1.
Introduction
Organometal halide perovskites have attracted great attention as emitting materials of high efficient light-emitting diodes (LEDs) for their unique advantages such as high color purity, tunable bandgap and facial solution processibility[1-6]. Substantial progresses have been made in the past few years through advanced materials development, surface defects passivation and device structure optimization, resulting in booming external quantum efficiency (EQE) exceeding 20%[7-9]. However, organometal halide perovskite LEDs (Pe-LEDs) are still greatly hampered by the following challenges: the toxicity of Pb and the thermal instability caused by the organic composition[10-13]. Therefore, all-inorganic lead-free halide perovskite is more favorable for practical applications, which is environment friendly and has better thermal stability[11, 12, 14, 15].
Despite most of the Pe-LEDs are fabricated via solution processing, vacuum-based deposition (VBD) is more favorable for the fabrication of high performance LEDs. Because of the controllable environment of vacuum preparation, devices fabricated by VBD are highly reproducible and reliable[16-20]. Besides, VBD has also been demonstrated as the most successful approach for commercial application. Thus, Pe-LEDs based on VBD method could be perfectly compatible with the current industry manufacturing technique of organic LED (OLED).
Recently, a set of high performance all-inorganic perovskites CsCu2I3 and Cs3Cu2I5 have been reported[11, 12]. By replacing the organic group with Cs element, their thermal and air stability are greatly enhanced. The significant weight loss temperature of CsCu2I3 and Cs3Cu2I5 is up to 600 °C, and no notable PLQY decrease could be observed even after months storage directly in the ambient condition[14]. Besides, the employing of non-toxic and abundant copper element in the B-site makes them more suitable for commercial production, considering the necessity of environmentally sustainable development.
Further research confirmed that the electron–phonon interaction is so strong in these materials that the excited electrons and holes would cause elastic distortions in the lattice surrounding them as self-trapped excitons (STEs)[15, 21, 22]. STE features greatly broad emission spectra, which would benefit white luminescence applications[23-25]. However, related reports of the STE electroluminescence are still quite rare. As far as we know, professor HOSON has realized LED devices with Cs3Cu2I5 as the emitting layer for the first time, promoting us for the further research of the STE electroluminescence[12, 26].
Herein, CsCu2I3 powder was successfully synthesized with a high photoluminescence quantum yield (PLQY) of nearly 20%. Bright yellow emission could be observed under UV light excitation, with a emission peak located at 560 nm and a quite broad full width at half maximum (FWHM) of nearly 180 nm due to STE emission[26, 27]. By taking advantage of its extremely thermal stability, we successfully fabricated uniform CsCu2I3 film via VBD, which was further confirmed by the scanning electron microscope (SEM) and X-ray diffraction (XRD) measurements. Finally, all-vacuum deposited Pe-LED with CsCu2I3 as the emitting layer was successfully fabricated, with brightness of 10 cd/m2 and EQE of 0.02%. To the best of our knowledge, this is the first all-vacuum deposited CsCu2I3 Pe-LED with STE emission, which provides a new avenue for high quality Pe-LEDs.
2.
Experiment
2.1
Materials
All chemicals were used without further purifications: CsI (cesium iodide, 99%, Aladdin Reagent Ltd); CuI (cuprous iodide, 99%, Aladdin Reagent Ltd); DMSO (dimethyl sulfoxide, AR99%, Aladdin Reagent Ltd); LiF (lithium fluoride, 99.99%, Aladdin Reagent Ltd); TPBi (Xi’an Polymer Light Technology Corp); Aluminum particles (99.99%, Xi’an Polymer Light Technology Corp).
2.2
Synthesis of CsCu2I3 powder
CsCu2I3 powder was synthesized using a simple and efficient one-step reaction. In the reaction, 1.45 g CsI and 2.11 g CuI were added in 9 mL DMSO and stirred for 2 h at 70 °C for complete dissolution. Then, the solution was heated to 90 °C to evaporate the solvent and the resulting precipitate was CsCu2I3 powder that we needed.
