production efficiency of film. For large-scale production, the battery area we currently make is generally within 1 cm2. In order to make perovskite cover a larger area, the spin-coating process must be changed to facilitate mass production. For example, Wang et al. loaded their perovskite spin-coating liquid into a syringe, with the syringe needle switched for a brush in order to coat the perovskite, which greatly improves coating efficiency[95]. This is also the future direction of coating development.
Use in the glass coloration industry. In this review, CsPbBr2I maximizes its efficiency in the absorption range by suppressing photo-induced phase segregation. However, the inherent 2.08 eV band gap makes its theoretical maximum efficiency lower than that of most other Cs-based perovskites, so its application advantages in the field of solar cells are limited. However, CsPbBr2I can be used as color changing glass, because the high-temperature phase is orange, and the low-temperature phase is nearly colorless, due to the reversibility of the phase transformation process. In the colorless-to-orange process, only heat is required, and in the orange-to-colorless process, only water induction is needed. Therefore, compared with AgBr, the use of CsPbBr2I in color-changing glass has the advantages of low cost and easy synthesis, leading to broad application prospects in the construction and automobile industries[45].
Acknowledgements
This work was funded by the National Natural Science Foundation of China (52073131, 51902148, 61704099, 61874166, U1832149, 51801088 and 51802024), the Natural Science Foundation of Gansu Province (20JR5RA227, 20JR5RA217, 20JR5RA278), and the Fundamental Research Funds for the Central Universities (lzujbky-2020-61, lzujbky-2019-88 and lzujbky-2020-kb06).
Optimization strategy | Perovskite structure | Perovskite fabrication method | VOC (V) | JSC (mA/cm2) | FF (%) | PCE (%) | Ref. |
Spin coating method | Glass/FTO/c-TiO2/CsPbBr2I/Au | Dual source evaporation | 0.959 | 8.7 | 0.56 | 4.7 | [22] |
ITO/SnO2/CsPbBr2I/Spiro-OMeTAD/Ag | Preheating assisted spin-coating | 1.267 | 10.69 | 0.71 | 9.86 | [62] | |
FTO/c-TiO2/CsPbBr2I/Carbon | One-step spin coating | 1.171 | 9.01 | 0.52 | 5.49 | [58] | |
Intermolecular exchange | 1.245 | 10.66 | 0.69 | 9.16 | [59] | ||
FTO/SnO2/CsPbBr2I/Spiro-OMeTAD/Au | Seed-assisted growth | 1.21 | 11.94 | 0.73 | 10.47 | [63] | |
Glass/FTO/bl-TiO2/c-TiO2/CsPbBr2I/ Spiro-OMeTAD/Au | Spray assisted deposition | 1.121 | 7.94 | 0.7 | 6.3 | [65] | |
Interface modification | ITO/SnO2 NPs/CsPbBr2I/CsXth/P3HT/Au | One-step spin coating | 1.3 | 10.19 | 0.738 | 9.78 | [72] |
FTO/TiO2(CsBr)/CsPbBr2I/Carbon | Intermolecular exchange | 1.261 | 11.8 | 0.72 | 10.71 | [73] | |
FTO/TiO2/SmBr3/CsPbBr2I/Spiro-OMeTAD/Au | One-step spin coating | 1.17 | 12.75 | 0.73 | 10.88 | [74] | |
FTO/SnO2/TiO2/CsPbBr2I/carbon | 1.273 | 10.91 | 0.66 | 9.31 | [75] | ||
ITO/In2S3/CsPbBr2I/Spiro-OMeTAD/Ag | 1.09 | 7.76 | 0.66 | 5.59 | [79] | ||
Glass/FTO/ZnO/CsPbBr2I/Carbon | 1.03 | 11.6 | 0.63 | 7.6 | [78] | ||
Glass/FTO/c-TiO2/Li-CsPbBr2I/ CuPc/Carbon | 1.22 | 10.27 | 0.74 | 9.25 | [87] | ||
Ion doping | FTO/c-TiO2/m-TiO2/ CsPb0.995Mn0.005Br1.99 I1.01/Carbon | Two steps spin coating | 0.99 | 13.15 | 0.57 | 7.36 | [91] |
FTO/c-TiO2/m-TiO2/ CsPb0.9Sn0.1Br2I/Carbon | 1.26 | 14.3 | 0.63 | 11.33 | [64] | ||
Glass/FTO/c-TiO2/CsPb(Ba)Br2I/Spiro-OMeTAD/Au | One-step spin coating | 1.19 | 11.91 | 0.74 | 10.51 | [93] |
Table1.
