1.
Introduction
InP high electron mobility transistors (HEMTs) have the characteristics of high electron mobility, low noise, low power consumption and high gain[1, 2]. InP HEMTs are widely used in solid-state power microwave and millimeter-wave (MMW) electronics[3, 4]. DC and microwave performances of InP HEMTs can be greatly improved by decreasing the gate length (Lg), thereby the devices have the capability of being used in higher frequency[5–7]. The integration of devices into a circuit environment for circuit design and simulation requires compact modeling of the device operating under various bias conditions and different signal levels. This has led to the desire for compact models that accurately predict DC, AC (small- and large-signal), trans-conductance frequency dispersion and other characteristics of devices. Developing models of InP HEMTs has an important role for accurate application and re-manipulation of these characteristics.
Many commercial experience models, including the Curtice model[8], the Statz model[9], the Angelov–Chalmers model[10] and the EEHEMT model implemented in Keysight Advanced Design Systems (ADS)[11] have been reported. Special functions such as the hyperbolic function are used to develop most of these models. These compact model[8–11] forms are based on GaAs MESFET/HFET or silicon devices, without considering the necessary physical mechanisms in InP technology. Compact models that are suitable for GaN HEMTs large-signal modeling have been proposed in Refs. [12, 13]. There are also several models proposed for use with GaN HEMTs, each with its own focus on a narrow range of applications[14–17]. InP HEMTs have unique physical properties. InAlAs/ InGaAs interface in InP devices has greater conduction band discontinuity, high electron density of two electrons, high ternary electron concentration in conductive channel, and greatly improves the current processing capability of the device. InP HEMT has a higher operating frequency than GaN HEMT and GaAs HEMT and the models of GaN HEMT and GaAs HEMT can only be used for reference[18, 19]. The study of large-signal modeling of InP HEMTs has been proposed in Refs. [20, 21]. Two models[22, 23] proposed for use with InP HEMTs focus more on low-frequency noise. The result presented in Ref. [24] just described the current-voltage characteristics of InP HEMT. Research on large-signal modeling for short gate length InP HEMTs is less involved, and understanding of the physical characteristics of the InP HEMTs is helpful for the modeling of the devices.
HEMTs of high indium content channel usually suffer from low breakdown voltage and high output conductance, which is caused by the generation of electron–hole pair created by impact ionization[25]. This phenomenon is even more remarkable for short gate length devices where much higher electric field exists under the gate area. To improve the frequency and gain of InP HEMT devices and circuits, and to reduce noise, the key is to reduce the gate length[26]. This will lead to the drain-induced barrier lowering (DIBL) effect. The DIBL effect refers originally to a reduction of threshold voltage of the transistor at higher drain voltages and causes the output volt-ampere characteristic curve to be not saturated, that is to say, the output AC resistance is decreased and the voltage gain of the device is decreased[20, 27]. To simulate the DIBL effect caused by gate length, higher challenge is put forward for modeling.
The purpose of this paper is to introduce nonlinear modeling of InP HEMTs. In order to accurately model the drain current with DIBL effect, a method of regional current fitting is introduced[10, 28, 29]. Both the equations for channel current and gate charge models were all continuous and high-order drivable, and the proposed gate charge model satisfied the charge conservation. The channel current model could fit DC performance of devices, such as the positive, negative, cut-off and sub-threshold region. The model is implemented by using Verilog-A language, which has been complied and linked into Keysight ADS for simulation. The modeling technique have been verified through comparison with measured DC I–V, C–V, small-signal S-parameters up to 40 GHz for a 2 × 25 μm × 70 nm InP HEMT using self-aligned T-gate process, fabricated at a commercial foundry.
A description of the nonlinear large model is provided in Section 2. The method to overcome the problems caused by DIBL effect for InP HEMTs modeling is presented. In Section 3, the model verification results are provided. Section 4 summarizes and concludes this work.
2.
Model description
The nonlinear model of InP HEMTs is shown in Fig. 1. The intrinsic elements of the model consists of the bias-dependent capacitances Cgsi, Cgdi, Cdsi and the drain–source current Ids. Lg, Ld, Ls, Rg, Rd and Rs are the parasitic inductors and resistors of gate (G), drain (D) and source (S), respectively. Igd and Igs are the gate–source and gate–drain diodes, respectively. Cgsx, Cgdx and Cdsx represent the external bias-independent parasitic capacitors. Rgsx, Rgdx represent the external bias-independent parasitic resistance. A simple AC current Idp, combined with Rdp and Cdp, is introduced to characterize the transconductance frequency dispersion effect of HEMTs. The thermal sub-circuit, which describes the thermal impedance behavior is also identified in Fig. 1.

class="figure_img" id="Figure1"/>
Download
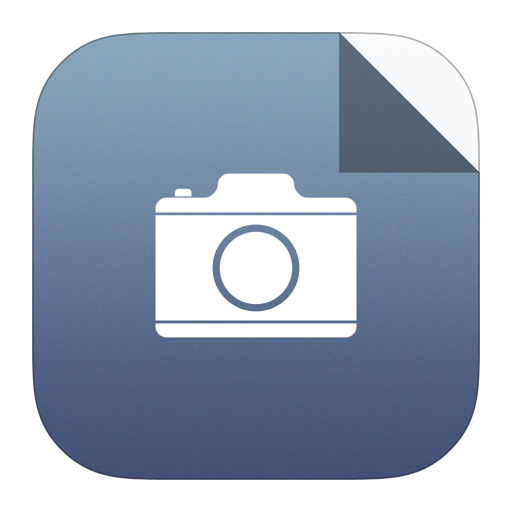
Larger image
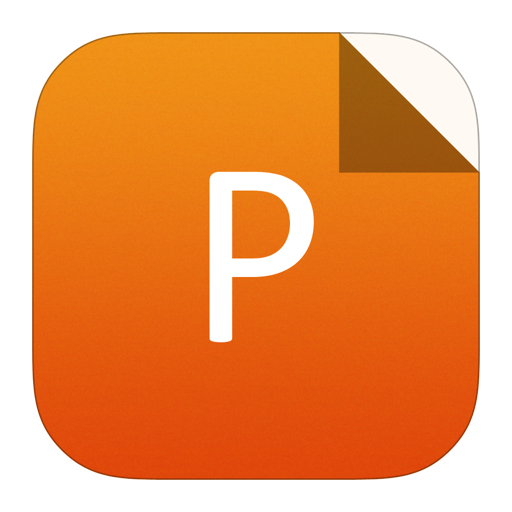
PowerPoint slide
Figure1.