2.3
LED device fabrication
Etched indium tin oxide (ITO) glass substrates were cleaned sequentially with detergent, acetone, isopropyl alcohol and ethanol by sonication for 20 min of each step, then dried with nitrogen. 20 nm Li doped NiOx was deposited on the ITO glasses by magnetron sputtering, with a radio frequency power of 200 W for 2 min. During the NiOx deposition, the substrates were held at 200 °C with a Ar2/O2 flow ratio of 99, and the vacuum was kept lower than 10–3 Pa. Afterwards, the synthesized CsCu2I3 powder was deposited on the room-temperature NiOx by vacuum-based deposition without further annealing. Next, a layer of 20 nm TPBi and patterned LiF (1 nm)/Al (80 nm) electrodes were deposited by thermal evaporation to complete the device fabrication. The deposition rates of CsCu2I3, TPBi, LiF and Al were kept at 0.2, 0.1, 0.02, and 0.2 nm/s, respectively. The rates were precisely monitored by calibrated quartz crystal. The vacuum was kept under 10-4 Pa during the whole deposition process.
2.4
Materials and devices characterization
The XRD data was accumulated using a Philips X'Pert Pro diffractometer with Cu Kα radiation (λ = 1.54 ?). The photoluminescence (PL) and photoluminescence exciation (PLE) spectra of the CsCu2I3 film were measured using an Edinburgh FLS980 spectrofluorometer. The absolute PLQY was further determined with an integrated sphere. The morphology of CsCu2I3 film was characterized by FEI Nova Nano field-emission SEM. Keithley 2400 Sourcemeter and Photo Research Spectra Scan PR655 were programmed and used to record the J?V?L curves, EQE, and CRI color coordinates of the operating Pe-LEDs.
3.
Results and discussion
In this work, we used a simple one-step way to successfully synthesize CsCu2I3 powder. Fig. 1(a) shows optical images of the synthesized CsCu2I3 powder under natural light and excited by ultraviolet light. The synthesized white CsCu2I3 powder under natural light emits bright yellow light under ultraviolet excitation. Then the precise PLQY of the CsCu2I3 powder is furthered confirmed as shown in Fig. 1(b). After calibration calculation, the absolute PLQY of the CsCu2I3 powder is 21.4%.

class="figure_img" id="Figure1"/>
Download
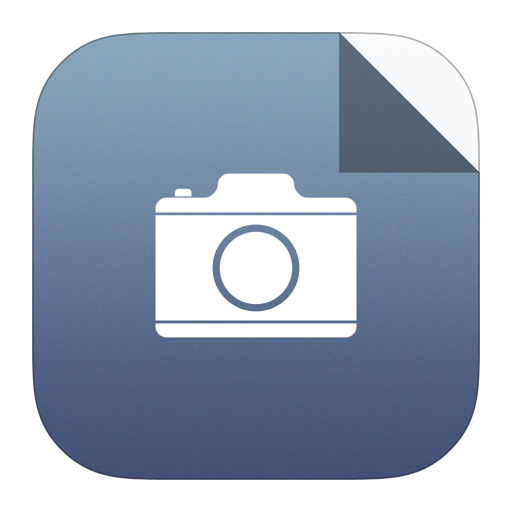
Larger image
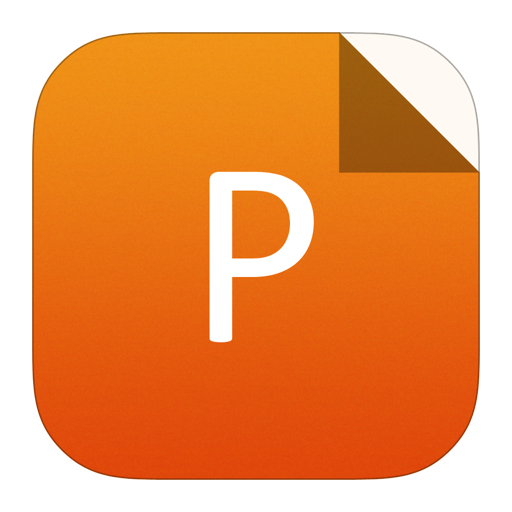
PowerPoint slide
Figure1.
(Color online) (a) Optical images of synthesized CsCu2I3 powder under natural light and excited by ultraviolet light. (b) PLQY of the CsCu2I3 powder.
