1.
Introduction
Quantum photonic technologies requires high-performance light sources that emit quantum state of photons on-demand. Semiconductor QDs have been long believed as a very promising system for such high-performance quantum light sources because it can emit one single-photon or one entangled photon pair at a time under external optical/electrical excitations[1–5]. Nevertheless, the photon collection efficiency of QDs in a bulk semiconductor is typically < 1% because of the total internal reflection associated with the large refractive index contrast between the semiconductor and air, and therefore greatly limits the real application of such sources in experiments of optical quantum computation/simulation[6–8].
Embedding single QDs in photonic nanostructures has been widely explored as a very effective strategy for improving the performance of quantum light sources based on QDs. Last decades have witnessed the tremendous developments on micro-cavities with high quality (Q) factors and small mode volumes in a variety of geometries, e.g., micro-disk[9–12], micro-pillars[13–18] and photonic crystal cavities[19–25] etc. The fusion of QDs and micro-cavities in semiconductors has been delivering enhanced light-matter interactions at single-photon level, which enables the realization of Purcell-enhanced high-performance single-photon sources, vacuum Rabi splitting and few-photon nonlinearities via cavity quantum electrodynamics (QED) effects. However, due to the random nature of the self-assembly during the epitaxial growth, the QDs inevitably exhibit a pronounced inhomogeneous broadening over a large spectral range (typically 50 nm). Therefore, achieving spectral resonances between the single quantum emitters and the high-quality cavity modes turns to be very challenging without post-tuning techniques. Despite the tremendous successes have been realized by either changing the QD emission wavelength via tuning the external physical parameters (e.g., temperature[26], electric field[27, 28] and strain[29–31]) or tuning cavity modes with gas depositions[32], it is still not yet feasible to independently tune multiple QDs or cavity modes in the same semiconductor chip. Consequently, developing broadband photonic nanostructures that is insensitive to the QD spectral inhomogeneity is highly desirable for readily achieving high-performance quantum light sources, especially when simultaneous coupling of multiple QDs to photonic nanostructure is involved in many-body quantum experiments.
In this review article, we summarize recently developed broadband photonic nanostructures that are designed for coupling to semiconductor QDs and their applications in high-quality non-classic light generations. The design principle, fabrication process and device performances of the photonic nanowires, photonic crystal waveguides (PCWs), micro-lens and circular Bragg gratings (CBGs) will be discussed in the following.
2.
Waveguide-based broadband photonic structures
2.1
Photonic nanowires
Nanowires were proposed to working as a waveguide because of their considerably enhanced light extraction efficiency along the axial direction. As illustrated in Figs. 1(a) and 1(b), a nanowire with the optimized diameter can efficiently funnel the spontaneous emission from QDs into the fundamental HE11 mode and suppress the coupling to other radiative modes[33, 34]. A bottom mirror (e.g. a dielectric/metal mirror)[35] is used to reflect the downwards emission back into the guided mode. The QD-to-mirror distance should be carefully designed to obtain an antinode in the electric field at the position of QDs. In order to efficiently collect the emitted photons with an objective or fiber, a conical tapered tip[36] is optimized so that the mode will be adiabatically expanded outside the nanowire. Theoretically, high beta factors that quantify the fraction of the emission coupling to a targeted mode above 0.9[34, 37] are achievable over a remarkably broad spectral range of 70 nm at λ = 950 nm[33]. The extraction efficiency for the photons from nanowires to an objective with a numerical aperture NA = 0.85 could go as high as around 95%[33]. Additionally, the Gaussian far-field emission pattern of the propagating mode enables the possibility of realizing high coupling efficiency to an optical fiber[38–40]. Thus QD-in-nanowire structure is an appealing candidate for the realization of bright quantum light sources.