(Color online) Large-signal model topology for compound semiconductor HEMTs.
A schematic cross section of typical InP HEMTs is shown in Fig. 2, in which Lg is gate length and Lc is gate cap length. The micrograph of InP HEMTs is shown in Fig. 3. The devices are fabricated by using process of self-aligned T-gate structure, dry etch and E-beam lithography. T-gate device has smaller parasitic parameters and can be used in high frequency and high gain applications. Coplanar waveguide (CPW) and common source structure are used in our device to reduce the effect of high order electromagnetic waves.

class="figure_img" id="Figure2"/>
Download
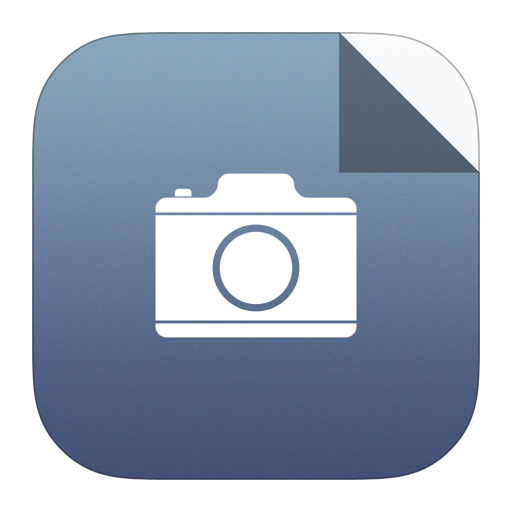
Larger image
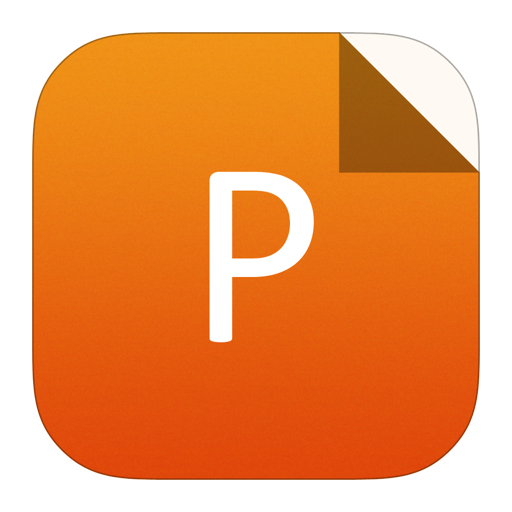
PowerPoint slide
Figure2.
(Color online) Schematic cross-section of InP HEMTs.

class="figure_img" id="Figure3"/>
Download
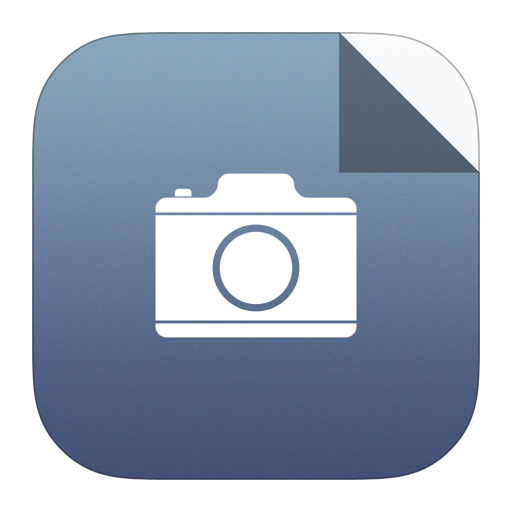
Larger image
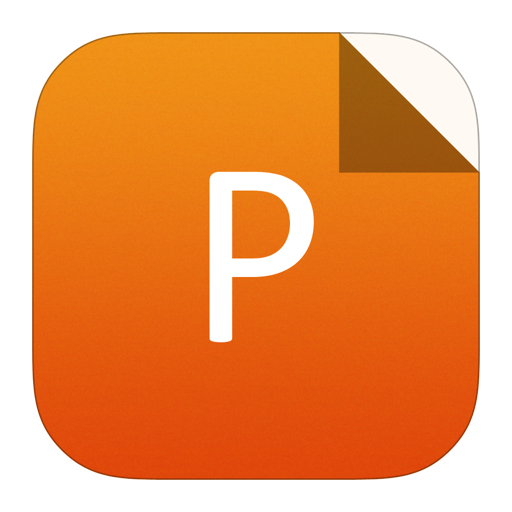
PowerPoint slide
Figure3.
(Color online) Micrograph of InP HEMTs.
