1.
Introduction
Besides the breakthrough fabrication of single-layered graphene[1, 2], the transition-metal dichalcogenides (TMDs) with MoS2 as the prototype material become another attractive family of atomically thin two-dimensioned (2D) materials that were successfully exfoliated from three-dimensional bulk phases[3]. The 2D TMDs show reasonably high carrier mobility (e.g., ~200 cm2V?1s?1 for MoS2 at room temperature[4, 5]) and direct optical band gap in the visible regime at monolayer limit (e.g., 1.8 eV for MoS2[6]). Additionally, it has been demonstrated that the flexibly tunable electronic and optical properties can be realized by controlling the structural parameters such as layer thickness[6–9] and stacking pattern of multiple layers[10–13], as well as applying strains[14–19] and external fields[20, 21]. Therefore, the family of 2D TMDs has attracted significant research interest for potential electronic[5, 22–24] and optoelectronic applications[25–27].
Carrier effective mass (m*), defined as the second derivative of energy dispersion, is a physical quantity influencing almost all the transport properties. It is therefore a critical property of the 2D TMDs for advancing their electronic and optoelectronic applications. While the carrier effective masses of MoS2 have been measured experimentally (for instance evaluated from the dispersion curve from the angle-resolved photoemission spectroscopy measurement[8]) and calculated theoretically[19, 28], the systemic study of the effective masses of all the members in the 2D TMDs family is, to our best knowledge, not yet available. Moreover, the multiple homogenous layers of 2D TMDs and the heterostructures consisting of different sublayers have been used/considered for electronic and optoelectronic devices[5, 22–27]. Studies of the layer dependence of carrier effective masses and how the effective masses change in the heterostructures are desired to purposely optimize carriers transport and improve the corresponding device performance.
In addition, the most remarkable feature of the 2D TMDs is the indirect to direct band-gap transition from multiple layers to monolayer[6], which converts the system from an optically inactive material into a strong light absorber/emitter. The physical mechanism underlying this indirect to direct band-gap transition was attributed to the kinetic-energy controlled quantum confinement effect[6, 8, 9], or the weak interlayer coupling of extended electronic states[29–31], which is still not yet conclusive. In fact, the mechanism could to some extent be revealed by simply evaluating the out-of-layer component of carrier effective mass. This is because in the purely quantum confinement dominated picture, the dependences of electron and hole energy levels on sample size can be described by the finite potential-well model, where the carrier effective mass with respect to the confinement direction enters as a constant (as shown in Fig. 1). The slower change of the curve (i.e., the red one) means the larger carrier effective mass. If the dependences of electron and hole energy levels do not comply with the finite potential-well model, the underlying physics is not the quantum confinement effect.
In such a context, we present here a theoretical study of the carrier effective masses of the family of 2D TMDs by using high-throughput density functional theory based calculations. We considered various TMD members in the formula of MX2 with M = Mo, W and X = S, Se, Te and several heterostructures based on them. The in-layer and out-of-layer components of carrier effective mass, dependence of mass value on the layer thickness, and variation of mass value with different heterostructure configuration are extensively calculated. The results demonstrate chemical trends of carrier effective mass, insights on how the effective mass controls optoelectronic properties, and suggestion on optimizing the effective mass to improve carrier transport.
2.
Computational approaches
First-principles DFT calculations were carried out by using the plane-wave pseudopotential method as implemented in the VASP code[32–35]. The electron–ion interaction was treated with the projector-augmented plane-wave pseudopotentials[36, 37]. We used the generalized gradient approximation of the Perdew–Burke–Ernzerhof parameterization[38] as exchange-correlation functional. The electronic integration within the Brillouin zone was done with the k-point meshes of 22 × 22 × 5, 22 × 22 × 2, and 22 × 22 × 1 for the bulk material, thinner (1 to 4 layers) and thicker (more than 4 layers) slabs, respectively. A vacuum thickness of more than 20 ? was adopted along the out-of-layer direction to isolate 2D TMD layers. We considered the interlayer van der Waals (vdWs) interactions essential for the 2D layered materials through the DFT-D2 scheme of Grimme[39]. For all the TMDs, the lattice parameters and internal atomic positions were fully optimized with the total energy convergence threshold of 10?4 eV/atom. The carrier effective masses are evaluated through the second derivative of the energy dispersion curve. We note that the standard DFT-GGA calculations are known to underestimate the band gap and overestimate the band dispersion of the semiconductors, so the calculated effective mass values are in principle overestimated as well. In spite of this problem, DFT-GGA calculations could give the reliable dependence of effective mass with thickness, and have also been adopted in previous publications[40–42]. Therefore, the conclusion will not be affected.
3.
Results and discussions
We begin with the evaluation of the out-of-layer component of effective mass to probe the mechanism underlying the indirect to direct band-gap transition upon dimensional reduction in TMDs. For the semiconductor nanostructures where the electronic states are dominated by the quantum confinement effect, the dependences of carriers’ energy levels (En) on the nanostructure size (L) can be depicted within the finite potential-well model,
m{alpha }}}}} + {C_0}$