class="figure_img" id="Figure1"/>
Download
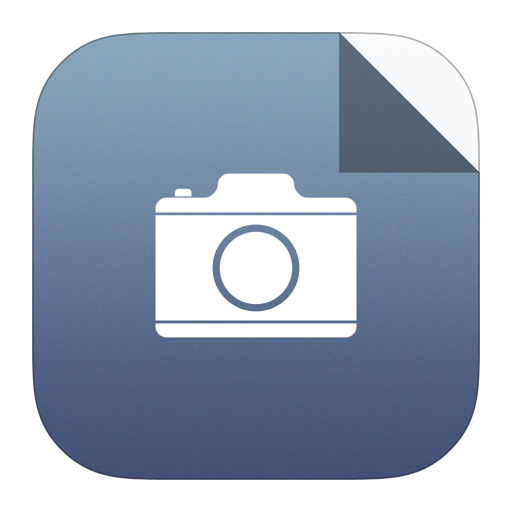
Larger image
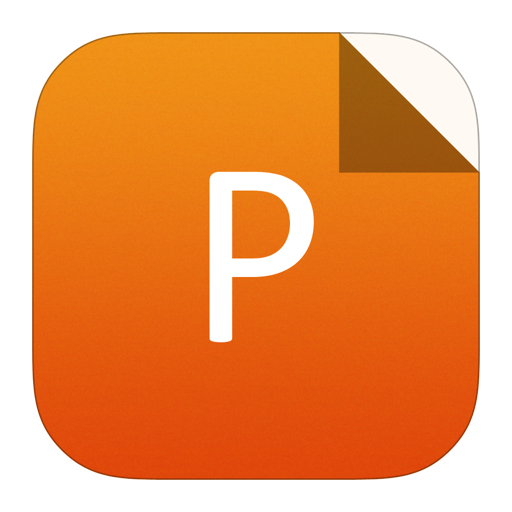
PowerPoint slide
Figure1.
(Color online) (a–b) Scanning electron microscopy (SEM) image (a) of a top–down tapered GaAs nanowire waveguide with an embedded InAs QD, together with the intensity profile for a 2D-cut along the nanowire growth axis by FDTD simulation (b). (c–d) SEM image (c) of a top–down GaAs photonic trumpet with an embedded InAs QD, together with the intensity profile for a 2D-cut along the nanowire growth axis by FDTD simulation (d). (e) SEM image of a bottom–up tapered InP nanowire waveguide containing a single InAsP QD[51], reprinted with permission, Copyright 2012, Springer Nature.
Top-down and bottom-up methods are mostly employed to fabricate QD-in-nanowires. The top-down approach is based on epitaxial growth of QD materials combined with plasma dry etching technique[41] while the bottom-up approach can directly form nanowires by vapor-liquid-solid (VLS)[42, 43] and selective-area epitaxy (SAE)[44] growth modes. Compared to bottom-up growth, the nanowire geometry (e.g. the tapered tip and the bottom mirror) can be engineered in a large degree of freedom in top-down fabrication, making it possible to achieve both nanowires with needle taper and photonic trumpet with inversed conical taper (Figs. 1(c) and 1(d)). The latter shows robustness against variations in the taper angle thus offers a reliable way to improve the photon collection. Highly-efficient single photon sources (SPSs) were experimentally demonstrated firstly in nanowire antenna with an extraction efficiency of 72%[45], and later in photonic trumpet with an extraction efficiency of 75% and an external coupling efficiency to a Gaussian beam as high as 0.58[46]. A monolithic optical-fiber-coupled SPS was also achieved via directly transferring a nanowire trumpet onto the core of a fiber pigtail, showing an external collection efficiency of QD emission of 5.8%[47]. Additionally, the QD emitter coupling to mechanical modes of the nanowire trumpet structure[48, 49] offers a new degree of freedom with potential application in exciton-phonon interaction, precision quantum sensing. However, the yield of devices in the top-down approach has been hindered by the randomness in the locations of self-assembled QDs. From this perspective, the bottom-up growth method offers a straightforward and deterministic fabrication approach with more choices in the material compositions. As shown in Fig. 1(e), the most popular configuration consists of QD positioned on the NW axis, forming an axial heterostructure. In general, Ⅲ–Ⅴ nanowires prefer the [111] crystalline orientation[50]. One of the most widely studied material systems is InAsP QDs in InP nanowires. A bottom-up-grown nanowire with a fine taper toward the tip (tapering angle approximately 2°) and a bottom gold mirror has been reported by Reimer et al. in 2012, yielding an overall efficiency of 42%[51]. It has also been successfully demonstrated that the grown antenna can be used to obtain Gaussian far-field emission pattern and high coupling efficiency of 93% to an optical fiber[39].