2.1
Drain current model
For the drain-induced barrier lowering effect which appears when the drain–source voltage is relatively low, a method of regional drain current modeling is introduced. Fig. 3 shows the schematic of regional drain current fitting method. In order to model the I–V curve more accurately, drain current is separated into two regions, region A and region B with current Idsa and Idsb, respectively. The overall drain current is Ids = Idsa + Idsb. We use Angelov drain current equations to model both Idsa and Idsb.

class="figure_img" id="Figure4"/>
Download
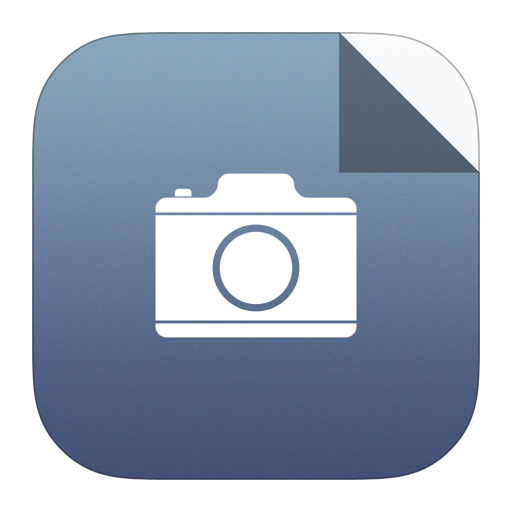
Larger image
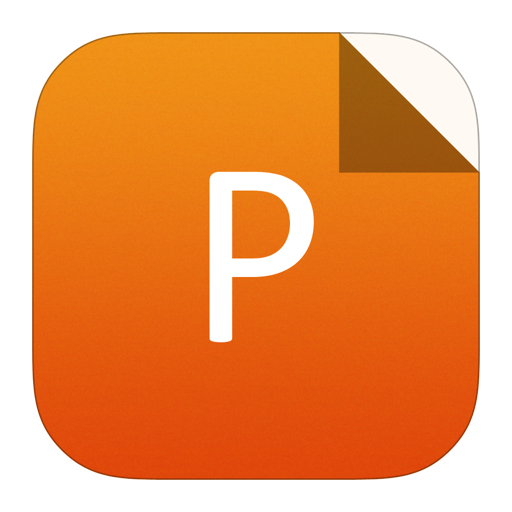
PowerPoint slide
Figure4.
(Color online) Schematic of regional drain current fitting method.
The equation for the drain current in the nonlinear model is
$${I_{ m {ds}}} = {I_{ m {dsa}}} + {I_{ m {dsb}}},$$ ![]() | (1) |
$${I_{ m {dsx}}} = 0.5left( {{I_{ m {dsp_x}}} - {I_{ m {dsn_x}}}} ight),$$ ![]() | (2) |
$$begin{split}{I_{ m {dsp_x}}} =,,& {I_{ m {pk0x}}}tanh left( {{psi _{ m {px}}}} ight)left[ {1 + tanh left( {{alpha _{ m {px}}}{V_{ m {ds}}}} ight)} ight] & times ,, left[ {1 + {lambda _{ m {px}}}{V_{ m {ds}}} + {lambda _{ m {p1x}}}exp left( {frac{{{V_{{ m{ds}}}}}}{{{V_{ m {knx}}}}} - 1} ight)} ight],end{split}$$ ![]() | (3) |
$$begin{split}{I_{ m {dsn_x}}} =,, & {I_{ m {pk0x}}}tanh left( {{psi _{ m {nx}}}} ight)left[ {1 - tanh left( {{alpha _{ m {nx}}}{V_{ m {ds}}}} ight)} ight] & timesleft[ {1 - {lambda _{ m {nx}}}{V_{ m {ds}}} - {lambda _{ m {n1x}}}exp left( {frac{{{V_{{ m{ds}}}}}}{{{V_{ m {knx}}}}} - 1} ight)} ight],end{split}$$ ![]() | (4) |
$$begin{split}{psi _{ m {px}}}=,, & {P_{ m {1mx}}}left( {{V_{ m {gs}}} - {V_{ m {pkm_x}}}} ight) + {P_{ m 2x}}{left( {{V_{ m {gs}}} - {V_{ m {pkm_x}}}} ight)^2}& + {P_{ m {3x}}}{left( {{V_{ m {gs}}} - {V_{ m {pkm_x}}}} ight)^3},end{split}$$ ![]() | (5) |
$$begin{split}{psi _{ m {nx}}} =,, & {P_{ m {1mx}}}left( {{V_{ m {gd}}} - {V_{ m {pkm_x}}}} ight) + {P_{ m {2x}}}{left( {{V_{ m {gd}}} - {V_{ m {pkm_x}}}} ight)^2} &+ {P_{ m {3x}}}{left( {{V_{ m {gd}}} - {V_{ m {pkm_x}}}} ight)^3},end{split}$$ ![]() | (6) |
$${P_{1{ m{mx}}}} = {P_{ m {1x}}}left[ {1 + {B_{ m {1x}}}/{{cosh }^2}left( {{B_{ m {2x}}}{V_{ m {ds}}}} ight)} ight],$$ ![]() | (7) |
$$begin{split}{V_{ m {pkm_x}}} = ,, & {V_{ m {pks_x}}} - {D_{ m {vpks_x}}} &+ {D_{ m {vpks_x}}}tanh left( {{A_{ m {lphas_x}}}{V_{ m {ds}}}} ight),end{split}$$ ![]() | (8) |
$${alpha _{ m {ux}}} = {A_{ m {lphar_x}}} + {A_{ m {lphas_x}}}left[ {1 + tanh left( {{psi _{ m {ux}}}} ight)} ight],$$ ![]() | (9) |
$${lambda _{ m {ux}}} = {L_{ m {ambda_x}}} + {L_{ m {vgx}}}left[ {1 + tanh left( {{psi _{ m {ux}}}} ight)} ight],$$ ![]() | (10) |
$${lambda _{ m {u1x}}} = {L_{ m {ambda_x}}} + {L_{ m {vgx}}}left[ {1 + tanh left( {{psi _{ m {ux}}}} ight)} ight],$$ ![]() | (11) |
where the B1x, B2x, P1x, P2x, P3x, Ipk0x, Vpks_x, Dvpks_x, Alphar_x, Alphas_x, Vtrx, Lambda_x, Lambda1_x, Lvgx, Vknx, λpx, λnx, λp1x, λn1x are the model parameters for drain current Idsa and Idsb. The value of x can be taken as a or b. The value of u in Eqs. (9)–(11) can be taken as n or p. The Eqs. (2)–(11) describe how to model the drain current Idsa and Idsb. The Ipk0x is the drain current at which the maximum of the transconductance occurs. The Vpkm_x is the gate voltage at maximum transconductance. The Lambda_x and Lambda1_x are the channel length modulation parameters. The hyperbolic tangent function tanh(Ψx) and tanh(Ψnx) are mainly used to simulate the general contour of the I–V characteristic curve of the device. The Vtrx, Vknx, λp1x and λn1x are parameters that control the breakdown effect. The tanh(αxVds) and tanh(αnxVds) are used to control the degree of bending of the I–V characteristic curve near the knee voltage.