The synthesized CsCu2I3 powder shows excellent air and thermal stability, whose decomposition temperature is as high as 600 °C, as confirmed in the early work[14]. Therefore, it is quite favorable to be deposited by VBD methods. The uniform and stable CsCu2I3 film was successfully fabricated by thermal evaporation of the synthesized powder. The crystal structure of CsCu2I3 film is further confirmed by XRD in Fig. 2(a), which is consistent with the simulated CsCu2I3 XRD pattern and CsCu2I3 powder and accords with the standard orthorhombic CsCu2I3 XRD pattern (JCPDS #45-0076). The CsCu2I3 crystal structure is shown in Fig. 2(b). Each [CuI4]3– tetrahedron is connected by three common edges, gradually forming a tetrahedral double-chain structure parallel to c axis. We further characterized the optical properties of CsCu2I3 film. The PLE and PL spectra of the CsCu2I3 film are shown in Fig. 2(c). The PL spectrum possesses a PL peak located at about 560 nm and a broad FWHM of 180 nm. As shown in the inset of Fig. 2(c), the VBD CsCu2I3 film also shows a bright yellow emission under ultraviolet excitation with the same color of the CsCu2I3 powder in Fig. 1. The broadband luminescence of CsCu2I3 is attributed to self-trapped excitons recombination. As confirmed in the early works, the strong electron-phonon interactions cause the distortion of lattice, resulting in large stokes shift and wide FWHM[13, 15, 16]. The photo physical process for CsCu2I3 can be described using a configuration coordinate diagram (Fig. 2(d)). The photo physical process for CsCu2I3 can be described using a configuration coordinate diagram (Fig. 2(d)). As confirmed by the low temperature of Raman test in the early works, the phonon mode vibrational intensity (corresponding to the I–Cu–I vibration) exhibits an obvious reduction at lower temperature, which demonstrates the strong electron-phonon couple effect in CsCu2I3[17]. The strong electron-phonon interaction causes the elastic distortion of the lattice and ultrafast excited state structural reorganization. Once the electrons are excited by incident light, they will be quick self-trapped leading to the self-trapped excitons. The subsequent radiative decay of the STEs generates a very bright broadband emission[26].

class="figure_img" id="Figure2"/>
Download
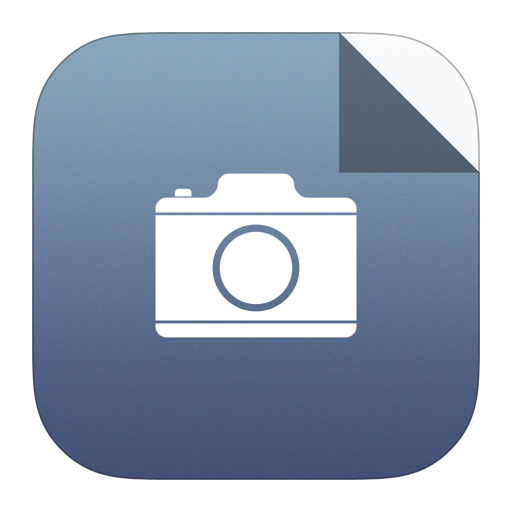
Larger image
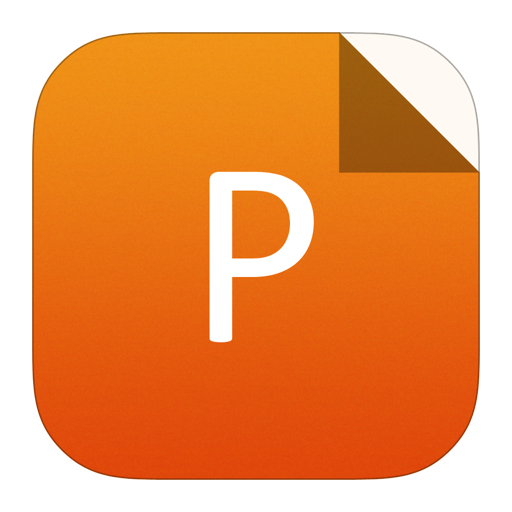
PowerPoint slide
Figure2.
(Color online) (a) XRD patterns of the CsCu2I3 film and powder. (b) Schematic of CsCu2I3 crystal structure. (c) PLE and PL spectra of the CsCu2I3 film. (d) Schematic of the energy level structure of STE.