High symmetry QDs fabricated in the [111] growth direction should exhibit vanishing FSS and, therefore, could emit entangled photon pairs via the biexciton-exciton cascaded radiative process[52, 53]. A QD entangle photon source with an extraction efficiency of 18 ± 3%, an entanglement fidelity of 0.859 ± 0.006 and concurrence of 0.80 ± 0.02 has been realized by using a position-controlled QD in a broadband nanowire antenna[54]. Furthermore, QD emission tuning is also an important issue in QD-in-nanowires, as it is still challenging to obtain QD SPSs with specific wavelengths. Strain tuning of QD emission has been demonstrated with bottom-up nanowire by transferring the nanowires onto an piezoelectric crystal[55] or by an amorphous capping shell[56].
Recently, a large static strain tuning of up to 25 meV for a QD embedded in a nanowire trumpet stressed by nanomanipulators[56] has been reported. Despite these impressive improvements on the photon extraction efficiency, the fiber-coupling efficiency and the emission wavelength tuning, quantum light sources based on nanowire with very high indistinguishability (> 99%) remains elusive. Spectral diffusion[57, 58] induced by fluctuating charges and defects around the QDs inevitably introduce extra dephasing process, resulting in reductions of the photon indistinguishability. Until now, the highest two-photon interference visibility achieved is 0.83 at 300 mK by excitation above the InP bandgap with high intensity to fill the charge traps[59]. Further reaching the Fourier-transform-limited photons may rely on surface passivation techniques[60, 61], resonant excitation techniques[2, 62-64] or accelerating the quantum dot emission via nanowire with the Purcell effect[40].
2.2
Photonic crystal waveguides
Instead of efficiently coupling the quantum light from semiconductor material to the free space optics, PCW serves as a very effective tool of routing the single-photons in the semiconductor chips due to its planar geometry.
The PCs discussed in this work refer to thin semiconductor membranes with periodically etched air holes which create the photonic bandgap for the photons travelling in the membranes (below the light line). The vertical confinement of photons is governed by the total internal reflection between the semiconductor and air interface. The band structure of an infinite PCW is shown in Fig. 2(a). By leaving a line of the air holes, a waveguide mode can be created in the photonic band gap, as illustrated in Fig. 2(b). The solid and dashed lines corresponds to the first and second waveguide modes within the TE-like band gap. The gray shaded area above the light line represents the continuum of radiation modes. A SEM image of a representative PCW device is shown in Fig. 2(c). Interestingly, the density of the optical state of the waveguide modes is greatly enhanced at the band edge, resulting in a pronounced Purcell factor for the QDs that couples to the waveguide modes[65]. Similar to the photonic nanowires, the couplings of QDs to the radiation modes are greatly suppressed due to the 2D photonic bandgap. By simultaneous enhancing the coupling to the PCW mode and suppressing the leakages to the radiation modes, the spontaneous emission factor (beta factor) of QDs to the targeted propagating mode could be reaching to near-unity.