2.2
Terminal charges model
The gate capacitance of the large signal model is connected in the form of a charge source or a non-linear capacitor at the corresponding node in the patch structure. In order to achieve the best convergence effect, charge-based models are used to model the gate charge. The model satisfies the law of charge conservation and can guarantee the continuity and self-consistency of large signal model and small signal model.
The charge-based models can be expressed as
$${L_{ m {c1}}} = ln left[ {cosh left( {{P_{10}} + {P_{11}}{V_{ m {gsc}}} + {P_{111}}{V_{ m {ds}}}} ight)} ight],$$ ![]() | (12) |
$${Q_{ m {gs0}}} = {P_{10}} + {P_{111}}{V_{ m {ds}}} + ln left[ {cosh left( {{P_{10}} + {P_{111}}{V_{ m {ds}}}} ight)} ight],$$ ![]() | (13) |
$${L_{ m c4}} = ln left[ {cosh left( {{P_{40}} + {P_{41}}{V_{ m {gdc}}} - {P_{111}}{V_{ m {ds}}}} ight)} ight],$$ ![]() | (14) |
$${Q_{ m {gd0}}} = {P_{40}} - {P_{111}}{V_{ m {ds}}} + ln left[ {cosh left( {{P_{40}} - {P_{111}}{V_{ m {ds}}}} ight)} ight],$$ ![]() | (15) |
$$begin{split}{Q_{{text{gs}}}} =& {C_{{text{gsp0}}}}{V_{{text{gsc}}}} + {C_{{text{gsi}}}},left( {{P_{{text{hi1}}}} + {L_{{text{c1}}}} - {Q_{{text{gs0}}}}} ight), hfill & times left{ {left[ {1 - {P_{111}} + tanh left( {{P_{{text{hi2}}}}} ight)} ight]/{P_{11}} + ,,2{P_{111}}{V_{{text{gsc}}}}} ight}, hfill end{split} $$ ![]() | (16) |
$$begin{split} {Q_{{text{gd}}}} =& {C_{{text{gdp0}}}}{V_{{text{gdc}}}} + {C_{{text{gdi}}}} left( {{P_{{text{hi4}}}} + {L_{{text{c4}}}} - {Q_{{text{gd0}}}}} ight) hfill & times left{ {left[ {1 - {P_{111}} + tanh left( {{P_{{text{hi3}}}}} ight)} ight]/{P_{41}} + ,,2{P_{111}}{V_{{text{gdc}}}}} ight},end{split}$$ ![]() | (17) |
where Phi1 = P10 + P11Vgsc + P111Vds, Phi2 = P20 + P21Vds, Phi3 = P30 ? P31Vds, Phi4 = P40 + P41Vgdc ? P111Vds. The main structure of the charge model equation is the ln (cosh ()) function, and the Pij (i = 1, 2, 3, 4; j = 0, 1) parameters are used to control the main trend of the C–V curve. The Qgs and Qgd equations can be used to derive the equations for Cgs and Cgs. Cgsp0, Cgdp0 is the gate–source capacitance and gate leakage capacitance of the device cutoff state. Cgsp0 and Cgsi, P11, P10 parameters are used to control the trend of the linear and saturation regions of the Cgs–Vgs curve. The P21 and P20 parameters are used to describe the effect of Vds on the Cgs capacitance. Cgdp0 and Cgdi, P31 and P30 are used to control the main trend of Cgd capacitance with Vds. P41 and P40 parameters are used to improve the fitting accuracy of Cgd with Vgd. The parameter P111 can be used to improve the fitting accuracy of Cgs and Cgd with Vds curve.
3.
Model verification
For verification, an InP HEMT with 2 gate fingers, that has a 25 μm gate width for each finger, 70 nm gate length, was fabricated by a commercial technology. On-wafer measure is executed for parameter extraction. S-parameters were measured and de-embedded (open + short) for parasitics introduced by GSG PAD using an Agilent E8364A network analyzer and a CASCADE Summit probe station for model parameter extraction. The DC characteristics of the device were measured using an Agilent 4156C Semiconductor Parameter Analyzer. In this work, extrinsic parasitics are extracted by using pinched and cold S-parameters. Then Ids model parameters can be determined from DC Ids–Vds, Ids–Vgs measurements. Intrinsic charge model parameters are extracted using hot S-parameters. The model parameters for Igs and Igd are determined from the forward bias I–V measurements. Cth is set to 1 μF for thermal effect simulation. Cdp and Rdp are simply determined by optimizing the gm and gds calculated from the hot S-parameters. The extracted results from the 2-finger HEMT are listed in Tables 1 and 2.
