1.
Introduction
Since the first organic–inorganic hybrid perovskite solar cell (PSCs) was proposed by Miyasaka’s group[1], its power conversion efficiency (PCE) has sky-rocketed from 3.8% to 25.2% over the past decade[2-4]. This quick growth rate has made it as a hotspot in recent years because of its fascinating properties, such as high absorption coefficient[5, 6], low exciton binding energy[7-9], tunable band gap[10, 11], long carrier diffusion length[12, 13], and superb carrier mobility[14, 15]. Though great progresses have been achieved in term of its PCE, there still exist some problems. Volatility and hygroscopic A-site cations decompose the perovskite structure under extreme environment and destroy the performance of the device[16-21].
CsPbX3 is a promising candidate to conquer these problems because Cs+ is the most feasible inorganic cation to replay volatility and hygroscopic A-site with suitable tolerance factor[22-26]. Besides, the structures of organic cation (e.g., CH3NH3+) have orientation freedom, while Cs+ is symmetric without a multiple structure, which make hybrid perovskites show an unstable structure under extreme environments[27-31]. Among all of the CsPbX3 materials, CsPbI3 with a bandgap of ~1.7 eV is a suitable and promising candidate for high performance and stable output photovoltaic material[32-34].
CsPbI3 has four different phases (cubic (α), tetragonal (β), orthorhombic (γ) and non-perovskite yellow (δ) phase) and each phase transforms under different temperatures[35]. At room temperature (RT), CsPbI3 will finally transfer into non-perovskite phase (δ-phase) with an unsuitable bandgap of 2.75 eV, which limits its practical application[36-38]. Many researchers have conducted a series of methods to conquer this problem[39-41]. In the CsPbI3 PSCs development process, HI hydrolysis-derived intermediate plays an important role[42-44]: stabilizing the crystal structure, optimizing the fabricated film and improving the device performance.
In this review, we aim to summarize the latest works about CsPbI3 PSCs based on HI hydrolysis-derived intermediate. First, we briefly review the different crystal and electronic structures of CsPbI3. We then trace the history and disputes of HI hydrolysis-derived intermediate to make this review more logical. Afterward, we highlight the functions of HI hydrolysis-derived intermediate, and systematically summarize some advanced works about HI hydrolysis-derived intermediate on CsPbI3 PSCs. Finally, present issues and outlines are discussed to further increase the CsPbI3 PSCs performance.
2.
Crystal/electronic structure
Photo-electric properties (e.g., optical transitions, charger transfer) are greatly related to crystal and electronic properties (e.g., phase transition, energy band)[45-47]. In this section, we mainly discuss the CsPbI3 perovskite from two aspects: crystal structure and electronic structure.
2.1
Crystal structure
The CsPbI3 perovskite structure can be described as: Pb-site and I-site ion form a corner sharing [PbI6]4– octahedron, while the Cs cation resides in the cuboctahedral cavities[48, 49]. There are mainly four types of structures: cubic structure (α, Pm


class="figure_img" id="Figure1"/>
Download
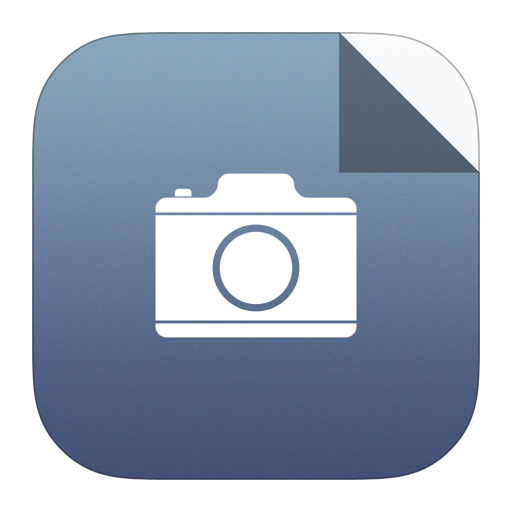
Larger image
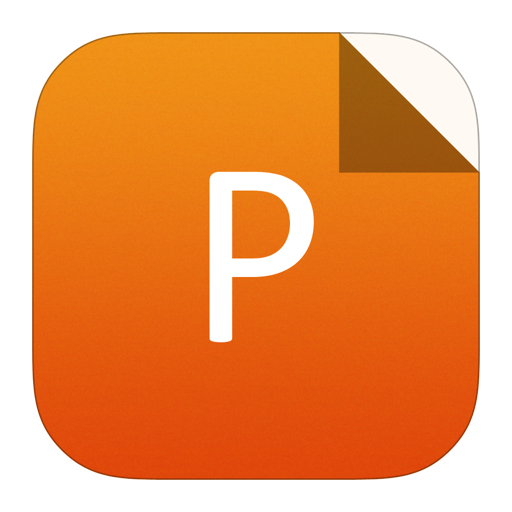
PowerPoint slide
Figure1.
(Color online) (a) The structure and transition of CsPbI3 phases versus temperature. Reproduced with permission[46]. Copyright 2018, American Chemical Society Publications. (b) The transition of CsPbI3 thermal phase and their transition mechanism. Reproduced with permission[35]. Copyright 2019, Science Publishing Group. (c) Schematic of bonding/antibonding orbitals in CsPbX3. Reproduce with permission[54]. Copyright 2016, American Chemical Society Publications. (d) Electronic band structure of CsPbI3 calculated by DFT and (e) tight-binding model. Reproduced with permission[46]. Copyright 2018, American Chemical Society Publications.
The detailed transition temperature between each phase was researched by Even et al., based on density functional theory (DFT) analyzation. The increasing thermal parameters of I– tended to strength the dynamic motion of the corner-connected [PbI6/2]– octahedral, which further induced a change of the unit cell volume and made δ-phase transformed to α-phase at 595 K. Then, with dynamic states gradually relaxing, α-phase transitions to β-phase at 539 K; β-phase transitions to γ-phase at 425 K; finally γ-phase turns to yellow non-perovskite phase (δ-phase) at RT[46].
The different stability of each phases can be ascribed to the different dissociated energies. The dissociation energy from CsPbI3 to CsI and PbI2 for α-, γ-, and δ-phase are 0.04, –0.09 and –0.16 eV, respectively. δ-phase CsPbI3 shows a small Pb–I–Pb bond angel (95.09° and 91.40°) than α-phase CsPbI3 (180°) and γ-phase CsPbI3 (154.74°), which reduces the orbital overlap between Pb and I atoms and also makes δ-phase CsPbI3 with a deeper defect transition energy level than α-, γ-phase. This indicates that δ-phase is the most stable phase because of its lowest dissociation energy[51]. Most importantly, different cooling rates change the formation energies for CsPbI3 phases. α-phase converts to γ-phase when rapidly cooled in dry air, while slowly cooling leads to δ-phase phase because of different formation energies[52]. Besides, changing the temperature to cause the structure transition, a polar solvent also induces lattice distortion in CsPbI3. A polar solvent would induce the lattice distortion of CsPbI3 nanocubes by triggering the dipole moment, which leads to the self-assembly from α-CsPbI3 to a γ-phase through oriented attachment process[53]. These studies indicate that metastable phases (contained β, γ-phase CsPbI3) are more promising than α-CsPbI3.