class="figure_img" id="Figure2"/>
Download
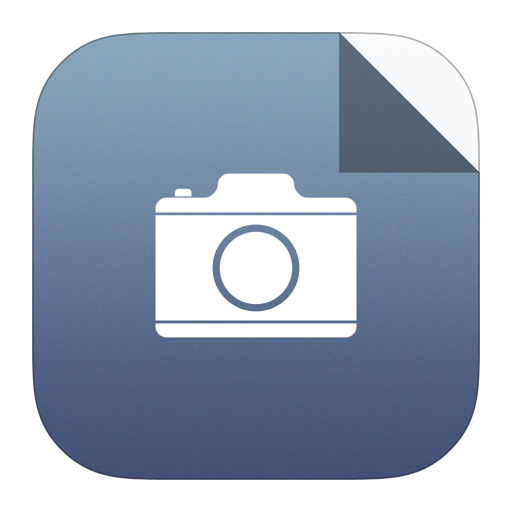
Larger image
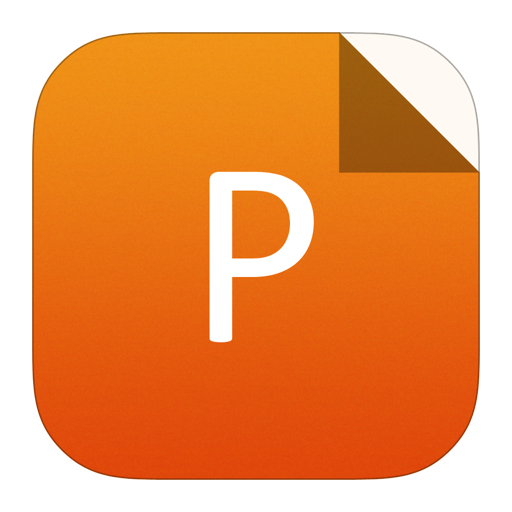
PowerPoint slide
Figure2.
(Color online) (a) Illustration of a finite PCW with a single QD embedded. (b) The band structure and waveguide modes of PCWs. (c) SEM picture of a PCW. (d) Decay dynamics for QDs that couple and uncouple to the PCWs[68], reprinted with permission, Copyright 2014, American Physical Society.
The first unequivocal experimental demonstration of highly efficient broadband coupling of InAs QDs to GaAs PCWs was shown by Hansen et al.[66], in which the decay rates of QDs that couple and uncouple to the waveguide modes were measured with a confocal micro-PL setup. A beta factor up to 0.89 was extracted from the measured decay rates. Measurements from QDs emitting at different wavelengths in the waveguides reveal a large operation bandwidth of ~20 nm.
Despite the impressive beta factors demonstrated in this work, the collection of photons coupling to the propagating waveguide modes was rather inefficient in a confocal micro-PL setup. Only a small portion of photons that couples to the waveguide can be collected by the objective above the waveguides due to some out-of-plane scattering, which results in a very low photon count rates in single-photon detectors. Therefore, it is highly desirable to measure decay rates of QDs that coupled to the PCWs from the end of the waveguides. In the measurements by Laucht et al.[67], the photon count rate from the edge of the waveguides is 55 times higher than that measured from the top, further highlighting the efficient coupling of QDs to the propagating modes. With further optimization of the side collection from waveguides[68], e.g., implementing grating coupler and taper structures, a near-unity (0.98) beta factor was experimentally achieved, approaching to the theoretical limit as shown in Fig. 2(d).
In addition, QDs with beta > 0.9 were measured across the large spectral range over 20 nm. Although the PCWs funnel the single-photons emitted by QDs to the targeted propagating waveguide modes, the photon count rates in the single-photon detectors is still far from satisfactory. This is mostly due to the mode mismatch between the modes with nanoscale cross-section in the PCWs and the single-mode fiber with a core diameter of a few microns. Such a technical challenge was recently handled by extracting photons in the PCWs via specially prepared tapered fibers. An optical fiber was tapered down to a few hundred nanometers to phase match with the waveguide modes. By deliberately making local minimal height in the tapered fiber and accurately launching to the waveguides regime, ~80% of photons in the waveguide was guided to the fiber with a single-photon count rate up to 4.38 MHz obtained in the single photon detector, corresponding to a source efficiency more than 10%[69]. The single-photon count rates obtained in the fiber-coupled PCWs devices are already comparable to the sources based on cavities. With the advantage of broad operation bandwidth, the PCWs could serve as a reliable source for photonic quantum simulations/computation by further improving the coherent properties of the photons, for instance, exploiting resonant excitations and charge stabilization techniques.