The model equation is described in Verilog-A language and is implanted in the Keysight ADS simulation tool for simulation. The model also implements the integration in the Keysight IC-CAP environment and extracts the model parameters.
In order to evaluate the accuracy of the model fitting, the relative error equation is introduced to evaluate the fitting ability of the model to the DC characteristics and AC characteristics of the device. The equation is as follows:
$${ m {RMS}}{_{ m {targ et}}} = sqrt {frac{1}{N}sumlimits_{i = 1}^N {{ m {Meas}}{{left( {i} ight)}^2}} } ,$$ ![]() | (18) |
$${ m{RM}}{{ m{S}}_{{ m{error}}}} = sqrt {frac{1}{N}sumlimits_{i = 1}^N {frac{{{{left| {{ m{Meas}}left( i ight){ m{ - Simu}}left( i ight)} ight|}^2}}}{{{{left| {{ m{RM}}{{ m{S}}_{{ m{t}}arg { m{et}}}}} ight|}^2}}}} } .$$ ![]() | (19) |
Meas(i) in Eqs. (18) and (19) represents the i-th measurement data, Simu(i) represents the i-th simulation data, and N represents the total number of data points.
Figs. 5 and 6 show the measured and simulated Ids versus Vds and gds versus Vds at at Tnom = 25 °C for Vgs = ?0.5 to 0.1 V, 0.1 V steps and Vds = 0 to 1.2 V, 0.05 V steps. Figs. 7 and 8 show the measured and simulated gm versus Vgs and Ids versus Vgs at Tnom = 25 °C for Vds = 0 to 1.2 V, 0.2 V steps and Vgs = ?0.5 to 0.1 V, 0.025 V steps.

class="figure_img" id="Figure5"/>
Download
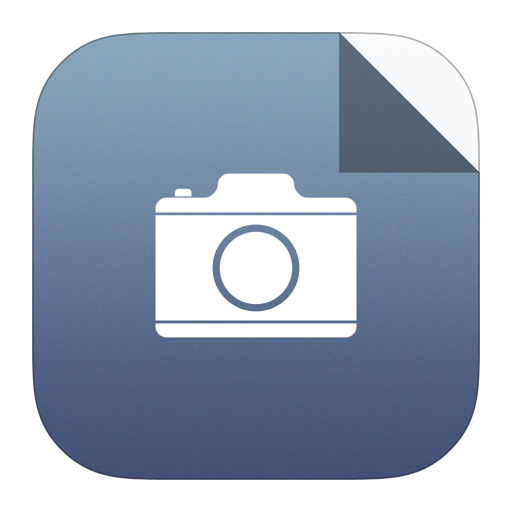
Larger image
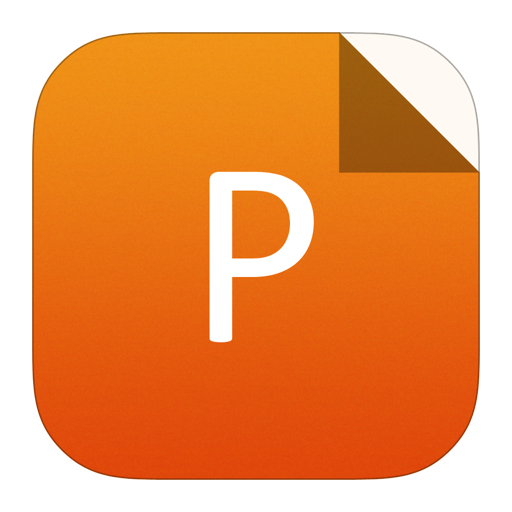
PowerPoint slide
Figure5.
(Color online) Measured and simulated Ids versus Vds at Tnom = 25 °C for Vgs = ?0.5 to 0.1 V, 0.1 V steps and Vds = 0 to 1.2 V, 0.05 V steps.

class="figure_img" id="Figure6"/>
Download
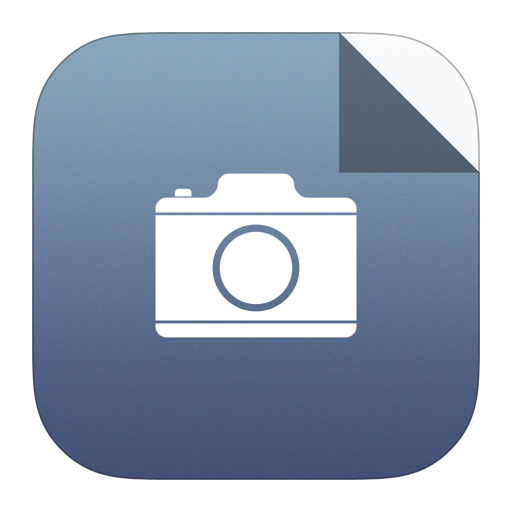
Larger image
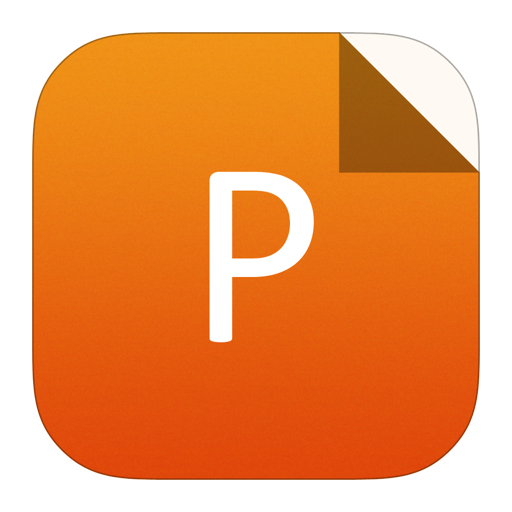
PowerPoint slide
Figure6.
