1.
Introduction
Due to its significant physical and chemical properties, as well as its broad application prospects in various fields, such as spintronic devices, sensors, solar cell and photocatalysis, etc., TiO2 has garnered great attention from both academia and industry[1, 2]. This is particularly true of its possible utilization in developing spintronic devices, due to a combination of semiconducting properties and ferromagnetic features, i.e., the simultaneous utilization of both charge and spin characteristics in a single material, RTFM in TiO2-based dilute magnetic semiconductors (DMS) has been intensively investigated both experimentally and theoretically over the past decade, since the pioneering discovery of ferromagnetism exhibited at about 400 K in cobalt-doped TiO2 films by Matsumoto et al. in 2001[3].
According to the available reported results, TiO2-based DMS include the following main categories: pristine TiO2[4], transition metal- (Fe, Co, Mn, Ni, V, etc.) doped TiO2[2, 5-7], rare earth- (Sm, Gd, etc.) doped TiO2[8, 9], nonmagnetic metal- (Cu, Au, etc.) doped TiO2[10, 11], nonmetal- (C, N, etc.) doped TiO2[12], and TiO2 systems, co-doped by different types of dopants[13], among which the transition metal-doped TiO2 system has the widest appeal, owing to its notable and stable RTFM[14-18]. In particular, Co-TiO2 DMS is considered to be the most promising candidate for the development of spintronic devices, by virtue of its remarkable ferromagnetism and stable Curie temperature at or above room temperature, as well as its simultaneous capacity for RTFM and tunable conductivity in a variety of morphologies[19-23]; as such, it has been the focus of a great deal of research[18-26].
However, the Co-TiO2 system is deemed to be more controversial than other TiO2-based DMS, as the room-temperature magnetism in this system, and its corresponding origins, as reported by different works, are extremely inconsistent, or even contradictory[27-31]. The debate in this issue focuses primarily on whether the RTFM of this system is an intrinsic property, originating from ferromagnetic exchange coupling between the Co ions, mediated by oxygen vacancy defects, or is driven by Co related impurity phases (Co clusters or tricobalt tetroxide) aggregated among the TiO2 lattices.
Based on in-depth investigation, researchers incline to the viewpoint that oxygen vacancies are the decisive factor in inducing RTFM in Co-TiO2, since an increasing number of results have demonstrated that RTFM can easily be trigged by introducing oxygen vacancy defects into the Co-TiO2 lattice, and the RTFM is evidently enhanced, along with the concentration of oxygen vacancies[17, 31]. However, it remains an open question as to whether the RTFM in Co-TiO2 will maintain its enhanced state with a consecutive increase in oxygen vacancies. What will the room temperature magnetism be when excessive oxygen vacancies are introduced into a Co-TiO2 lattice? There is currently a paucity of relevant research on this issue.
Clearly, the origin of RTFM in the Co-TiO2 system is far from being thoroughly understood, and the influence of oxygen vacancies on RTFM should be further clarified. Moreover, the magnetism, particularly the magnetization in the Co-TiO2 system, varies dramatically, depending on the preparation procedure and process conditions, and the reported Ms value is too low to satisfy the demand of spintronic devices. In this regard, more efforts should be devoted to addressing these two issues, so as to boost the feasible application of the Co-TiO2 system in the development of spintronic devices.
It has been reported that annealing the samples in vacuum, i.e., under an oxygen-deficient atmosphere, is a very effective method of inducing oxygen vacancies into the TiO2 lattice[32, 33]. Inspired by this experimental route, and with the aim of ascertaining the influence of oxygen vacancies on the room temperature magnetism in Co-doped TiO2, in this work, Co-TiO2 nanoparticles are prepared using a sol–gel technology, followed by vacuum annealing the samples for different durations. Vacuum annealing is anticipated to introduce oxygen vacancies into the Co-TiO2 lattice, and different annealing durations are assumed to facilitate tuning of the oxygen vacancies’ concentration, as well as the room-temperature magnetism. This work has two main objectives: the first is to further clarify the influence of oxygen vacancies on room-temperature magnetism, and the second is to achieve the high magnetization suitable for spintronic devices. The influence of oxygen vacancies on the room temperature magnetism of Co-TiO2 nanoparticles is systematically investigated by intensively assessing the correlation between oxygen vacancies and room-temperature magnetism in Co-TiO2 nanoparticles, and the corresponding origin for the RTFM of Co-TiO2 nanoparticles is anticipated to finally be ascertained.
2.