2.2
Electronic structure
The valence band maximum (VBM) of CsPbI3 perovskite is constituted of antibonding hybridized Pb 6s and X np orbitals, among which X np takes the lead. However, Pb 6p is in the dominant place of conduction band minimum (CBM), as shown in Fig. 1(c)[54]. Compared with organic A-site cations, Cs+ has little effect on CsPbI3 electronic properties. A-site cations could indirectly influence perovskite electronic properties through Coulombic interactions and steric hindrance to deform the perovskite lattice, which makes perovskite electronic structure close to the band edges and further changes the band gap energetics[55].
The calculated electronic of α-, β- and γ-CsPbI3 are depicted in Figs. 1(d) and 1(e)[46]. According to the tight-binding (TB) and DFT structures, taking band folding into account, we can vividly draw that all the different band gaps of CsPbI3 phases are direct, and the band gap of α-CsPbI3 shifts from the R point in the Brillouin zone to Z and Γ for β-CsPbI3 and γ-CsPbI3, respectively. This change indicates that the electronic band gap gradually increases with the transition from α-CsPbI3 to more distorted β- and γ-CsPbI3 because the [PbI6]4– rotations stabilize the top of VBM and destabilize the bottom of CBM[56].
3.
The functions of HI hydrolysis-derived intermediate
We summarize the performance of CsPbI3 PSCs after introducing HI hydrolysis-derived intermediate in Table 1 (sPCE is the stable PCE). Its main functions can be summarized as following:
Material | Configuration | JSC (mA/cm2) | VOC (V) | FF (%) | PCE (%) | sPCE (%) | Ref. |
α-phase CsPbI3 | ITO/PEDOT:PSS/CsPbI3/PCBM/BCP/LiF/Al | 8.17 | 0.870 | 69.0 | 4.88 | – | [62] |
ITO/PEDOT:PSS/CsPbI3/PCBM/BCP/LiF/Al | 5.89 | 0.960 | 64.0 | 3.66 | – | [63] | |
FTO/TiO2/CsPbI3·xEDAPbI4/Spiro/Ag | 14.53 | 1.150 | 71.0 | 11.86 | – | [78] | |
FTO/TiO2/CsPbI3/Carbon | 18.50 | 0.790 | 65.0 | 9.50 | – | [68] | |
ITO/SnO2/LiF/CsPbI3-xBrx/Spiro/Au | 18.30 | 1.234 | 82.6 | 18.64 | – | [70] | |
FTO/TiO2/CsPbI3-x-DETAI3/P3HT/Au | 12.21 | 1.060 | 61.0 | 7.89 | – | [67] | |
FTO/PTAA/OTG3-CsPbI3/PCBM/BCP/Ag | 15.81 | 1.120 | 75.2 | 13.32 | 13.20 | [80] | |
FTO/TiO2/PEAI-CsPbI3/Spiro/Ag | 18.40 | 1.110 | 69.6 | 14.30 | 13.50 | [79] | |
Metastable (β- and γ-) phase CsPbI3 | FTO/TiO2/CsPbI3/PTAA/Au | 18.95 | 1.059 | 74.9 | 15.07 | – | [43] |
FTO/NiOx/STCG-CsPbI3/ZnO/ITO | 18.29 | 1.090 | 80.5 | 16.04 | – | [84] | |
FTO/TiO2/CsPbI3/PTAA/Au | 19.75 | 1.135 | 76.6 | 17.17 | 16.83 | [86] | |
N-CQDs EDS/FTO/TiO2/CsPbI3/PTAA/Au | 19.15 | 1.106 | 75.6 | 16.02 | 15.90 | [89] | |
FTO/TiO2/CsPbI3/PTAA/Au | 18.31 | 1.110 | 78.0 | 15.91 | – | [85] | |
FTO/TiO2/CsPbI3/PTAA/Au | 20.34 | 1.090 | 77.0 | 17.03 | – | [88] | |
FTO/TiO2/CsPbI3/PTAA/Au | 21.15 | 1.090 | 77.0 | 17.30 | 16.78 | [76] | |
FTO/TiO2/CsPbI3/P3HT/Au | 16.53 | 1.040 | 65.7 | 11.30 | 9.70 | [83] | |
FTO/TiO2/CsPbI3/PTAA/Au | 19.58 | 1.084 | 75.7 | 16.07 | 15.47 | 87] | |
FTO/TiO2/CsPbI3/UCNP-PTAA/Au | 19.17 | 1.113 | 74.3 | 15.86 | 15.59 | [90] | |
FTO/TiO2/CsPbI3/PTAA/Au | 20.30 | 1.080 | 75.5 | 16.24 | – | [91] | |
Low dimension CsPbI3 | FTO/TiO2/CsPbI3/PTAA/Au | 19.51 | 0.993 | 70.5 | 13.65 | 13.29 | [98] |
ITO/PTAA/CsPbI3/C60/BCP/Cu | 17.21 | 1.090 | 67.5 | 12.65 | – | [101] | |
ITO/SnO2/CsPbI3/Spiro/Au | 16.59 | 1.070 | 70.0 | 12.40 | – | [97] | |
FTO/TiO2/CsPbI3/Carbon | 15.76 | 0.910 | 66.0 | 9.39 | – | [99] | |
DMAxCs1–xPbI3 | FTO/TiO2/DMA0.15Cs0.85PbI3/Spiro/Ag | 19.40 | 1.050 | 75.0 | 15.30 | – | [75] |
FTO/TiO2/DMAI-CsPbI3/Spiro/Ag | 20.23 | 1.137 | 82.7 | 19.03 | – | [74] | |
FTO/TiO2/Cs0.5DMA0.5PbI3/Spiro/Ag | 18.40 | 1.054 | 74.0 | 14.30 | – | [72] | |
ITO/PEDOT:PSS/Cs0.7DMA0.3PbI3/C60/BCP/Ag | 16.65 | 0.990 | 76.5 | 12.62 | – | [71] | |
FTO/TiO2/DMAI-CsPbI3/Spiro/Ag | 20.23 | 1.110 | 82.0 | 18.40 | – | [73] |
Table1.
Photovoltaic parameters of CsPbI3 PSCs fabricated by HI hydrolysis-derived intermediate.