(Color online) Measured and simulated gds versus Vds at Tnom = 25 °C for Vgs = ?0.5 to 0.1 V, 0.1 V steps and Vds= 0 to 1.2 V, 0.05 V steps.

class="figure_img" id="Figure7"/>
Download
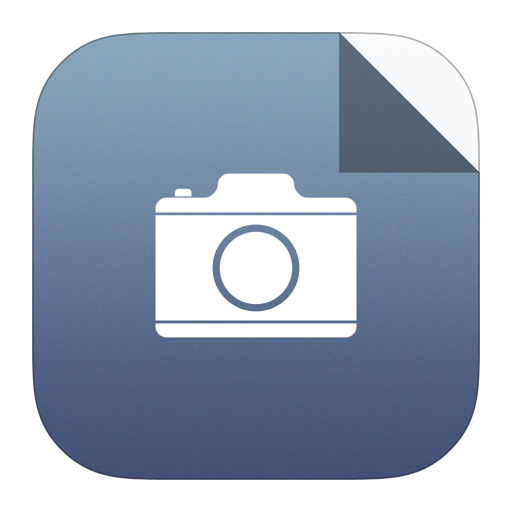
Larger image
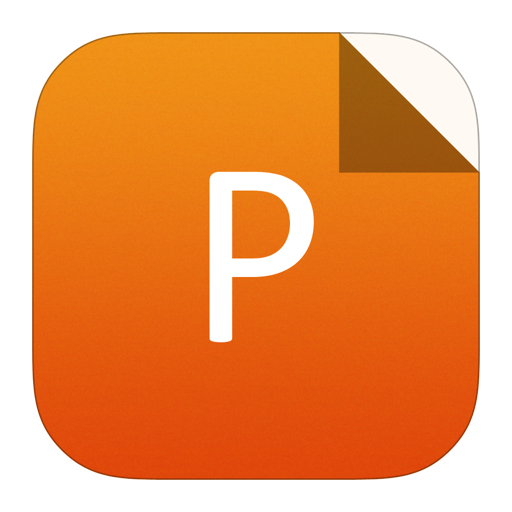
PowerPoint slide
Figure7.
(Color online) Measured and simulated gm versus Vgs at Tnom = 25 °C for Vds = 0 to 1.2 V, 0.2 V steps and Vgs = ?0.5 to 0.1 V, 0.025 V steps.

class="figure_img" id="Figure8"/>
Download
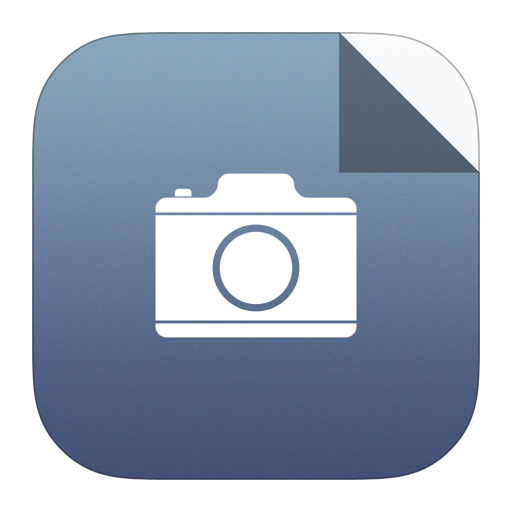
Larger image
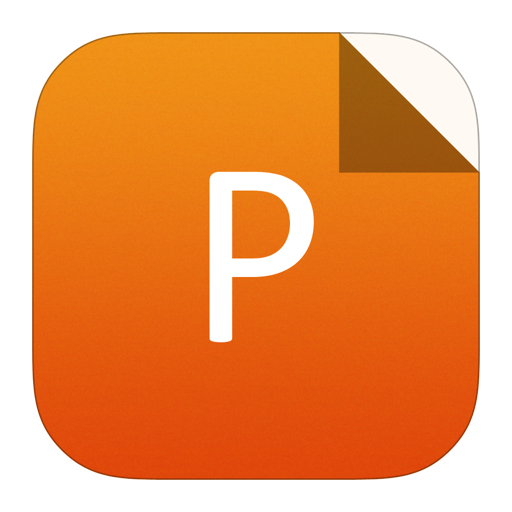
PowerPoint slide
Figure8.
(Color online) Measured and simulated Ids versus Vgs at Tnom = 25 °C for Vds = 0 to 1.2 V, 0.2 V steps and Vgs = ?0.5 to 0.1 V, 0.025 V steps.
It can be seen from the output characteristic curve that the fitting accuracy of the Angelov-GaN model in saturation region is low and the RMSerror is large. The relative error of Ids versus Vds of propesd model is 2.1% while the the traditional model is 66.6%. The relative error of gds versus Vds of propesd model is 15.2% while the the traditional model is 90.3%. The leakage barrier reduction effect is not considered in the traditional model. From the I–V curve and the transconductance/leakage conductance curve, the fitting degree of the new proposed model can accurately fitting the DC characteristics of the InP HEMTs. The I–V curve is very smooth, and the overall fitting precision is high, which can accurately simulate the DC characteristics of the InP HEMT device.