class="figure_img" id="Figure1"/>
Download
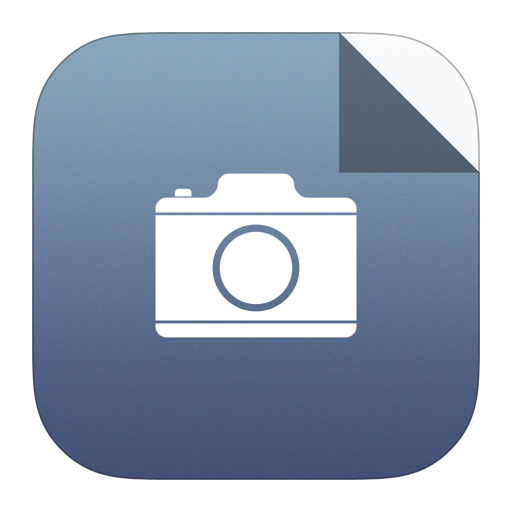
Larger image
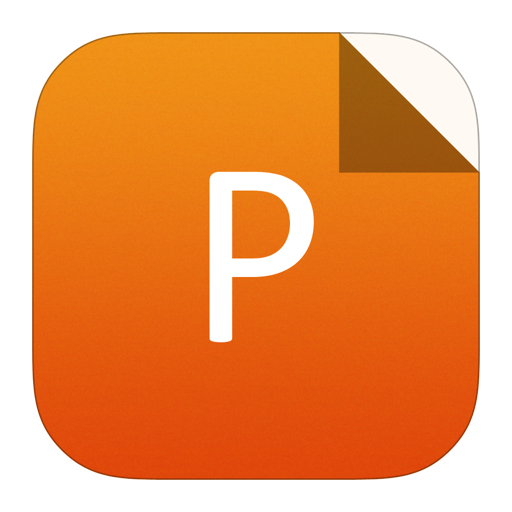
PowerPoint slide
Figure1.
(Color online) Schematic plot of the evolutions of electron and hole energy levels with the sample size L for the semiconductor nanostructures dominated by quantum confinement effect. The evolution of energy can be fitted into the finite potential-well model,
m{alpha }}}}} + {C_0}$
Fig. 2(a) shows the calculated band structure of bulk MoS2, where the band-edge states determining the indirect to direct band-gap transition are indicated. These involve TC, KC, ΓV, and KV states. The direct band gap occurs when the band edges changed from (TC, ΓV) to (KC, KV) with decreasing layer thickness[43–46]. It is known that while the TC and ΓV states are delocalized states, the KC and KV are rather localized ones[44]. Figs. 2(b)–2(e) show the energy dispersion of these states along the out-of-layer direction, based on which the out-of-layer components of effective mass (
m{out}}^*$