Table options
-->
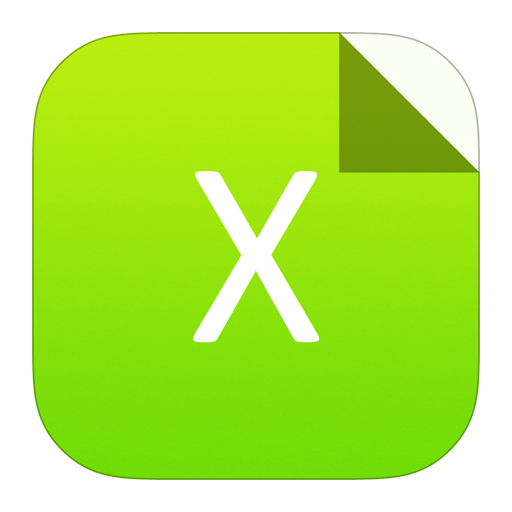
Download as CSV
Material | Configuration | JSC (mA/cm2) | VOC (V) | FF (%) | PCE (%) | sPCE (%) | Ref. |
α-phase CsPbI3 | ITO/PEDOT:PSS/CsPbI3/PCBM/BCP/LiF/Al | 8.17 | 0.870 | 69.0 | 4.88 | – | [62] |
ITO/PEDOT:PSS/CsPbI3/PCBM/BCP/LiF/Al | 5.89 | 0.960 | 64.0 | 3.66 | – | [63] | |
FTO/TiO2/CsPbI3·xEDAPbI4/Spiro/Ag | 14.53 | 1.150 | 71.0 | 11.86 | – | [78] | |
FTO/TiO2/CsPbI3/Carbon | 18.50 | 0.790 | 65.0 | 9.50 | – | [68] | |
ITO/SnO2/LiF/CsPbI3-xBrx/Spiro/Au | 18.30 | 1.234 | 82.6 | 18.64 | – | [70] | |
FTO/TiO2/CsPbI3-x-DETAI3/P3HT/Au | 12.21 | 1.060 | 61.0 | 7.89 | – | [67] | |
FTO/PTAA/OTG3-CsPbI3/PCBM/BCP/Ag | 15.81 | 1.120 | 75.2 | 13.32 | 13.20 | [80] | |
FTO/TiO2/PEAI-CsPbI3/Spiro/Ag | 18.40 | 1.110 | 69.6 | 14.30 | 13.50 | [79] | |
Metastable (β- and γ-) phase CsPbI3 | FTO/TiO2/CsPbI3/PTAA/Au | 18.95 | 1.059 | 74.9 | 15.07 | – | [43] |
FTO/NiOx/STCG-CsPbI3/ZnO/ITO | 18.29 | 1.090 | 80.5 | 16.04 | – | [84] | |
FTO/TiO2/CsPbI3/PTAA/Au | 19.75 | 1.135 | 76.6 | 17.17 | 16.83 | [86] | |
N-CQDs EDS/FTO/TiO2/CsPbI3/PTAA/Au | 19.15 | 1.106 | 75.6 | 16.02 | 15.90 | [89] | |
FTO/TiO2/CsPbI3/PTAA/Au | 18.31 | 1.110 | 78.0 | 15.91 | – | [85] | |
FTO/TiO2/CsPbI3/PTAA/Au | 20.34 | 1.090 | 77.0 | 17.03 | – | [88] | |
FTO/TiO2/CsPbI3/PTAA/Au | 21.15 | 1.090 | 77.0 | 17.30 | 16.78 | [76] | |
FTO/TiO2/CsPbI3/P3HT/Au | 16.53 | 1.040 | 65.7 | 11.30 | 9.70 | [83] | |
FTO/TiO2/CsPbI3/PTAA/Au | 19.58 | 1.084 | 75.7 | 16.07 | 15.47 | 87] | |
FTO/TiO2/CsPbI3/UCNP-PTAA/Au | 19.17 | 1.113 | 74.3 | 15.86 | 15.59 | [90] | |
FTO/TiO2/CsPbI3/PTAA/Au | 20.30 | 1.080 | 75.5 | 16.24 | – | [91] | |
Low dimension CsPbI3 | FTO/TiO2/CsPbI3/PTAA/Au | 19.51 | 0.993 | 70.5 | 13.65 | 13.29 | [98] |
ITO/PTAA/CsPbI3/C60/BCP/Cu | 17.21 | 1.090 | 67.5 | 12.65 | – | [101] | |
ITO/SnO2/CsPbI3/Spiro/Au | 16.59 | 1.070 | 70.0 | 12.40 | – | [97] | |
FTO/TiO2/CsPbI3/Carbon | 15.76 | 0.910 | 66.0 | 9.39 | – | [99] | |
DMAxCs1–xPbI3 | FTO/TiO2/DMA0.15Cs0.85PbI3/Spiro/Ag | 19.40 | 1.050 | 75.0 | 15.30 | – | [75] |
FTO/TiO2/DMAI-CsPbI3/Spiro/Ag | 20.23 | 1.137 | 82.7 | 19.03 | – | [74] | |
FTO/TiO2/Cs0.5DMA0.5PbI3/Spiro/Ag | 18.40 | 1.054 | 74.0 | 14.30 | – | [72] | |
ITO/PEDOT:PSS/Cs0.7DMA0.3PbI3/C60/BCP/Ag | 16.65 | 0.990 | 76.5 | 12.62 | – | [71] | |
FTO/TiO2/DMAI-CsPbI3/Spiro/Ag | 20.23 | 1.110 | 82.0 | 18.40 | – | [73] |
1) Reducing crystallization energy barrier in low temperature fabrication;
2) Increasing iodide coordination numbers to decrease structural disorder, modifying structure and forming higher-order iodoplumbate complexes;
3) Slowing down the rapid crystalline process and obtaining high-quality CsPbI3 film;
4) Inducing strain to generate distorted metastable phase (β- and γ- CsPbI3);
5) Modifying the band gap of perovskites films.
4.
History and disputes of HI hydrolysis-derived intermediate
The solution one-step method has advantages of simple, convenience and facile process, and can also be compatible roll-to-roll fabrication technology[57]. However, the one-step deposition process shows poor morphology and low performance because of the rapid reaction in the solution. The common solution is using intermediates to slow down the quick reaction and make it controllable. However, understanding the composition of the HI hydrolysis-derived intermediate still needs a long time.
4.1
HI
In the early stage, researchers focused on using HI additive in CsPbI3 PSCs fabrication to cause a microstrain and induce a low temperature phase transition process. Meanwhile, extra halides in HI precursor solution tended to fill the vacancies of perovskites, resulting in change of metal–halogen–metal bond connectivity, and consequently cell volumes and optical bandgap[58]. Besides, PbI2 first coordinated with DMF in the precursor through Pb–O bonds, but further added HI would eliminate PbI2–DMF coordination and form higher-order iodoplumbate complexes (e.g., PbI42?, PbI53?, and PbI64?), which benefited the formation of high-quality CsPbI3 film[59, 60].