Fig. 9 shows the comparison of the C–V characteristics of the devices extracted from the bias-dependent S-parameters obtained from the test and simulation. The gate charge model parameters are listed in Table 2. It can be seen that the measurement curve and simulation curve that the improved model has better fitting effect than the traditional model. The relative error of Cgs of proposed model is 8.2% while the the traditional model is 12.5%. The relative error of Cgd of proposed model is 9.3% while the the traditional model is 14.1%. From the comparison results, the proposed capacitance model the gate charge model can be proposed to achieve accurate fitting of the C–V characteristics of the InP HEMTs.

class="figure_img" id="Figure9"/>
Download
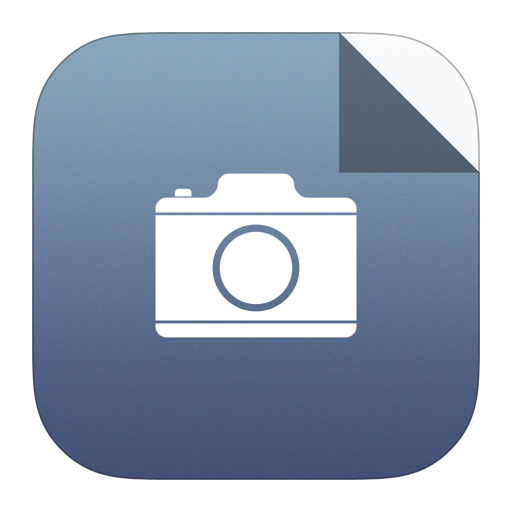
Larger image
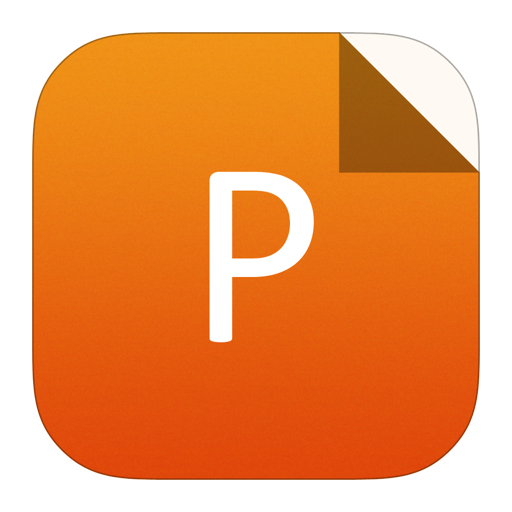
PowerPoint slide
Figure9.
(Color online) Cgs and Cgd extracted from measured and simulated S-parameters at Freq = 10 GHz, Tnom = 25 °C for Vgs = ?0.5 to 0.1 V, 0.05 V steps and Vds= 0 to 0.9 V, 0.3 V steps.
To demonstrate the accuracy of the model for small-signal RF drive condition, small-signal S-parameters simulations of the InP HEMTs model for different biases have been performed over a frequency range of 1–40 GHz and show close agreement with measured data (see Fig. 10). Validation of the model for small-signal operation also suggests that the extraction and modeling of the parasitic extrinsic are correct.

class="figure_img" id="Figure10"/>
Download
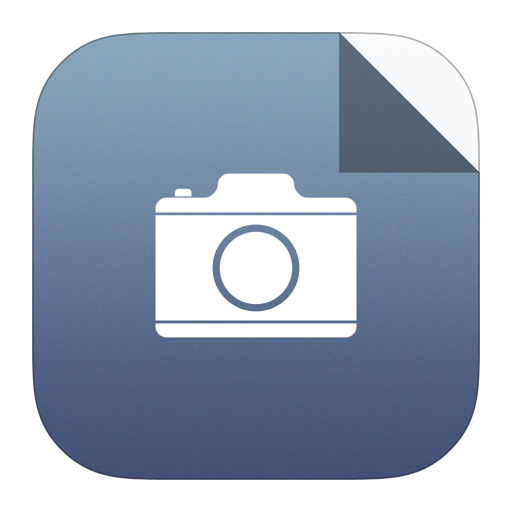
Larger image
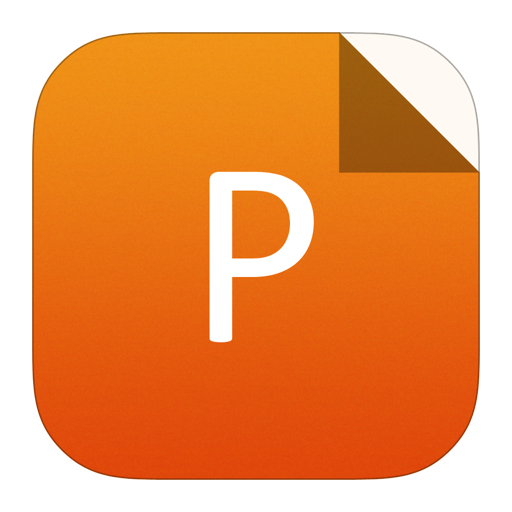
PowerPoint slide
Figure10.
(Color online) Measured and modeled S-parameters for 1 to 40 GHz, 1 GHz steps at (a) Vds = 0.8 V, Vgs = ?0.1 V and (b) Vds = 0.8 V, Vgs = ?0.05 V.
The extracted results from the 2-finger HEMT are listed in Tables 1 and 2. The experimental results show that the InP HEMT model proposed in this paper can accurately characterize the DC I–V, C–V and bias related S parameters of InP HEMTs accurately.