3.
Lens-based broadband photonic structures
3.1
Solid immersion lens
The most straightforward way to increase the light extraction is to use a lens to guide the emitted photons to the collection optics. For semiconductor QDs, solid immersion lens (SIL) made of high refractive index materials represents a viable option. Zirconia lens is widely employed in enhancing the photon count rate. But compared with the GaAs material, the refractive index of Zirconia is relatively small, leading to an insufficient extraction of QDs photons. Gallium phosphide (GaP) semi-sphere lens (with refractive coefficient similar to GaAs) is recently exploited by Chen and co-workers[70]. It is worth to note that the GaP lens used in their experiment is large in diameter (in the order of millimeter). To prevent large air gaps between the QDs and the lens, they stick the QD-containing membrane to the lens instead of placing the lens on the GaAs substrate, as the thin/flexible membrane could adapt itself to the ‘wavy’ bottom surface of GaP lens as is shown in Fig. 3(a). The back side of the membrane is sputtered with a silver layer as a mirror. They demonstrate an extraction efficiency of 65% for single photons and 0.37 pair per pulse for entangled photons.

class="figure_img" id="Figure3"/>
Download
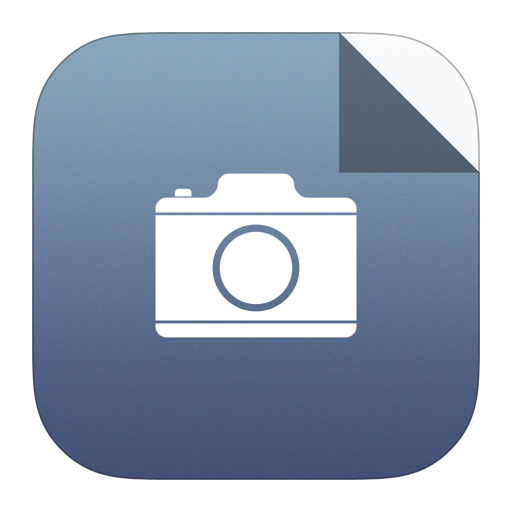
Larger image
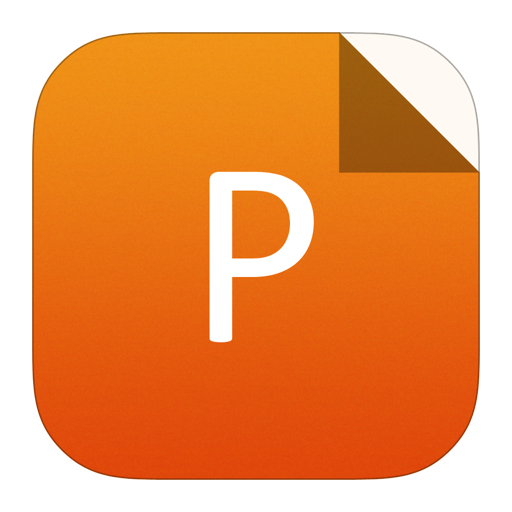
PowerPoint slide
Figure3.