In 2015, HI was first used as an additive in CsPbI3 PSCs. Snaith et al. introduced a small amount of HI in the precursor solution before spin-coating. They found that HI additive could change the solubility of precursor materials and induce a strain to lower the temperature phase transition. Then, strain triggered small crystals appearing and significantly stabilized its structure in RT, as shown in Fig. 2(a)[42]. Uddin et al. controlled the concentration of HI on purpose to modify CsPbI3 bandgap. They demonstrated that introducing 36 μL/mL HI would decrease the bandgap from 1.75 eV to optimized value of 1.7 eV and show excellence electronic properties with low charge-transport (12.8 kΩ). From the scanning electron microscopy (SEM) images, optimal concentration HI additive led to the appearance of small grain sizes with a few nanometers, which is beneficial to increase the stability of black phase CsPbI3[61]. This conclusion was also proven by Kim et al., who found that HI formed small grains and stabilized the black phase of CsPbI3 at low temperature[62]. Furthermore, they investigated the function of NH4+ (e.g., NH4Cl, NH4Br and NH4I) and H+ (HCl, HBr and HI) based additive and found that HI additive is the most efficient, and which could reduce the roughness and increase the stability of perovskites films. In particular, when it was exposed in ambient for 3 h, the optimal device with CsPbI3 perovskite only dropped its PCE from 3.55% to 2.78%[63].

class="figure_img" id="Figure2"/>
Download
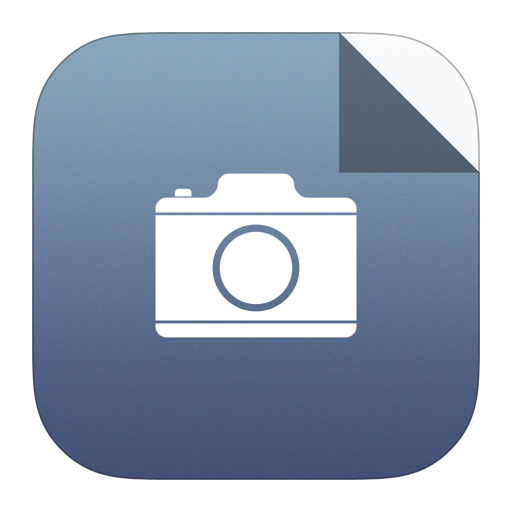
Larger image
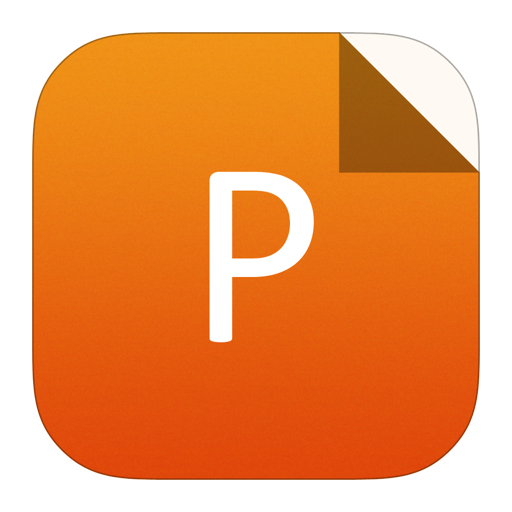
PowerPoint slide
Figure2.
(Color online) (a) The diagrammatic of HI fabricated CsPbI3. Reproduced with permission[42]. Copyright 2015, The Royal Society of Chemistry. (b) Schematic of using HPbI3 to fabricate FAPbI3 PSCs. Reproduced with permission[65]. Copyright 2015, Wiley-VCH Publications. (c) Detail information of PbI2 and HPbI3 fabricated perovskite film. Reproduced with permission[69]. Copyright 2018, Wiley-VCH Publications. (d) The molecular structure of FA and DMA, and the tolerance factor of corresponding perovskite (CsPbI3, Cs0.7DMA0.3PbI3 and DMAPbI3). Reproduced with permission[71]. Copyright 2018, Nature Publishing Group.
4.2
PbI2·xHI or HPbI3
In addition to HI additive, HI hydrolysis-derived intermediate is more effective because it eliminates water in the HI solution and is an intermediate compound to increase perovskite crystallinity[64]. It was first proposed in 2015 by Zhao et al. They developed a new precursor compound (named HPbI3) through a reaction of HI and PbI2 in DMF solution, and used it to replace PbI2 in fabrication FAPbI3-based PSCs, as shown in Fig. 2(b)[65]. Such HPbI3 has a pseudo-3D crystal structure, where 1D face-shared [PbI6]4– octahedra with intercalated protons (H+) for charge balance[66].
Zhu et al. introduced HPbI3 into the CsPbI3 PSCs and assisted with a triple cation NH3+C2H4NH2+C2H4NH3+ (named as DETA3+) to further stabilize the α-CsPbI3 perovskite phases[67]. Subsequently, Chen et al. used HPbI3 to substitute PbI2 and found that tensile lattice strain appeared in HPbI3-process CsPbI3 perovskite[68]. The tensile lattice strain generated because HPbI3 crystals serve as a template to guide the nucleation and growth of α-CsPbI3. A similar work was conducted by Zhao et al., who introduced the I-excess precursor HPbI3+x and replace PbI2 to reduce crystallization energy barrier, and fabricated α-CsPbI3–xBrx PSCs in low temperature (130 °C). The champion PCE of 13.61% was measured by a reverse scanning, as shown in Fig. 2(c)[69]. More recently, in order to overcome the low PCE problem of CsPbI3-based PSC, You et al. used CsI, HPbI3+x and PbBr2 as precursor to fabricate high-quality CsPbI3–xBrx perovskite films. They developed an inorganic shunt-blocking layer lithium fluoride (LiF) in the ETL/perovskite interface to align the bandgap and suppress the surface defect. Furthermore, a small amount of PbCl2 were introduced to further suppress the recombination. Finally, they obtained CsPbI3–xBrx with the highest PCE of 18.64%, and boosted VOC to 1.25 V with little loss. After continuous 1 sun equivalent illumination, the best device dropped only 6% of its initial PCE after 1000 h[70].
4.3
PbI2·xDMAI or DMAPbI3
Kanatzidis et al. recently claimed that HPbI3 did not exist and was replaced by a compound of DMAPbI3, which generated through DMF hydrolysis in HI solution. Importantly, they pointed out that some early reports of inorganic perovskite are actually the hybrid perovskite. They found that DMAPbI3 possessed a larger tolerance factor and mixing with Cs+ could adjust tolerance factor (t) of the compounds (Cs1?xDMAxPbI3) toward an ideal factor (t, 0.9–1). Finally, they achieved a champion PCE of 12.62% in Cs1?xDMAxPbI3-based PSCs, as shown in Fig. 2(d)[71]. Liu et al. dissolved PbI2 and HI in DMF to synthesize DMAPbI3 and further confirmed that no HPbI3 existed. They used it as precursor to fabricate high-quality CsxDMA1?xPbI3 perovskite films with 14.3% PCE, and the initial PCE kept more than 85% when exposed in air 20 days without encapsulation, as shown in Fig. 3(a)[72].