m{out}}^*$

m{out}}^*$

m{out}}^*$


class="figure_img" id="Figure2"/>
Download
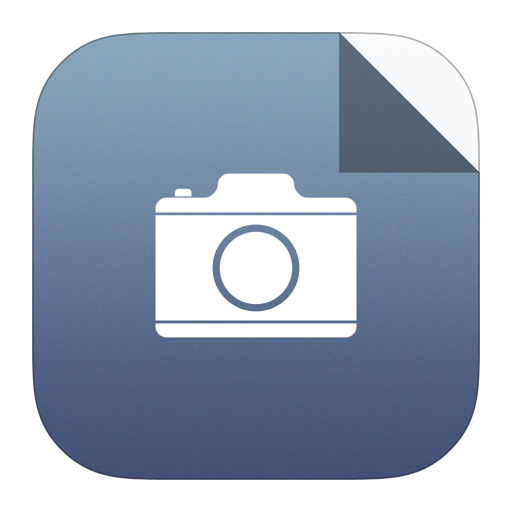
Larger image
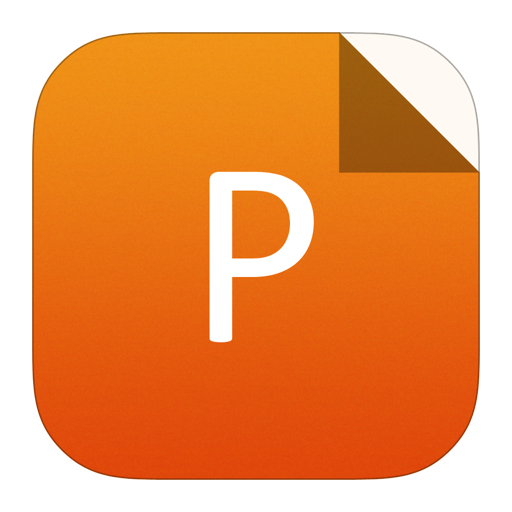
PowerPoint slide
Figure2.
(Color online) (a) Calculated band structure of bulk MoS2, where the band-edge states forming the indirect and direct band-gap transitions are indicated. (b–e) The energy dispersion of the states marked in (a) along the out-of-layer direction for each band-edge state, based on which the out-of-layer components of effective mass (
m{out}}^*}$
Fig. 3 shows the evolution of calculated band energies of the aforementioned band-edge states with the layer thickness L for MoS2. The absolute band energies were obtained by aligning the band structures at different L with respect to the averaged electrostatic potential in the vacuum region. The layer thickness L is defined by the distance between two S atoms terminating the layer plus a 1 ? region for electronic wave-function extension. As seen the ΓV state shows the most dramatic change in the evolution of band energies (green squares), though the corresponding
m{out}}^*$


class="figure_img" id="Figure3"/>
Download
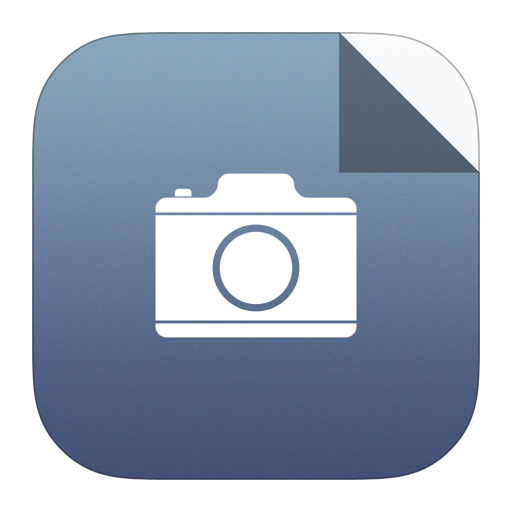
Larger image
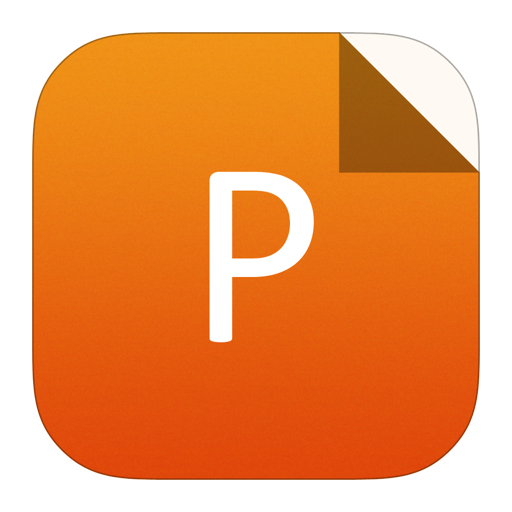
PowerPoint slide
Figure3.
(Color online) Evolution of calculated band energies of the band-edge states determining the indirect to direct band-gap transition with the thickness L for MoS2. The solid lines represent the fitting results based on the finite potential-well model (i.e., the equation mentioned above with n = 1). The dash lines are drawn as a guide to the eye.
We further extend the above calculation and analysis to other TMD materials. Table 1 lists the calculated
m{out}}^*$