Experimental methods
2.1
Ti0.97Co0.03O2 samples preparation
The nominal doping ratio of Co-TiO2 nanoparticles is 0.03, and the component is Ti0.97Co0.03O2; the samples were prepared using a sol–gel method similar to that reported in previous works[32-35]. A stoichiometric amount of tetra-n-butyl titanate was added into ethanol (30 mL) under continuous stirring for 30 min at room temperature to obtain the first solution. At the same time, the required quantity of Co(NO3)2·4H2O was dissolved into a mixed solution, containing 28 mL ethanol, 7 mL deionized water, 0.5 mL hydrochloric acid and 20 mL acetic acid, after which the mixed solution was continuously stirred for 30 min at room temperature to obtain the second solution. The second solution was then mixed dropwise into the first solution, followed by vigorous stirring for 4 h to hydrolyze the mixed solution into a pink transparent Ti0.97Co0.03O2 precursor sol. The purpose of adding a minute quantity of hydrochloric acid was to tune the pH value of the mixed solution, thereby controlling the hydrolysis rate.
After aging in air under ambient conditions for 72 h, the Ti0.97Co0.03O2 precursor sol was dried in a drying oven at 100 °C for 24 h to obtain the Ti0.97Co0.03O2 precursor gel, which was then annealed in a vacuum tube furnace, under a vacuum pressure of 11.2 Pa at 450 °C for different durations (1, 2, 3, 4, 5, 6, 7, and 8 h, respectively), and then naturally cooled to room temperature in the furnace after annealing. Finally, a set of Ti0.97Co0.03O2 samples was obtained; the products are designated as TC1, TC2, TC3, TC4, TC5, TC6, TC7, and TC8, respectively.
2.2
Ti0.97Co0.03O2 samples characterization
The microstructure of the all Ti0.97Co0.03O2 samples was measured using an X-ray diffraction spectrometer (XRD, Miniflex Ⅱ) with I = 25 mA and V = 30 kV, Cu Kα radiation, and λ = 0.154056 nm. The scanning angle, 2θ, ranges from 15° to 80°, and the step size was 0.02°. The morphology images were detected using a field-emission scanning electron microscopy (FE-SEM, JSM-7401F). Raman spectra ranging from 100 to 800 cm–1 were recorded using a Raman spectrophotometer (DXR, Thermo Fisher) operated under an excitation source of 532 nm. The existence, as well as the various trends in the oxygen vacancy defects of all the samples was determined by means of electron paramagnetic resonance spectroscopy (EPR, Bruker). The valence state of Co and Ti ions in all the Ti0.97Co0.03O2 samples was measured via X-ray photoelectron spectroscopy (XPS, Escalab 250Xi, Thermo Fisher). The room-temperature magnetism of the Ti0.97Co0.03O2 samples as a function of externally applied magnetic field (M–H curves) was detected using a vibrating sample magnetometer (VSM, VersaLab magnetometer), with an externally applied magnetic field ranging from –30 to +30 kOe at room temperature.
3.
Results and discussion
3.1
Microstructure and micromorphology analysis
XRD patterns for all the samples are presented in Fig. 1(a). We note that all the samples present similar XRD diffraction peaks, separately located at around 25.04°, 37.74°, 47.70°, 53.88°, 54.78°, 62.40°, 68.62°, 70.04°, and 75.02°, corresponding to the characteristic XRD spectra for anatase TiO2. No impurity phases, such as rutile, metallic cobalt, oxides of cobalt, etc., are observed, indicating the satisfactory incorporation of Co ions into the TiO2 lattice, and the monophase of anatase in all the samples. An enlarged view of the peaks for the lattice plane (101) (indexing to 25.04° angle) is provided in Fig. 1(b). This clearly shows that the (101) peaks shift gradually to lower diffraction angles with an increase in annealing duration. To further reveal the influence of annealing duration on the samples’ microstructure, the average crystallite size, D, and the interplanar spacing of (101) plane, d101, are calculated for all the samples, using the Scheler formula and the Bragg formula, respectively, as shown in Fig. 1(c). The results indicate that both D and d increase continuously with an increase in annealing duration, demonstrating the lattice distortion caused by annealing duration[33].