class="figure_img" id="Figure3"/>
Download
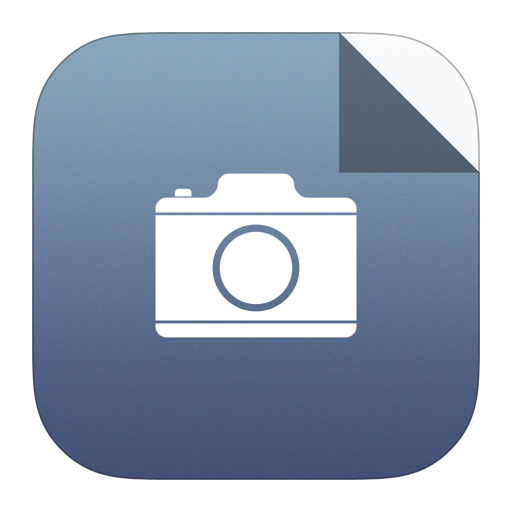
Larger image
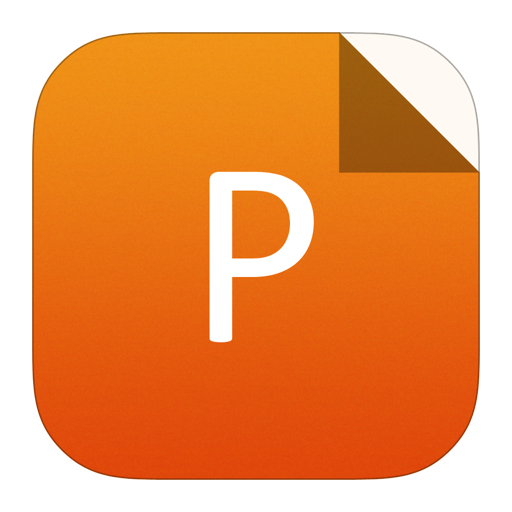
PowerPoint slide
Figure3.
(Color online) (a) Schematic illustration the fabrication process of CsxDMA1–xPbI3. Reproduced with permission[72]. Copyright 2019, Elsevier Inc Publications. (b) Schematic diagram of using DMAI additive to form CsPbI3 films. Reproduced with permission[74]. Copyright 2019, Wiley-VCH Publications. (c) The changeable component of DMAI-fabricated perovskite versus annealing temperature. Reproduced with permission[75]. Copyright 2020, American Chemical Society Publications. (d) Schematic diagram of DMAPbI3 synthesis process and the information of corresponding perovskite. Reproduced with permission[76]. Copyright 2019, Wiley-VCH Publications.
Zhao et al. used PbI2·xDMAI to fabricate CsPbI3 PSCs recently, and they concluded that the fabricated perovskites are actually all inorganic composition because the organic ion DMA+ are easily lost during the high-temperature (210 °C) annealing process[73]. Later, they proved that DMAI is a volatile additive, and used it to assist with phenyltrimethylammonium chloride (PTACl) passivation. Finally, they obtained the highest PCE of CsPbI3 PSC, 19.03%, as shown in Fig. 3(b)[74]. This conclusion was also confirmed by Pang et al., who found that DMAPbI3 and Cs4PbI6 first formed in annealing 100 °C for 10 min. When the annealing temperature was increased to 180 °C for 15 min, DMAPbI3 converted into DMA0.15Cs0.85PbI3 with a small amount Cs4PbI6 residue. Once the annealing temperature exceeded 200 °C, the γ-phase CsPbI3 was formed with a small number of DMA0.15Cs0.85PbI3 residue. The number of DMA0.15Cs0.85PbI3 greatly decreased and a little δ-phase appeared, as shown in Fig. 3(c)[75].
Our groups also confirmed this conclusion. We synthesized a series of intermediate compounds (DMAI and DMAPbI3) by different ratio of HI/DMF, and used them to fabricate CsPbI3 PSCs. After detailed analysis, we found that the major component of CsPbI3 was still inorganic in this reaction route. Most of DMA+ organic molecules lost during the annealing process, and only a small amount of DMA+ remained to stabilize perovskite structure. Excessive DMA+ interacted with Pb2+ to further passivate CsPbI3 surface, as shown in Fig. 3(d)[76].
In conclusion, the organic molecule DMAI mainly influence the crystallization kinetics and perovskite phase. During the annealing process, DMAI will sublimate quickly, change the rate of crystallization and form metastable (β- and γ-) phase based CsPbI3. The controllable crystallization kinetics and stable (β- and γ-) phase are beneficial to morphology and stability of perovskite, respectively. Besides, DMA+ (2.72 ?) possesses a larger ionic radius than Cs+ (1.88 ?)[77]. Therefore, if the DMA+ is residual (non-sublimate) in the CsPbI3 film: (1) DMA+ doped into the lattice of CsPbI3 can increase its tolerance factor for avoiding crystal structure distortion; (2) DMA+ reacted with Pb2+ to passivate CsPbI3 surface, further reducing leakage current generation for increasing the device performance.
5.
Applying HI hydrolysis-derived intermediate in CsPbI3 PSCs
5.1
α-phase CsPbI3 based PSCs
As we discussed earlier, HI hydrolysis-derived intermediate showed a lot of advantages in high-quality film fabrication and device performance. Importantly, perovskite films with better crystallinity, morphology, and higher range of absorption are the foundation of efficiency.
The first working α-CsPbI3 PSCs with a PCE of 2.9% was fabricated in low temperature (100 °C) by Snaith and his cooperators via a small amount HI additive adding[42]. Many relevant works have been done to boost its performance. Uddin et al.[61] and Kim et al.[62] used HI to modify the α-CsPbI3 film morphology and boost its PCE to 6.44% and 4.88%, respectively. Later, Zhao et al. discovered that PbI2.xHI could reduce the crystallization energy barrier for α-CsPbI3 phase. They used PbI2.xHI assist with two-dimension EDAPbI4 perovskite to stabilize α-CsPbI3 phase and avoid lattice distortion. Finally, α-CsPbI3 phase based on the EDAPbI4 passivation showed a record PCE of 11.8% together with superior stability, as shown in Fig. 4(a). The α-CsPbI3 phase perovskite kept its structure after annealing at 100 °C for more than 150 h and stable at RT for months[78].