Parameter | Value | Parameter | Value | ||
P10 | 1.761 | P40 | 0.9383 | ||
P11 | 7.353 | P41 | 3.108 | ||
P111 | 0.1626 | Cgs0 | 1.720 fF | ||
P20 | 0.2277 | Cgdi | 9.869 fF | ||
P21 | 0.9625 | Cgd0 | 6.456 fF | ||
P30 | 1.275 | Cgdi | 0.2882 fF | ||
P31 | 0.9696 |
Table2.
Extracted terminal charges model parameter values from a 2-finger InP HEMT.
Table options
-->
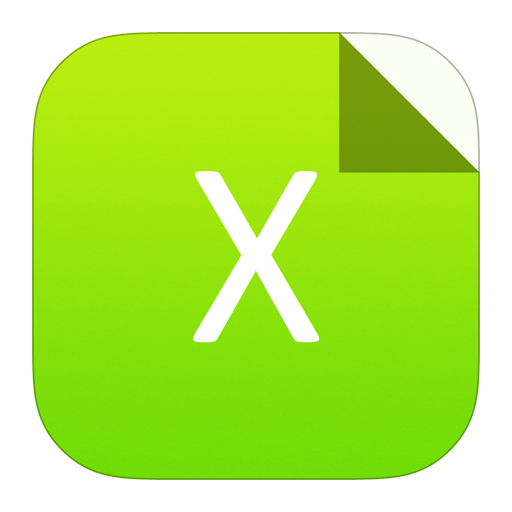
Download as CSV
Parameter | Value | Parameter | Value | ||
P10 | 1.761 | P40 | 0.9383 | ||
P11 | 7.353 | P41 | 3.108 | ||
P111 | 0.1626 | Cgs0 | 1.720 fF | ||
P20 | 0.2277 | Cgdi | 9.869 fF | ||
P21 | 0.9625 | Cgd0 | 6.456 fF | ||
P30 | 1.275 | Cgdi | 0.2882 fF | ||
P31 | 0.9696 |
Parameter | Value | Parameter | Value | ||
Ipk0a | 3.264 mA | Ipk0b | 0.010 81 mA | ||
Vpks_a | ?280.7 mV | Vpks_b | ?378.8 mV | ||
Dvpks_a | ?183.5 mV | Dvpks_b | ?1.152 V | ||
P1a | 0.6875 | P1b | 0.9929 | ||
P2a | 0.2338 | P2b | 0.4749 | ||
P3a | 0.4857 | P3b | 0.6076 | ||
Alphar_a | 13.58 | Alphar_b | 2.111 | ||
Alphas_a | 0.045 07 | Alphas_b | 2.194 | ||
Vkna | 1.0 V | Vknb | 1.0 V | ||
Vtra | 50.0 V | Vtrb | 50.0 V | ||
Lambda_a | 2.467 | Lambda_b | 0.4419 | ||
Lambda1_a | 0.004 526 | Lambda1_b | 0.067 31 | ||
B1a | 1.852 | B1b | 2.882 | ||
B2a | 0.1618 | B2b | 0.072 43 |
Table1.
Extracted drain current model parameter values from a 2-finger InP HEMT.
Table options
-->
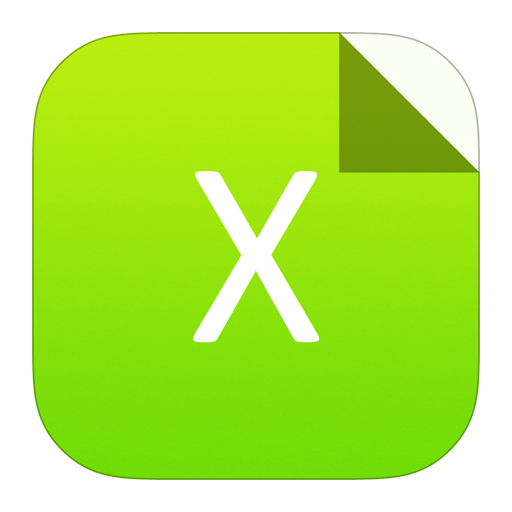
Download as CSV
Parameter | Value | Parameter | Value | ||
Ipk0a | 3.264 mA | Ipk0b | 0.010 81 mA | ||
Vpks_a | ?280.7 mV | Vpks_b | ?378.8 mV | ||
Dvpks_a | ?183.5 mV | Dvpks_b | ?1.152 V | ||
P1a | 0.6875 | P1b | 0.9929 | ||
P2a | 0.2338 | P2b | 0.4749 | ||
P3a | 0.4857 | P3b | 0.6076 | ||
Alphar_a | 13.58 | Alphar_b | 2.111 | ||
Alphas_a | 0.045 07 | Alphas_b | 2.194 | ||
Vkna | 1.0 V | Vknb | 1.0 V | ||
Vtra | 50.0 V | Vtrb | 50.0 V | ||
Lambda_a | 2.467 | Lambda_b | 0.4419 | ||
Lambda1_a | 0.004 526 | Lambda1_b | 0.067 31 | ||
B1a | 1.852 | B1b | 2.882 | ||
B2a | 0.1618 | B2b | 0.072 43 |
4.
Conclusion
An improved large-signal model of InP HEMTs is presented in this paper. A method of regional drain current modeling is introduced for the drain-induced barrier lowering effect, and good fitting result is achieved. The characterization of the charge conservation, the high-order derivability and continuity of the model equations, which are key requirements for nonlinear simulation, is thus guaranteed in the proposed model. The quality of the model is verified and validated by direct comparison to DC measurements, small signal S-parameters of a 2 × 25 μm × 70 nm InP HEMT. The nonlinear large model can be used in the simulation and design of InP HEMTs-based circuits.