class="figure_img" id="Figure1"/>
Download
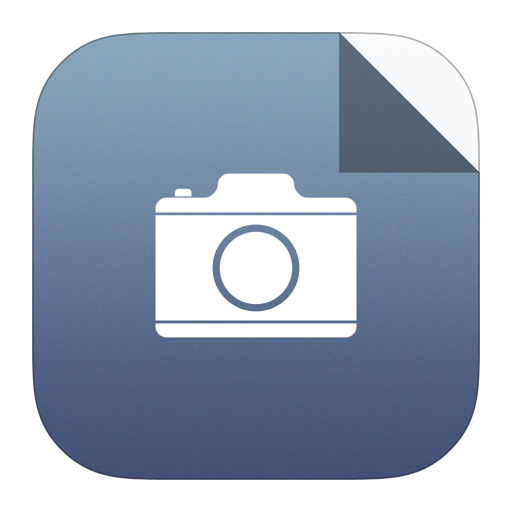
Larger image
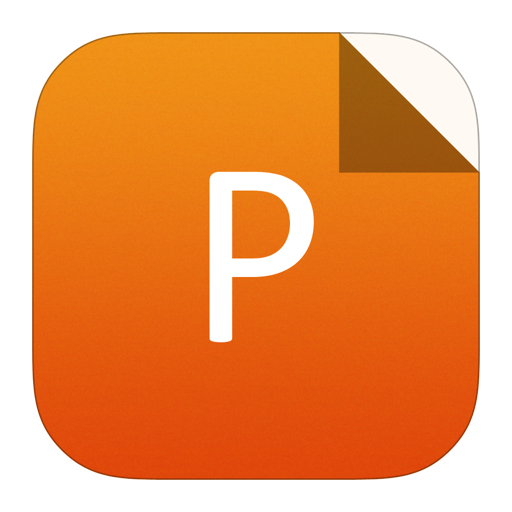
PowerPoint slide
Figure1.
(a) XRD patterns for all the samples. (b) Enlarged view of all the (101) diffraction peaks. (c) Average crystallite size, and interplanar spacing as a function of the annealing duration.
As noted in our previous work[33], oxygen vacancy defects are introduced into the lattice when TiO2 samples are annealed under vacuum; the degree of crystallization is undermined by these defects, inducing lattice distortion. Therefore, it can reasonably be inferred that oxygen vacancies are induced in all the samples used in this work, and the concentration of oxygen vacancies will certainly increase in line with annealing duration, due to the concentration gradient of oxygen atoms between the annealing atmosphere and the samples. As has been demonstrated theoretically[36, 37], oxygen vacancies in the TiO2 lattice will cause relaxation of Ti–Ti and Ti–O bonds, owing to the outward movement of Ti atoms to strengthen the bonding of Ti–Ti and Ti–O; thus the lattice expands gradually with the increase in oxygen vacancies, and the crystallinity of the samples is gradually distorted. Santara et al.[38] also observed similar lattice expansion and crystallinity distortion in a TiO2 lattice, caused by the presence of oxygen vacancies.
The micromorphology for the samples is presented in Fig. 2. We find that all the samples exhibit similar morphology, and are composed of a large number of particles, and the micromorphology is nearly spherical, with diameters ranging from around 7 to 10 nm, comparable to the crystallite size calculated based on the XRD data. The SEM patterns confirm that Co-TiO2 nanoparticles have been successfully synthesized.

class="figure_img" id="Figure2"/>
Download
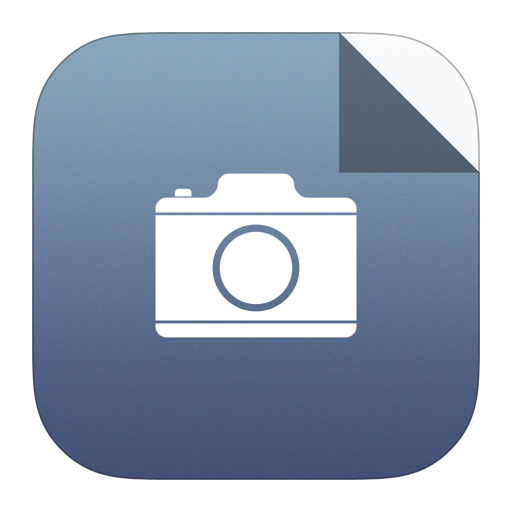
Larger image
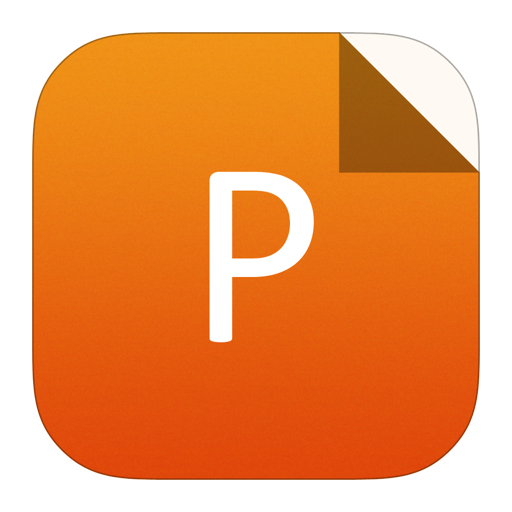
PowerPoint slide
Figure2.