class="figure_img" id="Figure4"/>
Download
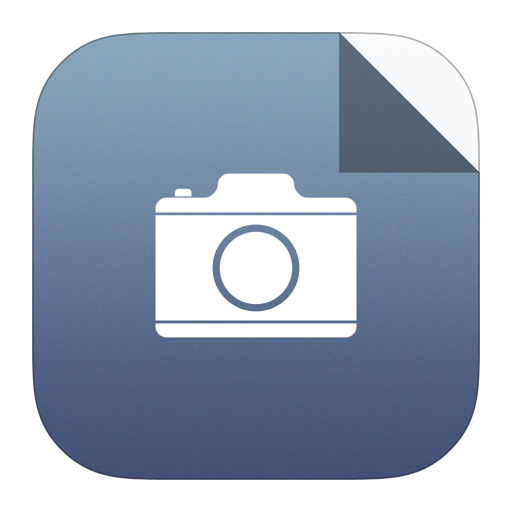
Larger image
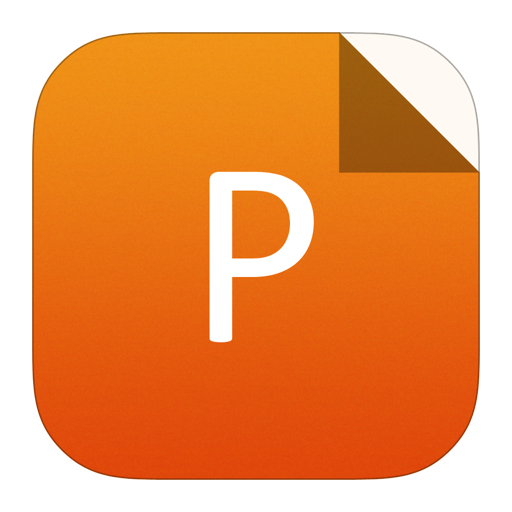
PowerPoint slide
Figure4.
(Color online) (a) The detail information of PbI2.HI and PbI2 fabricated perovskite, inserted pictures are their digital photos. Reproduced with permission[78]. Copyright 2017, Wiley-VCH Publications. (b) The diagram of PbI2 and HPbI3 fabricated CsPbI3 film, respectively. Reproduced with permission[68]. Copyright 2018, American Chemical Society Publications. (c) Schematic of PEA+ organic ligand treatment on CsPbI3 thin film. Reproduced with permission[79]. Copyright 2018, Elsevier Inc Publications. (d) Diagram illustrates the mechanism of with/without OTG passivation. Reproduced with permission[80]. Copyright 2019, Wiley-VCH Publications.
Compared with HI, the absence of H2O molecules in HI hydrolysis-derived intermediate can optimize the perovskite crystallinity and morphology. Chen and his cooperators replaced PbI2 with HPbI3 in fabricating stable α-CsPbI3 film. They found that the bandgap was shifted from 1.72 to 1.68 eV owing to formation of tensile lattice strain. Finally, a HTL free α-CsPbI3 was obtained with a higher PCE of 9.5%. Besides, the optimal device showed enhanced stability, which maintained 90% of its initial PCE under illumination for more than 3000 h in dry environment, as shown in Fig. 4(b)[68]. Then, organic terminal groups were widely used to assist HPbI3 and further improve α-phase CsPbI3 stability. Zhu et al. introduced DETA3+ additive into the HPbI3 containing precursor to stabilize α-CsPbI3 perovskite phases. DETA3+ has NH3+ or RHN2+ group, which could combine with I– or [PbI6]4– and avoid octahedral tilting. Besides, oil-wet (hydrophobic) hydrocarbon chains of DETA3+ increased the CsPbI3 humidity-resistance. Ultimately, the optimal device kept its structure for more than 6 h in a humid environment (~30 °C, 60%–70% RH), while the reference device changed its color from black to yellow in the same condition in 55 min, indicating a phase transition[67]. Zhao et al. fabricated HPbI3+x to form α-CsPbI3 perovskite film. With the help of PEAI post-treated on the α-CsPbI3, superior PCE of 13.5% was obtained with improved stability. Because of PEA+ terminated on the CsPbI3 surface and did as a capping layer, PEA+-CsPbI3 remained stable structure after 80 °C annealing for 7 days, while the pure one degraded into non-perovskite in the same condition, as shown in Fig. 4(c)[79] . Han et al. added organic terminal groups (OTG) into CsI and HPbI3 precursors to design an inverted planar CsPbI3 PSCs. They report that OTG induced a steric hindrance and suppressed octahedral [PbI6]4– tilting. Moreover, OTG passivated the surface electronic traps states to further increase its performances. Finally, the inverted planar OTG-CsPbI3 PSCs showed the highest PCE of 13.2% and retained about 85% of its initial PCE for 30 days at RT, while the reference device degraded completely in 3 weeks, as shown in Fig. 4(d)[80].
5.2
Metastable (β- and γ-) phase CsPbI3 based PSCs
Recently, the CsPbI3 films fabricated by HI hydrolysis-derived intermediate were proved metastable phases (combined β-phase CsPbI3 with γ-phase CsPbI3).
The β-phase CsPbI3 can also be formed at low temperature and show more stable perovskite structure than α-phase one. However, it is difficult to deposit and stabilize its perovskite structure[81]. Zhao et al. adopted PbI2·DMAI and CsI as precursor to fabricate stable β-phase CsPbI3 with stable structure. Furthermore, they used choline iodine (CHI) to passiviate the surface trap states and aligned the energy level in the TiO2/β-phase interface. β-phase CsPbI3 PSCs has a particularly high PCE of 18.4% with distinguished ambient stability because of PbI2·DMAI and CHI. The stability of β-CsPbI3 PSCs were greatly improved: it retained 92% of its initial PCE after 500 h illumination at the maximum power point, as shown in Fig. 5(a)[73].

class="figure_img" id="Figure5"/>
Download
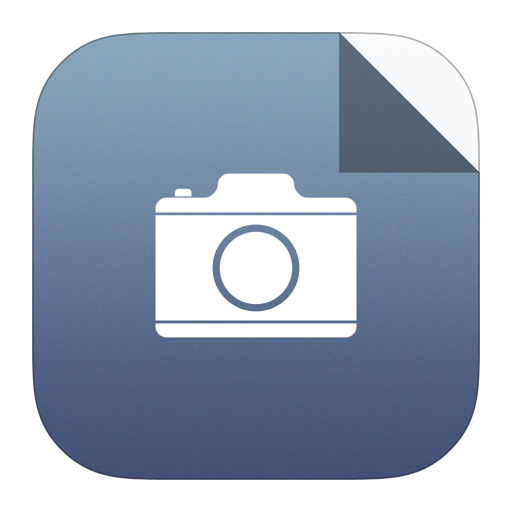
Larger image
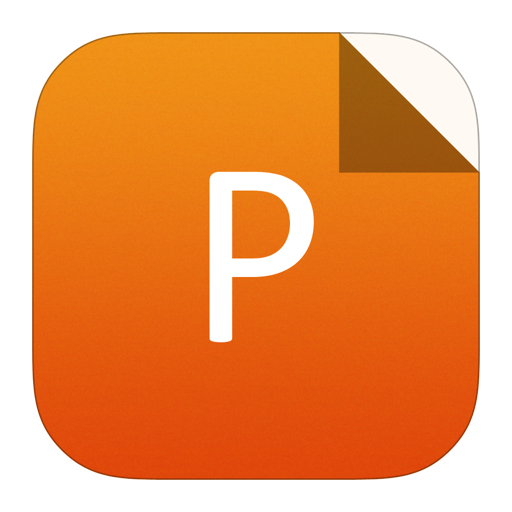
PowerPoint slide
Figure5.