Parameter | $m_{{ m{out}}}^*left( {{T_{ m{C}}}} ight)$ | $m_{{ m{out}}}^*left( {{K_{ m{C}}}} ight)$ | $m_{{ m{out}}}^*left( {{varGamma _{ m{V}}}} ight)$ | $m_{{ m{out}}}^*left( {{K_{ m{V}}}} ight)$ |
MoS2 | 0.18 | > 10.00 | 0.29 | 0.63 |
MoSe2 | 0.16 | > 10.00 | 0.42 | 0.41 |
MoTe2 | 0.13 | > 10.00 | 0.26 | 0.25 |
WS2 | 0.16 | > 10.00 | 0.22 | 0.89 |
WSe2 | 0.30 | > 10.00 | 0.33 | 1.98 |
WTe2 | 0.16 | > 10.00 | 0.17 | 0.45 |
Table1.
Calculated out-of-layer components of effective mass (
m{out}}^*$
m{out}}^*$
Table options
-->
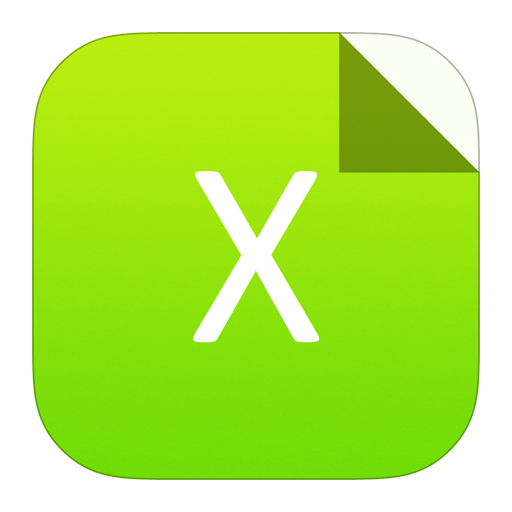
Download as CSV
Parameter | $m_{{ m{out}}}^*left( {{T_{ m{C}}}} ight)$ | $m_{{ m{out}}}^*left( {{K_{ m{C}}}} ight)$ | $m_{{ m{out}}}^*left( {{varGamma _{ m{V}}}} ight)$ | $m_{{ m{out}}}^*left( {{K_{ m{V}}}} ight)$ |
MoS2 | 0.18 | > 10.00 | 0.29 | 0.63 |
MoSe2 | 0.16 | > 10.00 | 0.42 | 0.41 |
MoTe2 | 0.13 | > 10.00 | 0.26 | 0.25 |
WS2 | 0.16 | > 10.00 | 0.22 | 0.89 |
WSe2 | 0.30 | > 10.00 | 0.33 | 1.98 |
WTe2 | 0.16 | > 10.00 | 0.17 | 0.45 |
We next calculated the in-layer component of effective mass (
m{in}}^*$