SEM images for all samples.
3.2
Raman scattering analysis
Raman spectra for all the samples are shown in Fig. 3. It is evident that all the samples exhibit similar Raman behaviors, with five Raman scattering peaks, approximately centered at 151.06, 199.48, 395.80, 513.12, and 635.57 cm–1, respectively. All these Raman scattering peaks respectively correspond to the Raman active modes of Eg(1), Eg(2), B1g, A1g, and Eg(2) for anatase TiO2[39]. No other Raman peaks assigned to rutile, metallic cobalt, or oxides of cobalt are observed from the Raman spectra, confirming the monophase of anatase in all the samples, and impurity phases, with specific reference to Co-related clusters, or tricobalt tetroxide in the TiO2 lattices, can be ruled out. Furthermore, the Raman spectra indicate that the intensity of all the Raman scattering peaks is reduced gradually relative to annealing duration, signifying that the crystallinity of the samples is gradually damaged with the increase in annealing duration, which is consistent with the results shown in the XRD patterns.

class="figure_img" id="Figure3"/>
Download
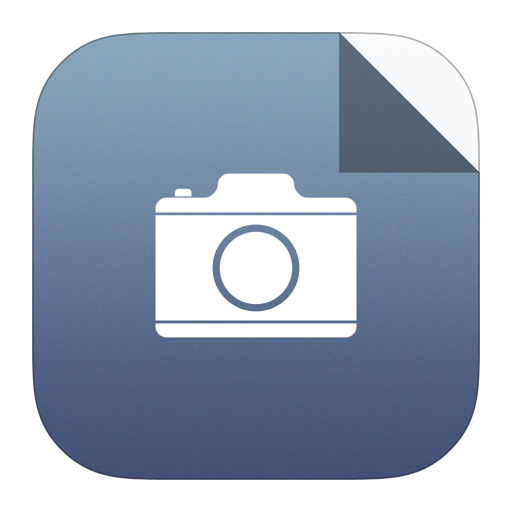
Larger image
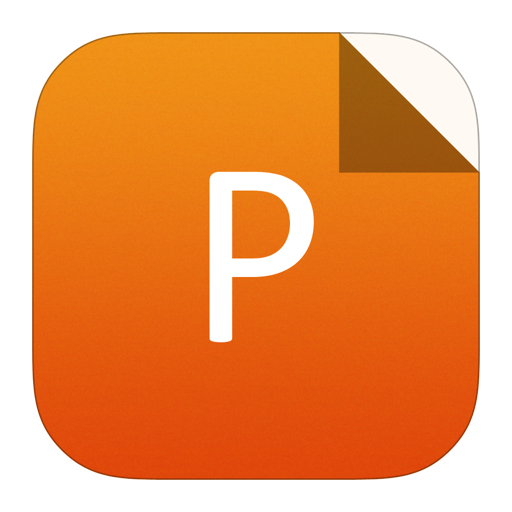
PowerPoint slide
Figure3.
Raman spectra for all samples. The inset shows an enlarged view of all the Eg(1) Raman peaks.
It has been demonstrated that the Eg(1) mode located at 151.06 cm–1 is extremely sensitive to the oxygen vacancy defects in the TiO2 lattice, and that variations in oxygen vacancy concentration can be deduced based on the shift in this scattering peak[30-33]. Here, we observe that the Eg(1) mode of the Co-TiO2 samples exhibits blue shift towards high Raman shift, as shown in the inset of Fig. 3, indicating that the oxygen vacancy concentration steadily increases in line with vacuum annealing duration. Combining the results and analysis of both XRD and Raman scattering, we can conclude that vacuum annealing duration has a significant impact on the microstructure of the Co-TiO2 samples.
3.3
EPR results analysis
To further corroborate the influence of vacuum annealing duration on variations in oxygen vacancy concentrations, EPR spectra for the samples were obtained via electron paramagnetic resonance spectroscopy, and are shown in Fig. 4. All the samples exhibit similar EPR behavior, with g-values of g ≈ 2.005, indicating the presence of oxygen vacancy defects in all the samples. Moreover, as discussed in our previous work[33], the intensity of the EPR signal resulting from oxygen vacancies is indicative of the concentration of oxygen vacancies in the TiO2 lattice.