Perovskite structure, preparation techniques, and device performance for all reported variants.
Table options
-->
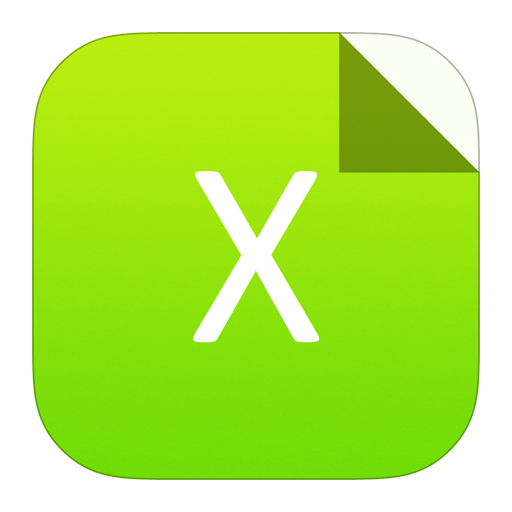
Download as CSV
Optimization strategy | Perovskite structure | Perovskite fabrication method | VOC (V) | JSC (mA/cm2) | FF (%) | PCE (%) | Ref. |
Spin coating method | Glass/FTO/c-TiO2/CsPbBr2I/Au | Dual source evaporation | 0.959 | 8.7 | 0.56 | 4.7 | [22] |
ITO/SnO2/CsPbBr2I/Spiro-OMeTAD/Ag | Preheating assisted spin-coating | 1.267 | 10.69 | 0.71 | 9.86 | [62] | |
FTO/c-TiO2/CsPbBr2I/Carbon | One-step spin coating | 1.171 | 9.01 | 0.52 | 5.49 | [58] | |
Intermolecular exchange | 1.245 | 10.66 | 0.69 | 9.16 | [59] | ||
FTO/SnO2/CsPbBr2I/Spiro-OMeTAD/Au | Seed-assisted growth | 1.21 | 11.94 | 0.73 | 10.47 | [63] | |
Glass/FTO/bl-TiO2/c-TiO2/CsPbBr2I/ Spiro-OMeTAD/Au | Spray assisted deposition | 1.121 | 7.94 | 0.7 | 6.3 | [65] | |
Interface modification | ITO/SnO2 NPs/CsPbBr2I/CsXth/P3HT/Au | One-step spin coating | 1.3 | 10.19 | 0.738 | 9.78 | [72] |
FTO/TiO2(CsBr)/CsPbBr2I/Carbon | Intermolecular exchange | 1.261 | 11.8 | 0.72 | 10.71 | [73] | |
FTO/TiO2/SmBr3/CsPbBr2I/Spiro-OMeTAD/Au | One-step spin coating | 1.17 | 12.75 | 0.73 | 10.88 | [74] | |
FTO/SnO2/TiO2/CsPbBr2I/carbon | 1.273 | 10.91 | 0.66 | 9.31 | [75] | ||
ITO/In2S3/CsPbBr2I/Spiro-OMeTAD/Ag | 1.09 | 7.76 | 0.66 | 5.59 | [79] | ||
Glass/FTO/ZnO/CsPbBr2I/Carbon | 1.03 | 11.6 | 0.63 | 7.6 | [78] | ||
Glass/FTO/c-TiO2/Li-CsPbBr2I/ CuPc/Carbon | 1.22 | 10.27 | 0.74 | 9.25 | [87] | ||
Ion doping | FTO/c-TiO2/m-TiO2/ CsPb0.995Mn0.005Br1.99 I1.01/Carbon | Two steps spin coating | 0.99 | 13.15 | 0.57 | 7.36 | [91] |
FTO/c-TiO2/m-TiO2/ CsPb0.9Sn0.1Br2I/Carbon | 1.26 | 14.3 | 0.63 | 11.33 | [64] | ||
Glass/FTO/c-TiO2/CsPb(Ba)Br2I/Spiro-OMeTAD/Au | One-step spin coating | 1.19 | 11.91 | 0.74 | 10.51 | [93] |