m{in}}^*$

m{in}}^*$

m{in}}^*$

m{in}}^*$

m{in}}^*$

m{in}}^*$

m{in}}^*$

m{in}}^*$

m{in}}^*$

m{in}}^*$


class="figure_img" id="Figure5"/>
Download
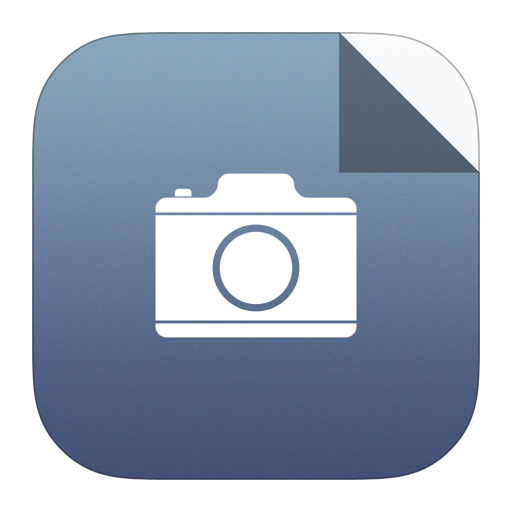
Larger image
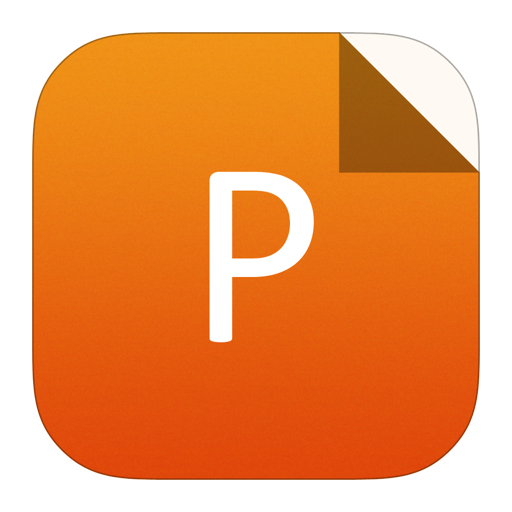
PowerPoint slide
Figure5.
(Color online) Evolution of the in-layer component of effective mass (
m{in}}^*$
Parameter | Layers | $m_{{ m{in}}}^*left( {{T_{ m{C}}}} ight)$ | $m_{{ m{in}}}^*left( {{K_{ m{C}}}} ight)$ | $m_{{ m{in}}}^*left( {{varGamma _{ m{V}}}} ight)$ | $m_{{ m{in}}}^*left( {{K_{ m{V}}}} ight)$ |
MoS2 | 1 | 0.63 | 0.65 | 2.49 | 0.69 |
10 | 0.61 | 0.87 | 0.67 | 0.65 | |
MoSe2 | 1 | 0.60 | 0.74 | 3.63 | 0.77 |
10 | 0.54 | 1.34 | 0.81 | 0.73 | |
MoTe2 | 1 | 0.49 | 0.75 | 10.93 | 0.86 |
10 | 0.41 | 1.34 | 1.15 | 0.83 | |
WS2 | 1 | 0.59 | 0.75 | 2.18 | 0.57 |
10 | 0.59 | 2.08 | 0.75 | 0.54 | |
WSe2 | 1 | 0.54 | 0.48 | 3.43 | 0.62 |
10 | 0.54 | 0.65 | 0.85 | 0.57 | |
WTe2 | 1 | 0.43 | 0.53 | 8.74 | 0.67 |
10 | 0.42 | 0.84 | 0.98 | 0.59 |
Table2.
Calculated in-layer components of effective mass (
m{in}}^*$
Table options
-->
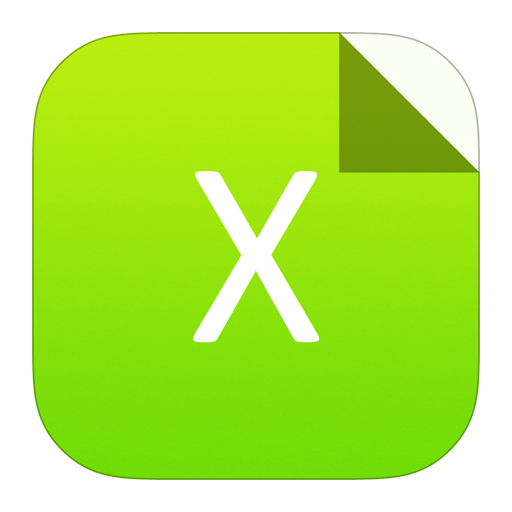
Download as CSV
Parameter | Layers | $m_{{ m{in}}}^*left( {{T_{ m{C}}}} ight)$ | $m_{{ m{in}}}^*left( {{K_{ m{C}}}} ight)$ | $m_{{ m{in}}}^*left( {{varGamma _{ m{V}}}} ight)$ | $m_{{ m{in}}}^*left( {{K_{ m{V}}}} ight)$ |
MoS2 | 1 | 0.63 | 0.65 | 2.49 | 0.69 |
10 | 0.61 | 0.87 | 0.67 | 0.65 | |
MoSe2 | 1 | 0.60 | 0.74 | 3.63 | 0.77 |
10 | 0.54 | 1.34 | 0.81 | 0.73 | |
MoTe2 | 1 | 0.49 | 0.75 | 10.93 | 0.86 |
10 | 0.41 | 1.34 | 1.15 | 0.83 | |
WS2 | 1 | 0.59 | 0.75 | 2.18 | 0.57 |
10 | 0.59 | 2.08 | 0.75 | 0.54 | |
WSe2 | 1 | 0.54 | 0.48 | 3.43 | 0.62 |
10 | 0.54 | 0.65 | 0.85 | 0.57 | |
WTe2 | 1 | 0.43 | 0.53 | 8.74 | 0.67 |
10 | 0.42 | 0.84 | 0.98 | 0.59 |
The interlayer coupling resulted in the dependence between
m{in}}^*$