class="figure_img" id="Figure4"/>
Download
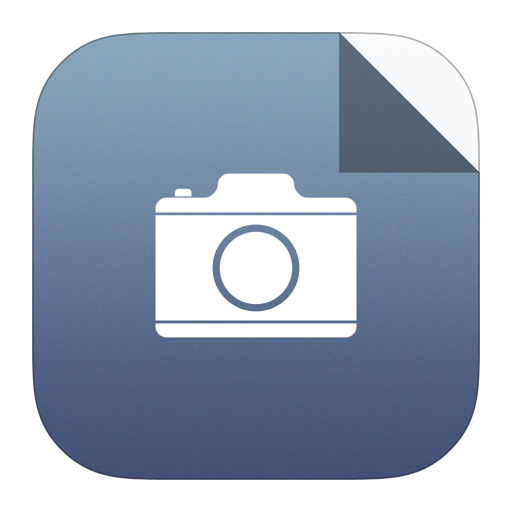
Larger image
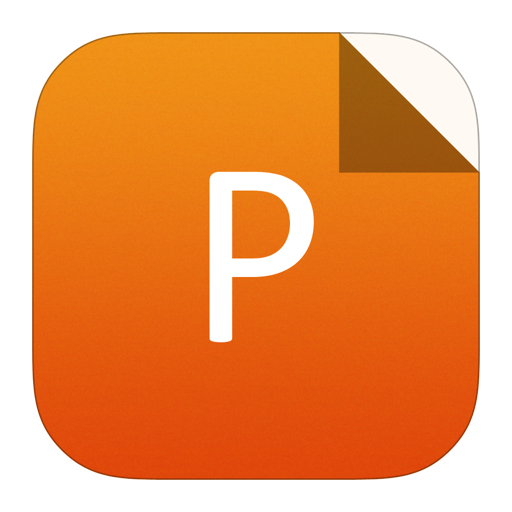
PowerPoint slide
Figure4.
EPR spectra for all samples.
We can therefore assert, based on the variation in the intensity of the EPR peaks, that the concentration of oxygen vacancies in the Co-TiO2 lattice rises steadily with vacuum annealing duration, with the TC8 sample having the highest concentration of oxygen vacancies, while that in TC1 is the lowest. As such, the variation trend in the oxygen vacancies content inferred from XRD and Raman scattering results is confirmed.
3.4
XPS analysis
The chemical state of Co and Ti elements in the Co-TiO2 lattice is determined via X-ray photoelectron spectroscopy, and the core level XPS spectra for Co 2p are presented in Fig. 5. This shows that there are two main XPS peaks, separately located at 781.03 and 796.88 eV, corresponding to the core level Co 2p3/2, and Co 2p1/2, respectively. The difference in the binding energy for these two XPS peaks is 15.8 or 15.9 eV in all the samples, indicating that the chemical state of Co in all the Co-TiO2 samples is the divalent state of Co2+[16].

class="figure_img" id="Figure5"/>
Download
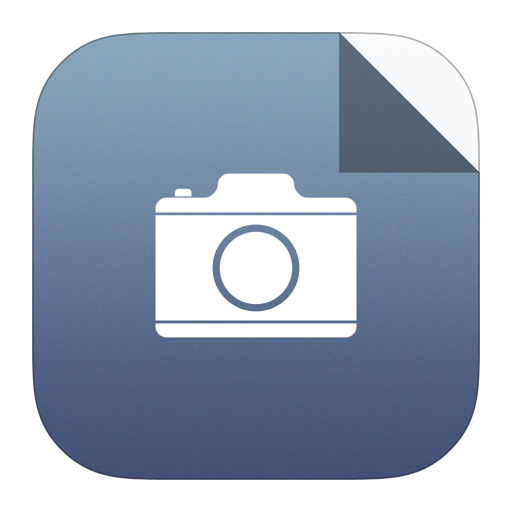
Larger image
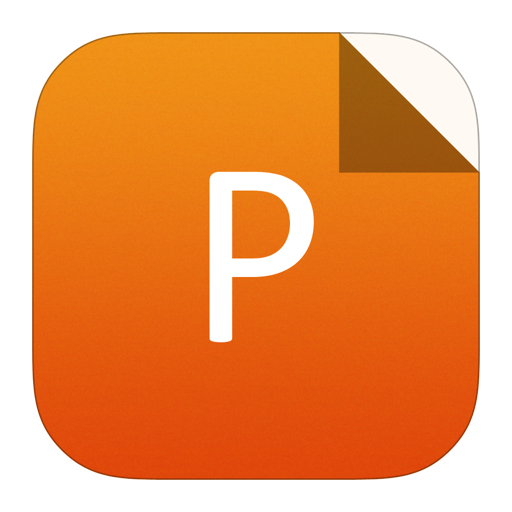
PowerPoint slide
Figure5.