(Color online) (a) Schematic illustration of CHI crack-filling interface engineering. Reproduced with permission[73]. Copyright 2019, Science Publishing Group. (b) Schematic diagram CsPbI3 crystal formation by using HI and H2O. Reproduced with permission[83]. Copyright 2018, American Chemical Society Publications. (c) Mechanism of STCG-CsPbI3 film formation by assistant of ADMA molecule. Reproduced with permission[84]. Copyright 2020, Wiley-VCH Publications. (d) The schematic illustration of HI and PEAI do on the CsPbI3. Reproduced with permission[43]. Copyright 2018, Nature Publishing Group.
The γ-phase CsPbI3 is the most stable black phase because of its lowest dissociation energy[82]. Hence, γ-phase CsPbI3 was systematically researched to boost its stability and performance. Hu et al. used HI to fabricate high-quality CsPbI3 film. After that, they introduced a small amount of H2O into precursor to induce a proton transfer process in CsPbI3 film, which could manipulate the grains size and improve stability of perovskite. When stored in ambient conditions, the optimal device showed no drop of its performance while the reference’s PCE was greatly degraded, as shown in Fig. 5(b)[83]. Then, Nazeeruddin et al. developed a soft template-controlled growth method to fabricate pinhole-free γ-phase CsPbI3 film, where (adamantan-1-yl)methanammonium (ADMA) acted as a template and ionized by HI. They pointed that ADMA absorbed on CsPbI3 surface and induced a steric effect to further increase the nucleation rate at the initial stage of CsPbI3 formation. Taking into account of controllable nucleation rate and excellent morphology, superior PCE of 16.04% was obtained with improved stability (drop only 10% after continuous light soaking and heating for 1000 h), as shown in Fig. 5(c)[84].
In our recent research, we reported the synergistic effect of HI and PEAI additives, where HI transferred to an intermediate (HPbI3+x) to fabricate distorted black phase-based CsPbI3 thin films and PEAI induced a steric effects to avoid phase transition. It is noteworthy that the best device maintained 92% of its initial PCE for 60 days storage in ambient (RH ~ 20%–30%, 25 °C), while the reference one degraded to 0.65% in the same condition for 8 days, as shown in Fig. 5(d)[43]. Then, HPbI3 was used to fabricate CsPbI3, assisted with anti-solvent hot substrate spin-coating method, and 15.91% PCE was obtained in a humidity environment (RH ~ 50%)[85]. Furthermore, we added a small amount of Br- (5%) to the HPbI3-CsPbI3 lattice to increase phase stability by suppressing bulk trap-assisted non-radiative recombination and relaxing lattice strain. The performance was boost up to a record PCE of 17.17% together with excellent stability[86]. Another work we reported was that 3 mol% Cl– was added to the γ-CsPbI3 film with a 16.07% PCE obtained. It not only showed the excepted increase crystalline dynamics for an excellent CsPbI3 morphology, but also improved crystalline orientation. The Cl-doping CsPbI3 showed high stability, for optimal device dropped only 0.45% of its initial PCE under continuous light soaking for 200 h. More important, non-encapsulated CsPbI3 PSCs with Cl– doping degraded only 6% when stored in RH ~ 30% for 60 days (the fresh one dropped to 85% of its initial PCE in the same conditions)[87]. We also introduced 2% Pb(SCN)2 into the DMAPbI3 and CsI precursor to control the morphology of the CsPbI3 film. In this case, a PCE of 17.04% with VOC of 1.09 V was obtained[88].
One of the notorious problems to limit CsPbI3 performance is the lower JSC compared with hybrid one. Thus, we have also developed several strategies to increase its JSC, such as harvesting short wavelength ultraviolet light (UV-light) or near-infrared (NIR) light, and designing device structure to capture light. First, we developed a downconversion nanoparticles (DCNPs) nitrogen-doped graphene quantum dots (N-GQDs) as an energy-down-shift to harvest the short wavelength (< 350 nm) UV-light. After combining it with HPbI3-formed γ-CsPbI3, the optimal device showed an improved short circuit current density (JSC) from 18.67 to 19.15 mA/cm2, with an increase of 2.57%. Meanwhile, its performance was greatly increased 3.15%, from 15.53% to 16.02%[89]. Furthermore, we developed a core-shell-structured upconversion nanoparticles (UCNPs) to capture the NIR light, making it possible to obtain 15.86% PCE (noted that the improvement of PCE is negligible), JSC = 19.17 mA/cm2[90]. We also investigated the influence of different haze glass substrates, and used the optimized one to fabricate CsPbI3 PSCs with 16.24% PCE. We found that the improvement came from scattering effect of FTO, refractive index and roughness of each layer[91].
5.3
Low dimension CsPbI3 based PSCs
Reducing dimension can further increase the stability of CsPbI3 PSCs because reducing materials dimension can lead to more symmetric crystal structure and show a smaller surface energy[92-96].
However, the poor solubility of CsX in the precursor solution would severely limited the thickness of CsPbI3 film and influence the light absorption. Chen et al. used HPbX3 and CsAc as new precursor to overcome the poor solubility of Cs+ precursor and fabricate α-CsPbX3 with optimal thickness. They introduced phenylethylammonium iodide (PEAI) to HPbX3 and CsAc system and further controlled the dimension of CsPbX3 from three dimension (3D) to two dimension (2D). Finally, a champion PCE of 12.4% in 2D CsPbI3 perovskite was obtained, and maintained 93% of its initial PCE in ambient for 40 days, as shown in Fig. 6(a)[97]. Similarly, our group used DMAPbI3 as a new precursor to fabricated γ-CsPbI3 perovskite, and then we introduced a judicious amount of PEAI into the DMAPbI3 contained precursor to convert γ-CsPbI3 PSCs into a 2D Ruddlesden–Popper (RP) structure (PEA)2(Cs)n?1PbnI3n+1 perovskite. And the optimal 2D RP PSCs with highest PCE of 13.65%, showed a similar charge extraction and carrier lifetime compared with the 3D samples. The thermostability was tested in N2 filled glovebox. The superior 2D RP PSCs kept 88% of its initial PCE when stored at 80 °C for 15 days, while the reference 3D γ-CsPbI3 degraded to 69%. Besides, 2D RP PSCs maintained its black color whereas 3D γ-CsPbI3 appeared yellow phase in RH ~ 30% for 12 days, as shown in Fig. 6(b)[98]. In addition to PEAI, Chen et al. first introduced a novel dual ammonium cation piperazine-1,4-diium (PZD+) to generate 2D RP CsPbI3. It’s noted that one of the ammonium groups coordinated with [PbI6]4– octahedral, and another one interacted with I– to balance charge. Finally, the optimized device achieved a PCE of 9.39% and maintained its performance without decomposition under heating at 100 °C for 24 h[99].