m{in}}^*$

m{in}}^*$

m{in}}^*$


class="figure_img" id="Figure4"/>
Download
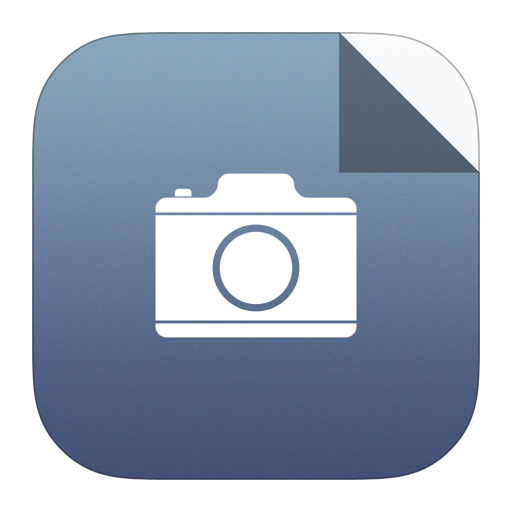
Larger image
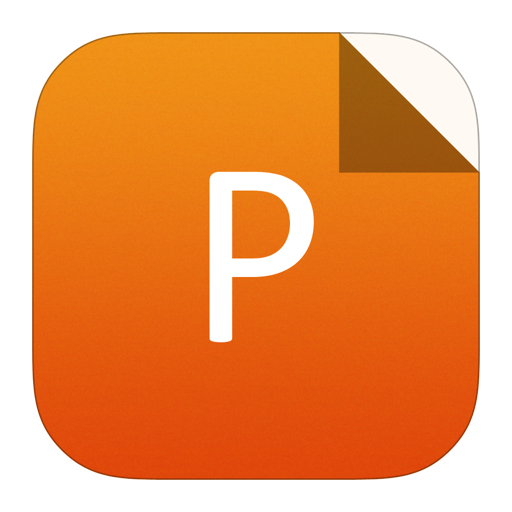
PowerPoint slide
Figure4.
(Color online) Evolution of band energies of the relevant band-edge states with the thickness L for all the investigated group-VIB TMDs MX2 (M = Mo, W and X = S, Se, Te). Similar to Fig. 3, the solid lines represent the fitting results based on the finite potential-well model and the dash lines are drawn as a guide to the eye.
Finally, we construct the van der Waals heterostructures by combining together two TMD materials and calculate their carrier effective masses. Two types of heterostructures were considered: one consists of MoS2 and MoSe2 with common cations and the other is composed of MoS2 and WS2 with common anions. The heterostructures are in the 2H symmetry and along the out-of-layer direction have the period of 10 monolayers, in which two TMDs with different compositons are randomly arranged. All possible stacking orders for each composition are considered. The heterostructures (including structural parameters and internal atomic coordinates) are fully optimized for strain relaxation. Fig. 6 shows their calculated in-layer component of effective mass (
m{in}}^*$