Core level XPS spectra of Co 2p for all samples.
Meanwhile, two extra XPS peaks, located at 786.69 and 803.01 eV, are observed in the XPS spectra, which are respectively ascribed to the resonant shake-up satellite peaks for the multiplet of Co 2p3/2 and Co 2p1/2, further demonstrating the divalent state of Co2+ in all the as-synthesized samples[40, 41]. It has been reported that the XPS peak for metallic Co 2p3/2 is located approximately at 778.3 eV[31, 41], but no obvious signal corresponding to this peak is detected here, and as such, the presence of metallic Co clusters in all the samples can be ruled out, and the incorporation of Co into the TiO2 lattice in the divalent state of Co2+ is confirmed.
The core-level XPS spectrum of Ti 2p for the TC1 sample is presented in Fig. 6. It is apparent that two main XPS peaks separately center at banding energies of 458.7 and 464.5 eV, which are indexed to Ti 2p3/2, and Ti 2p1/2, respectively. The banding energy difference between these two peaks is 5.8 eV, indicating the tetravalent state of Ti4+ in TC1[31]. The XPS spectra of the remaining samples are similar to that of TC1, indicating the presence of tetravalent Ti4+ in all the samples.

class="figure_img" id="Figure6"/>
Download
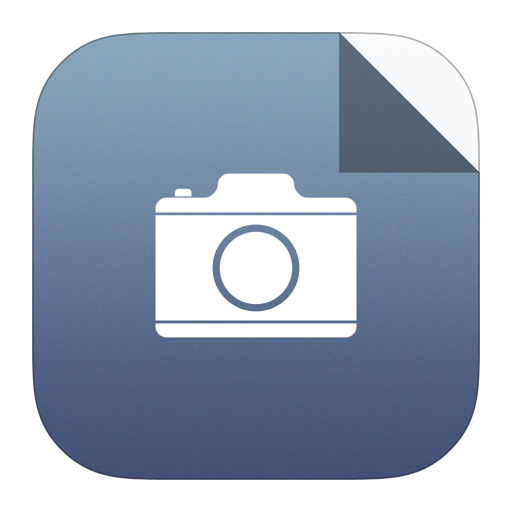
Larger image
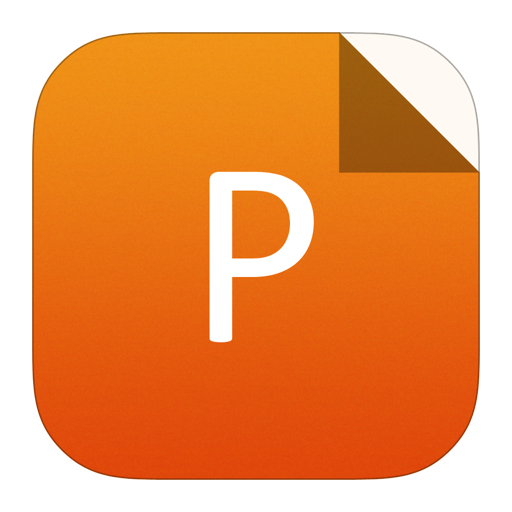
PowerPoint slide
Figure6.
Core level XPS spectrum of Ti 2p for the TC1 sample.
The radius of Co2+ (7.5 × 10–11 m) is larger than that of Ti4+ (6.1 × 10–11 m), but small variations in d101, impacted by increasing annealing duration, is observed in the XRD analysis, indicating that most of the Co2+ ions are located in the interstitial sites or on the surface[31]. Since the bond length of Ti–O in standard anatase ((1.934–1.980) × 10–10 m) is much larger than the radius of Co2+, it can reasonably be inferred that more and more interstitial Co2+ will gather together, due to the recombination and relaxation of Ti–Ti and Ti–O bonds caused by the outward movement of Ti atoms, as revealed in the XRD analysis; as such, the distance between neighboring Co2+ ions will be reduced.