As CsCu2I3 is a reported environmentally friendly material with excellent PLQY and thermal stability[14], we continued to explore its application in LED devices. Here, the optimized structure of ITO/NiOx/CsCu2I3/TPBi/LiF/Al was employed as shown in Fig. 3(a). Different from the traditional solution method, the CsCu2I3 layer was prepared by VBD, followed by the function layers without the break of vacuum. Vacuum-based deposition has been proved to be suitable for depositing all inorganic perovskites due to the free of solubility limitation and coating onto rough substrates such as the textured silicon surface[18, 28]. What’s more, with the controllable vacuum preparation environment, VBD leads to highly reproducible and reliable results which are compulsory for manufacturing and further equipment improvement. In the device preparation, 20 nm Li-doped NiOx as the hole transport layer was first deposited on the clean and etched ITO glasses by magnetron sputtering. Then the CsCu2I3 powder was deposited on the NiOx by VBD. As shown in Fig. 3(b), CsCu2I3 source was placed under the substrate with a distance of 40 cm. The morphology of deposited CsCu2I3 film was characterized using a SEM and shown in Fig. 3(c). The deposited CsCu2I3 film with continuous coverage and small grains is beneficial to the fabrication of Pe-LED. Afterwards, a layer of 20 nm TPBi was prepared as the electron transport layer. Finally the patterned LiF (1 nm)/Al (80 nm) electrodes were deposited by thermal evaporation to complete the device fabrication. The energy level diagram of CsCu2I3 Pe-LED is also shown in Fig. 3(d). Energy levels of organic semiconductor layers and CsCu2I3 are taken from literatures[29, 30]. As shown, electrons and holes could be effectively blocked by the NiOx and TPBi respectively, which could ensure the exciton recombination region confined in the CsCu2I3 emitting layer. Charge carrier injection barrier between the transport layer and emitting layer is very small, which is easy to overcome by applying a very small voltage. This could be further demonstrated by the relatively lower device turn-on voltage.

class="figure_img" id="Figure3"/>
Download
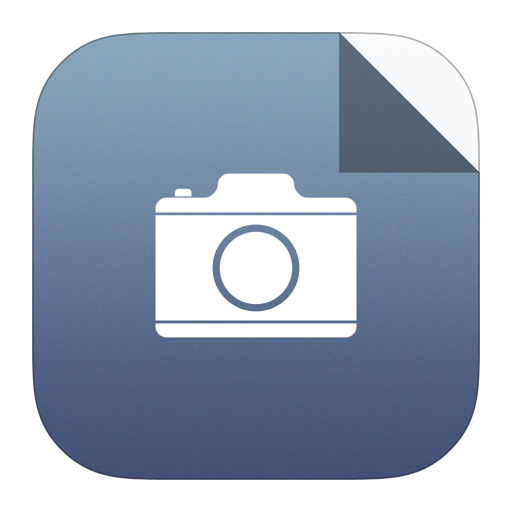
Larger image
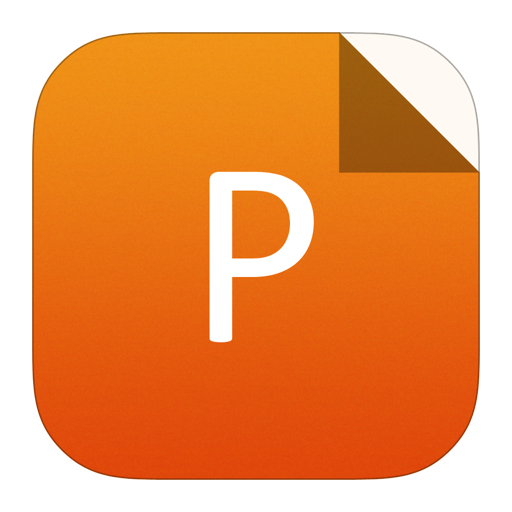
PowerPoint slide
Figure3.
(Color online) (a) Structure of CsCu2I3 Pe-LED. (b) Schematic of VBD for CsCu2I3 film. (c) SEM image of CsCu2I3 film. (d) Energy level diagram of CsCu2I3 device.