m{in}}^*$

m{in}}^*$

m{in}}^*$


class="figure_img" id="FigureS1"/>
Download
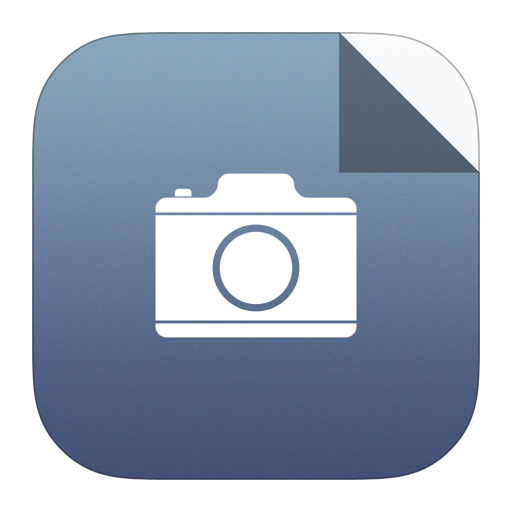
Larger image
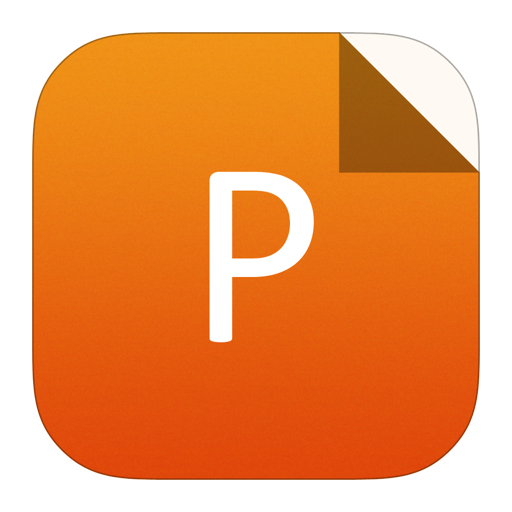
PowerPoint slide
FigureS1.
(Color online) the scheme of the stacking orders for each structures with the lowest
m{in}}^*$

class="figure_img" id="Figure6"/>
Download
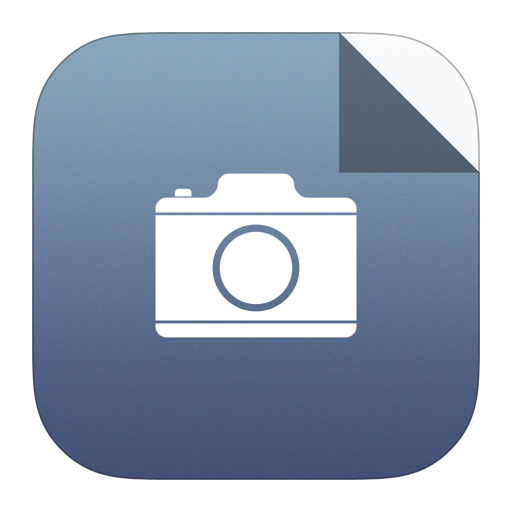
Larger image
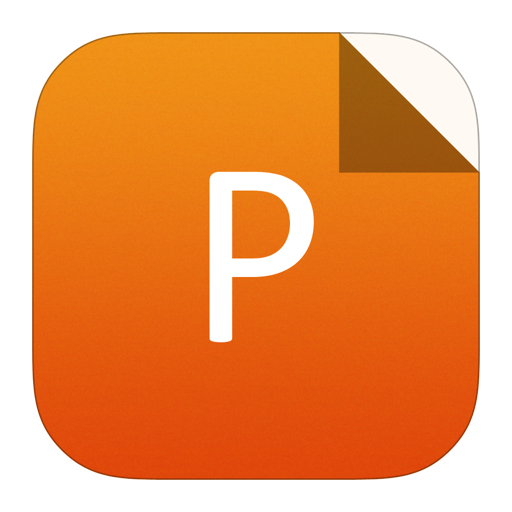
PowerPoint slide
Figure6.
(Color online) Calculated in-layer component of effective mass (
m{in}}^*$
4.
Conclusions
In summary, through first-principle density functional calculations, we present a comprehensive study of carrier effective masses for the family of two-dimensional layered group-VIB transition metal dichalcogenides (i.e., MX2 with M = Mo, W and X = S, Se, Te). Particularly we calculate the in-layer component of carrier effective masses for the single, multiple layers and also the van der Waals heterostructures, as well as the out-of-layer component for the bulk phase. Analysis of the out-of-layer effective masses within the framework of the finite potential-well model provides insight into the mechanism underlying the indirect to direct band-gap transition upon dimensional reduction. The ab initio calculated data of the in-layer effective masses give useful guidance for tuning the carrier effective masses in the two-dimensional transition metal dichalcogenides to meet the requirement of electronic and optoelectronic applications.