3.5
Room-temperature magnetism analysis
Magnetization as a function of the external magnetic field (M–H curve), ranging from –30 to +30 kOe at room temperature for all the samples, is displayed in Fig. 7. It is apparent in Fig. 7(a) that the TC1, TC2, TC3, TC4, TC5, and TC6 samples possess the typical magnetic hysteresis loop, with the magnetization saturating under a high magnetic field, demonstrating the occurrence of ferromagnetism at room temperature in these samples. Conversely, the magnetization of the TC7 sample rises sharply with the external magnetic field without any sign of saturation, even under a magnetic field of up to 30 kOe, as shown in Fig. 7(b), which indicates the coexistence of paramagnetic phase and a fraction of ferromagnetic phase in the TC7 sample. The magnetic property of TC7 is similar to that reported in previous work[32]. With regard to the TC8 sample, the magnetization linearly increases with the external magnetic field, as shown in Fig. 7(c), which is a clear demonstration of room-temperature paramagnetism in the TC8 sample.

class="figure_img" id="Figure7"/>
Download
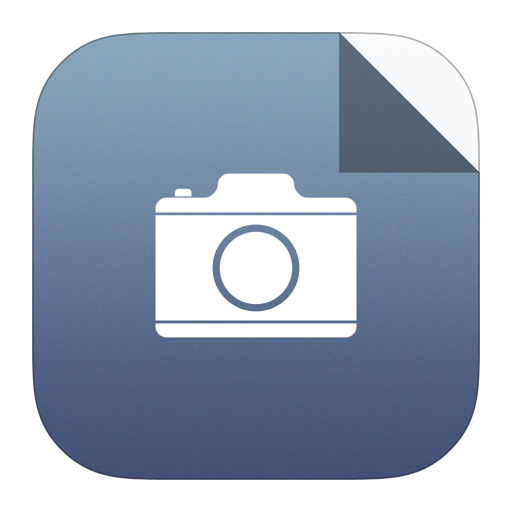
Larger image
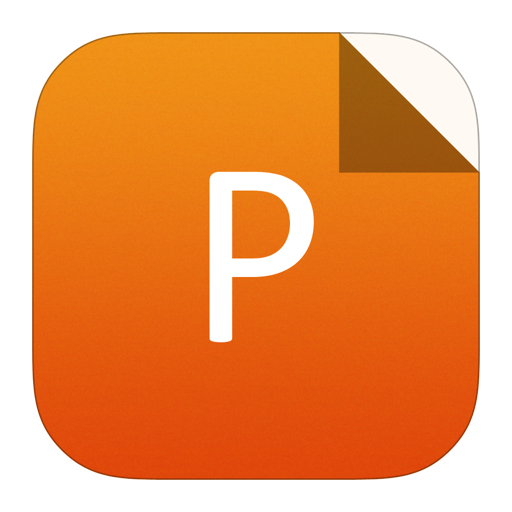
PowerPoint slide
Figure7.
Room-temperature hysteresis loops for all samples: (a) TC1, TC2, TC3, TC4, TC5, TC6, (b) TC7, (c) TC8.
It can therefore be concluded that the room temperature magnetism of the Co-TiO2 nanoparticles is significantly influenced by vacuum annealing duration. When vacuum-annealed for a short duration, less than or equal to 6 h, Co-TiO2 nanoparticles exhibit RTFM, with the Ms value varying significantly with the increase in annealing duration. The Ms shows an initial gradual increases in line with the annealing duration, and reaches a maximum of 1.19 emu/g when vacuum annealed for 4 h, and then sharply declines with a further increase in annealing duration, as shown in Fig. 8. On extending the annealing duration beyond 6 h, the RTFM rapidly weakens, but paramagnetism raises dramatically, and the RTFM eventually undergoes a complete transformation into paramagnetism, based on increased annealing duration. In addition, the maximum Ms obtained in this work is far higher than the reported results[16, 17, 30, 31, 42]. From perspective of the application of DMS in spintronic devices, enhanced RTFM, and, in particular, strong Ms, are among the desired properties pursued by researchers[1, 43]. In this regard, the maximum Ms obtained here is a promising value with respect to the potential development of spintronic devices.