class="figure_img" id="Figure6"/>
Download
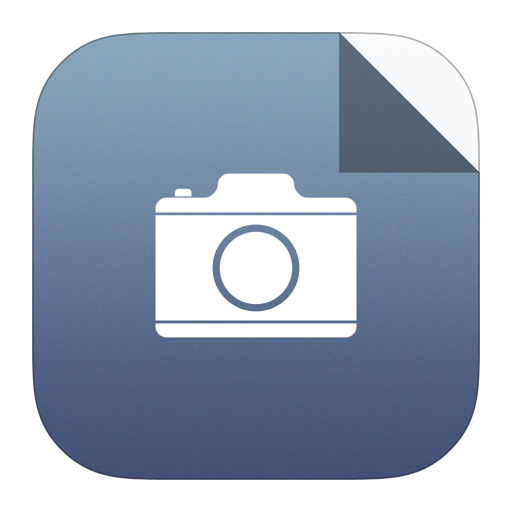
Larger image
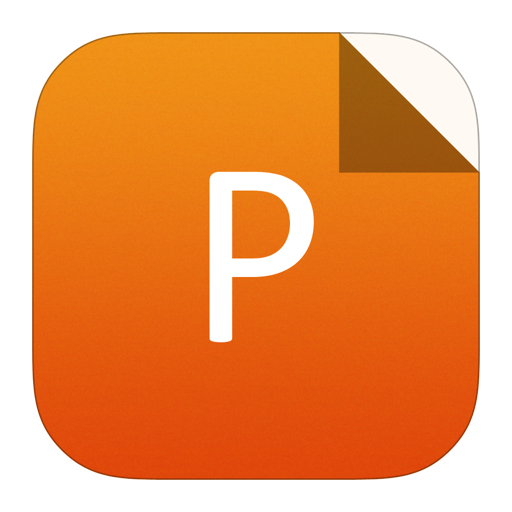
PowerPoint slide
Figure6.
(Color online) (a) The structure and decomposition energies of different n values PEA2Csn-1PbnX3n+1. Reproduced with permission[97]. Copyright 2018, Elsevier Inc Publications. (b) The controllable n values and structures of PEA2Csn-1PbnX3n+1. Reproduced with permission[98]. Copyright 2019, Wiley-VCH Publications. (c) Schematic illustration the fabrication process of shell ligand, HPbI3, H2PbI4 and in-suit assembled of them. Reproduced with permission[101]. Copyright 2019, Wiley-VCH Publications.
Pradhan et al. fabricated stable CsPbI3 nanocrystals (NCs) with superior stability by using a higher temperature (260 °C) than usual (160 °C), and adding olelyamine (OLA) and HI respectively in the reaction process (noted that only OLA or HI are less efficient). Taking the NMR analyzation into account, they found that higher temperature helped the OLA+ ligands to occupy the Cs+ position on the surface and further stabilized its structure[100]. To solve adverse shell ligands and unstable core lattices in nanocrystals, Choi et al. developed a rational core-shell design method in CsPbI3 NCs. They used a novel ligand named 4-fluorophenethylammonium iodide (FPEAI) to enhance the binding force between the ligand and CsPbI3 NCs and efficient charge coupling between NCs, to increase charge extraction. Besides, H2PbI4, which was synthesized with excessive HI, was used to assist doping Mn2+ ion into perovskite lattices and to further raise the performance of CsPbI3 NCs to 13.4% with superb stability (maintained 92% of its PCE in air for 500 h), as shown in Fig. 6(c)[101].
6.
Prospects and outlook
Although lots of advanced works about HI hydrolysis-derived intermediate have been done to boost PCE of CsPbI3 PSCs, its PCE still far behind the hybrid ones. Therefore, we need to analyze the urgent problems that remain and develop corresponding strategies to improve the performance of CsPbI3 perovskites.
Increasing the light absorption. As we show in Table 1, though the performance of CsPbI3 PSCs has reach 19.03%[74] , the JSC (about 20 mA/cm2) is still lower than the hybrid one. This maybe cause by current loss and insufficient utilization of light, resulting from the relatively large bandgap (compared with hybrid perovskite films). Developing high efficiency DCNPs/UCNPs, to capture UV-light or NIR could be an useful solution[90].
Doping X-site halide to improve CsPbI3 PSCs stability. The unstable nature of CsPbI3 is an unsuitable tolerance factor (t), as we mentioned earlier. Partially replacing I– (2.2 ?) with smaller radius halides, e.g., Cl– (1.87 ?) and Br– (1.96 ?) or pseudohalide SCN– (2.15 ?), could enlarge t value to reach ideal one (0.9–1)[32].
Ligand assists to stabilize CsPbI3 crystal structure. Introducing ligand (e.g., OTG[80], poly-vinylpyrrolidone (PVP)[102], ADMA[84], PEAI[79], and DETA[67]) into a perovskite precursor and inducing a steric hindrance to further stable CsPbI3 crystal structure is a useful way to boost its stability. Therefore, it is promising to develop and design new ligands.
Increasing the carrier transport for low dimension perovskite. Low dimension CsPbI3 tends to increase surface-area-to-volume ratio and raise the Gibbs free energy to make the structure more stable[103]. It is noted that though low dimension CsPbI3 can keep CsPbI3 structure more stable, the PCE of low dimension CsPbI3 is still far below the 3D CsPbI3. Hence, we should pay more attention to increase the carrier transport for low dimension CsPbI3.
Avoiding the disadvantage of intermediate. Liu et al. claimed that intermediates had negative influence during low dimension perovskite deposition process because they slowed down intercalation of ions and increased nucleation barrier, and further caused the byproduct formation[104]. Although there are no negative effects on HI hydrolysis-derived intermediate so far, we should be more vigilant against HI hydrolysis-derived intermediate.
In conclusion, CsPbI3 perovskite, particularly the metastable phases (β- and γ-Phase CsPbI3), is a promising material to replace the unstable hybrid perovskite. Besides, CsPbI3 PSCs with suitable bandgap make it more suitable to apply in tandem solar cells and commercialization. Based on these advantages, we conclude that CsPbI3 PSCs maybe the mainstream research direction in the near future, and we should adopt a positive attitude to it.
Acknowledgements
This work was funded by the National Natural Science Foundation of China (51902148, 61704099 and 51801088), the Fundamental Research Funds for the Central Universities (lzujbky-2020-61, lzujbky-2019-88 and lzujbky-2020-kb06), and the Special Funding for Open and Shared Large-Scale Instruments and Equipments of Lanzhou University (LZU-GXJJ-2019C023 and LZU-GXJJ-2019C019).