The current density–voltage–luminance (J–V–L) characteristics and external quantum efficiency (EQE) versus luminance are described in Figs. 4(a) and 4(b). The resulting device shows a relatively low turn-on voltage of 2.9 V at the luminance of 1 cd/m2. It exhibits the maximum luminance of 10 cd/m2 and the best EQE is 0.02% at 1 cd/m2. Fig. 4(c) shows an image of the operating Pe-LEDs and the CIE color coordinates. It emits bright yellow light with a greatly broad FWHM just same as the PL results, which is attribute to the STE emission. The measured CIE color coordinate is (0.489, 0.485) as shown in Fig. 4(c). It is believed that the successful realization of the STE electroluminescence based on CsCu2I3 would make a step further in the aspect of wide spectrum electroluminescence. And much more continuous works are expected to further improve device brightness and luminescence.

class="figure_img" id="Figure4"/>
Download
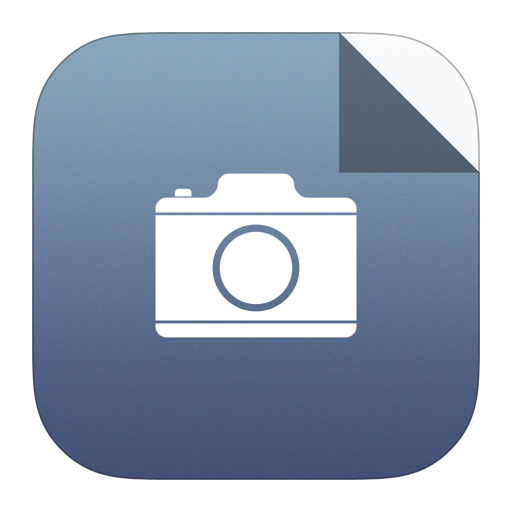
Larger image
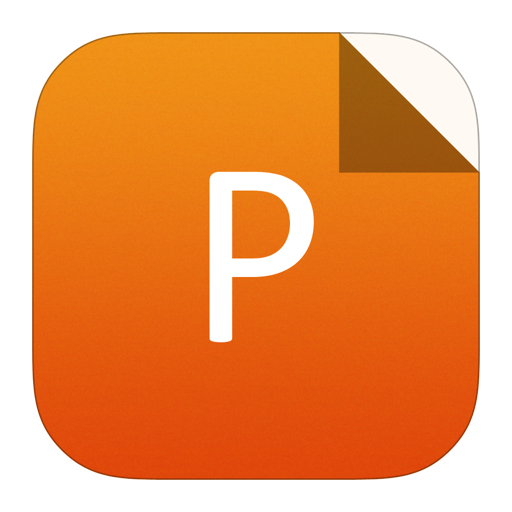
PowerPoint slide
Figure4.
(Color online) (a) Current density–voltage (J–V) and luminance–voltage (L–V) curves of the CsCu2I3 Pe-LED. (b) EQE curve of the CsCu2I3 Pe-LED. (c) The CIE color coordinate of the CsCu2I3 PeLED.
4.
Conclusion
In summary, we synthesized the CsCu2I3 powder with PLQY exceeding 20% by a simple method. By taking advantage of its excellent thermal stability, the uniform and stable CsCu2I3 film was successfully fabricated by the direct evaporation of CsCu2I3 powder through VBD technology. We further characterized the VBD CsCu2I3 film by XRD and the pure phase of was determined. For the CsCu2I3 film prepared by VBD, the morphology was observed with flat surface and dense grains. The CsCu2I3 Pe-LED emitted bright yellow light with a quite broad FWHM attributed to the STE emission. It showed the maximum luminance of 10 cd/m2 and the best EQE is 0.02%. As far as we know, it is the first time of the successful realization of the STE electroluminescence based on CsCu2I3 through the advanced VBD technology. Despite its relatively low brightness and efficiency at present, the great improvement of the device performance could be sincerely expected with the further optimization of the device structure. We believe this work would provide a new avenue for high quality Pe-LED, and promote the further exploration of its working mechanism.
Acknowledgments
This work was supported by the National Key R&D Program of China (2016YFB070700702), the National Natural Science Foundation of China (51761145048), the Fundamental Research Funds for the Central Universities (HUST: 2019421JYCXJJ004) and the China Postdoctoral Science Foundation Grant (2019M662624). The authors thank the Analytical and Testing Center of HUST.