class="figure_img" id="Figure8"/>
Download
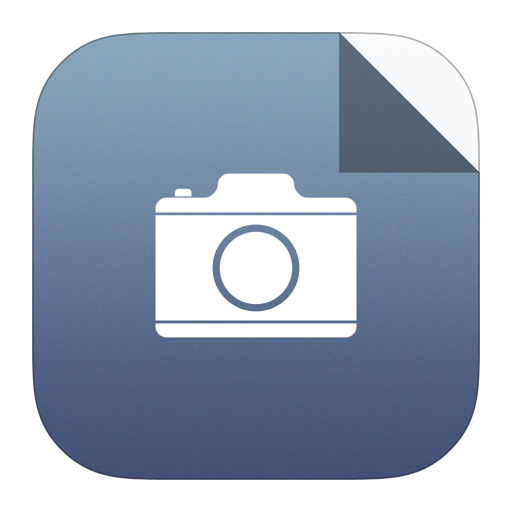
Larger image
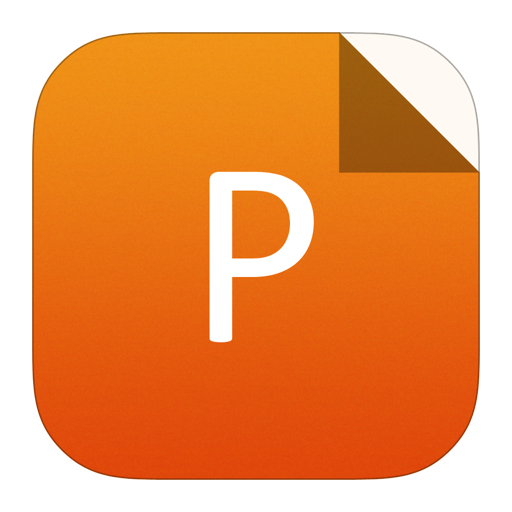
PowerPoint slide
Figure8.
Saturation magnetization as a function of annealing duration for all samples.
According to the analysis above, we conclude that vacuum annealing for different durations is an extremely effective approach to tuning room temperature magnetization, as well as the concentration of lattice defects, in particular the oxygen vacancy content of Co-TiO2 nanoparticles. Moreover, it can be deduced from the analysis that there must be a connection between RTFM and oxygen vacancies in Co-TiO2 nanoparticles. The RTFM is initially enhanced with the increased oxygen vacancy concentration, and then sharply declines, even disappears, with a further increase in the concentration of oxygen vacancies. It can therefore reasonably be inferred that oxygen vacancies, modulated by annealing duration, play a critical role in tuning room-temperature magnetism, since no impurity phase is present in the samples, and oxygen vacancy defects are the sole endogenous variable affecting the level of room-temperature magnetism.
As has been demonstrated, Co2+ ions are apt to align with neighboring Co2+ ions, coupling via oxygen vacancies to form bound magnetic polarons (BMPs), and triggering ferromagnetic exchange interaction; hence, RTFM occurs in Co-TiO2 nanoparticles[28]. Accordingly, RTFM in Co-TiO2 nanoparticles will certainly be sustainably enhanced by an increase in oxygen vacancies.
Nevertheless, an excessive oxygen vacancy content will reduce the distance between neighboring Co2+ ions, owing to the lattice distortion caused by oxygen vacancy defects, as revealed in the XRD and XPS results; the nearest neighboring Co2+ ions will therefore couple with each other to form Co2+–Co2+, giving rise to antiferromagnetic super exchange interaction between Co2+ ions[31]. As such, the RTFM will decline or even disappear with further increases in oxygen vacancies, due to the strengthening of antiferromagnetic superexchange interaction, and paramagnetism is consequently induced in the Co-TiO2 nanoparticles. To summarise, appropriate concentration of oxygen vacancies is beneficial in terms of promoting RTFM in Co-TiO2 nanoparticles, while an excessive oxygen vacancy content will result in a negative impact on RTFM, inducing paramagnetism.
4.
Conclusion
In conclusion, in this work, a series of Co-TiO2 nanoparticles were successfully prepared by a sol–gel route, followed by vacuum annealing of the resulting samples for different durations. The contribution of oxygen vacancies to room-temperature magnetism in the Co-TiO2 system has been systematically clarified by investigating the influence of different annealing duration on oxygen vacancy defects, as well as on magnetism. Our four conclusions can be summarized as follows:
(1) Vacuum annealing for different durations is a very effective method of tuning the concentration of oxygen vacancies and RTFM in a Co-TiO2 system.
(2) Oxygen vacancies play a critical role in generating room temperature magnetism. An appropriate concentration of oxygen vacancies is beneficial for promoting RTFM in Co-TiO2. However, excessive oxygen vacancies cause room-temperature magnetism to transform from ferromagnetism into paramagnetism.
(3) Vacuum annealing for 4 h is the ideal annealing duration required to obtain high RTFM in Co-TiO2 nanoparticles.
(4) The maximum Ms of 1.19 emu/g obtained in this work is a promising value with respect to the future development of spintronic devices.
Acknowledgements
This work was supported by the National Training Program of Innovation and Entrepreneurship for Undergraduates (No. 201910389022).