(Color online) (a) The dielectric antenna consists of, from bottom to top, a silver layer, an AlGaAs membrane (with embedded QDs), a low refractive index PMMA spacer and the GaP SIL. Most photon emission is funneled into the GaP SIL[70], reprinted with permission, Copyright 2018, Springer Nature. (b) Comparison of the photon-extraction efficiency for different micro-lens mirror structures. With DBR bottom mirror, the photon extraction efficiency reaches to a plateau value of only around 23%, while with a gold bottom mirror, it is improved to more than 80% for large numerical aperture collection objectives[71], reprinted with permission, Copyright 2015, Springer Nature. (c) Schematic view of the QD micro-lens/micro-objective device. A micro-objective is printed directed on top of a QD micro-lens[73], reprinted with permission, Copyright 2017, American Chemical Society.
3.2
Micro-lens
Despite the simplicity and the effectiveness of the SIL approach, the large footprint of the macroscopic lens prevents the possibility of addressing individual QDs independently. The idea of the lens can be transformed from macroscopic SIL to microscopic lens, i.e., micro-lens.
Similar to SIL, the micro-lens shapes the propagation of the emitted photons, effectively guiding the photons towards the collection lens. With a backside reflector, those photons not caught by the nanostructure can be reflected back to the lens and significantly improve the device performances.
By deterministically placing singe QDs in the micro-lens with a bottom distributed Bragg mirror (DBR), single-photon collection efficiency up to 23% across a broad operation bandwidth is observed (red line in Fig. 3(b))[71]. Notably, the photon indistinguishability achieved in this lens is as high as 80% even under saturation, which indicates the great potential of micro-lens for generating highly-coherent single-photons. The bandwidth and the efficiency of such a structure are basically limited by the bottom DBR that has a relatively limited bandwidth and a finite acceptance angle[72]. By replacing the DBR with a broadband metallic mirror, the calculated collection efficiency can be boosted to up to 86% as is indicated by the black line in Fig. 3(b), which is among the state-of-the-art results. The device’s performance can be further improved with the implementation of high-NA micro-objectives[73] that are aligned to the micro-lens and written with sub-micrometer accuracy (Fig. 3(c)) by means of 3D femtosecond direct laser writing[74, 75].
4.
Cavity based photonic structures: circular Bragg gratings
Apart from generating single-photons, the polarization entangled photon pairs can also be deterministically triggered from semiconductors by using the bi-exciton(XX)/exciton(X) cascaded radiative recombination process. Generally, the X and XX transitions are not frequency-degenerate, resulting in a wavelength separation of X and XX photons for a few nanometers[70, 76]. In order to obtain strongly-entangled photon pairs with high-brightness and indistinguishability, photonic nanostructures exhibiting Purcell enhancements simultaneously for both X and XX are long being sought.
One of the clever solutions is to use a low-Q circular Bragg grating (CBG) resonator proposed by Davanco et al.[7]. As shown in Figs. 4(a) and 4(c), the CBG is made by suspended semiconductor membranes with shallowly etched circular trenches. When a QD is embedded in the center of the CBG, the in-plane single-photons are partially reflected back to center, forming a low-Q cavity and also scattered preferentially upwards, enabling highly directional emission. In the early CBG devices, collection efficiency around 10% was demonstrated due to the non-ideal positions of QDs respective to the CBG center. Thanks to the cryogenic photo-luminescence imaging technique, it is feasible to optically locate the positions of the QDs and deterministically fabricate the device around them[6, 77]. A deterministically fabricated CBG device shows a collection efficiency of 49% with an NA of 0.4. The collection efficiency of CBGs is theoretically limited to 80% with a high NA objective (0.7) because of the photon leakage to the bottom of the devices[77]. Such a limitation was recently broken by the development of hybrid CBG devices in which a CBG sits on top of a highly-efficient broadband reflector consisting of a thin SiO2 layer and a gold film, see Fig. 4(d). As illustrated in Fig. 4(e), photons leaking down to the bottom of CBG are efficiently reflected back to the device regime and constructively interfere with the upwards emitted photons, resulting in a theoretical collection efficiency up to 90% over ~33 nm and a calculated Purcell factor of > 2 for a bandwidth of ~ 13 nm[3]. The unique combinations of collection efficiency and Purcell enhancements over a broad spectral regime are particularly appealing for generating high-performance entangled photon pairs. By harnessing the broadband feature of the hybrid CBG, strongly-entangled photons with unprecedented brightness and photon indistinguishability have been very recently achieved in both InGaAs QDs and GaAs QDs, immediately opening possibilities for efficiency-demanding experiments such as Boson sampling and entanglement swapping[3, 78].

class="figure_img" id="Figure4"/>
Download
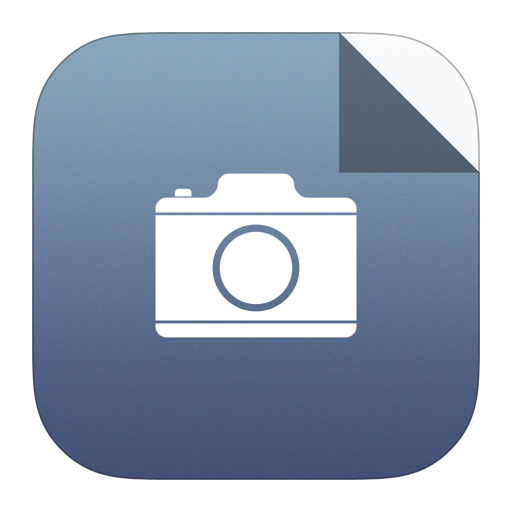
Larger image
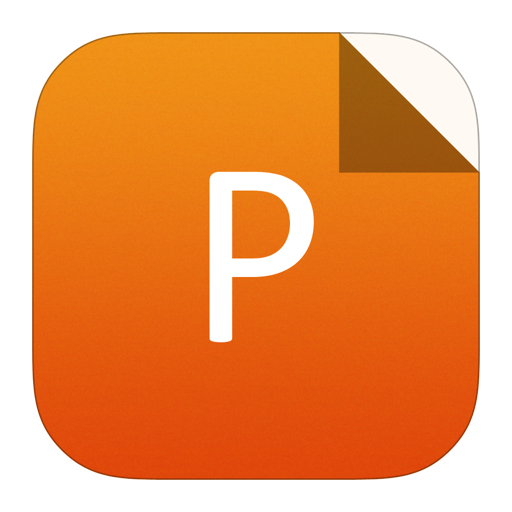
PowerPoint slide
Figure4.
(Color online) (a–c) SEM images of CBG structure[7], (a–c) are reprinted with permission, Copyright 2011, AIP Publishing. (d) The schematic of the CBR-HBR. (e) Simulated Purcell facor and collection efficiency of the CBR-HBR[3], (d) and (e) are reprinted with permission, Copyright 2019, Nature Springer.
5.
Conclusion
Last decades have witnessed the race of different photonic structures for the realizations of semiconductor quantum light sources. The broadband photonic structures greatly reduce the challenge of spectral resonance between the QDs and the structures which is particularly technically difficult for the high-Q cavities. In addition, the propagating mode feature of the waveguide based structure offers a unique opportunity of routing light in planar chips, facilitating the on-chip integration of multiple functional devices. The unique combination of broadband enhancements of spontaneous emission rate and collection efficiency simultaneously further enables the on-demand generation of non-classic state beyond single-photons, i.e., entangled photon pairs. With further developments of the broadband photonic structures, more advanced quantum photonic experiments could be envisioned in the near future, e.g., two-photon quantum random walking and multi-photon boson sampling etc.
Acknowledgments
This work was supported by National Key R&D Program of China (No. 2018YFA0306100), the National Natural Science Foundations of China (Nos. 11874437,11704424), the Natural Science Foundation of Guangdong Province (Nos. 2018B030311027, 2017A030310004, 2016A030310216) and Guangzhou Science and Technology Project (No. 201805010004). the National Natural Science Foundation of China (No. 60123456).