1.
Introduction
Materials with a nano-crystalline structure are anticipated to display unexpected physical, mechanical and optical properties. As the grain size decreases to nano-meter scales, the grain boundary becomes more and more elusive and potent to manifest extraordinary behavior. It was identified that the presence of the grain boundaries in nano-crystalline CdS based solar cells helped them out perform their crystalline counter parts[1]. Cadmium Sulphide is an n-type semiconductor with a band gap of 2.4 eV[2–4]. Materials science and engineering research is focused on how to imbibe smart functionality in the simplest of the device structures that can be grown[5, 6]. CdS is widely studied because of its lower band gap as compared to metal oxides and because of its specific sensitivity for gas sensing application[7–10]. Gas sensing plays a vital role in today’s realm with environmentally hazardous gas being pumped from industries and household appliances to our environment.
The sequential ionic layer adsorption reaction (SILAR) method is one of the most versatile and economically feasible techniques for the growth of nano-crystalline structures[11]. This method can be used to grow/deposit materials of any dimension on any kind of substrate. The method involves the use of cationic and anionic precursor baths maintained at relatively low temperature with the substrate being immersed alternatively in the baths enabling an atom by atom layered growth[12]. The low temperature used for the deposition prevents the appearance of artifacts from film-substrate inter diffusion, contamination and impurity redistribution. The principal advantage of the SILAR technique is the control of the rate of growth of the material. By varying the adsorption and reaction time, the growth of the material layer and their subsequent properties can be controlled[12]. The technique provides veracity on the facsimile physical, electrical and optical properties of grown structures[13, 14]. The fabrication of SILAR grown devices and their characteristics play pivotal roles in developing this technique as an alternate, economic and industrially attractive technology.
Schottky diodes attract wide attention because of their ease of fabrication and multifaceted application potential in diverse sensing applications[15, 16]. Schottky diodes have been used as actuators for gases and electromagnetic radiations[17]. They have been implemented in our day to day safety systems, transportation devices and environmental monitoring schemes because of their ease of fabrication, robustness and effortlessness in implementation[18, 19]. Materials and structures that can respond uniquely to a given input serve as the bastion to the actuator industry.
A device/material is an ideal sensor for a specific reaction if its sensitivity is specific and if the device/material retraces its original state after its actuation. The prospectus of synthesizing junctions on a nano-metric dimension using the Sequential Ionic Layer Adsorption Reaction (SILAR) method motivated our research on the use of CdS/Cu Schottky diodes in nano-bulk form. We demonstrate the use of the SILAR grown nano-bulk junction for distinguishing hydrogen and oxygen and have proposed a model explaining the mechanism of oxygen sensing.
2.
Experimental
The CdS/Cu nano-bulk junction was grown on well-cleaned 30 μm-thick copper plate (2 × 5 cm2) (99.0% purity). The details of the cleaning method are reported elsewhere[1]. The cleaned sheets served as the substrate for the growth of CdS. For the growth of CdS a fully automated SILAR system was used (Prompt Engineering Ltd.) Four chemical baths were used for the growth process where, one was for cation Cadmium chloride (0.3 M), one for anion Thiourea (0.3 M), and two others for the rinsing solutions. The rinsing solution was a mixture of TEA (Triethanolamine) and distilled water taken in the ratio of 1 : 3. To deposit bulk CdS on to the Cu substrate a process sequence comprising of 40 cycles was optimized with the bath at a temperature 333 ± 2 K. The CdS/Cu structure was immersed for 300 seconds in a chemical bath containing a mixture of the same cation, anion and TEA maintained at a temperature of 333 ± 2 K with the pH of the bath maintained at 10.3 for the growth of nano CdS over the bilayer.
Using a Rigaku (D.Max.C) X-ray diffractometer, having Cu Kα (λ = 1.5406 ?) radiation and Ni filter operating at 30 kV and 20 mA, structural analysis was conducted. Surface morphology of the samples was studied using a JEOL scanning electron microscope. Thicknesses of the layers were measured using an optical interference technique. An air wedge was formed by overlaying a glass slide over the substrate, where half the length was deposited with the CdS film. Interference fringes were obtained using monochromatic light from a sodium vapor lamp. Using the measured values of the thickness of the bright and dark interference fringes, the thickness of the film is estimated using relation[20]:
$$t = frac{L}{{Delta L}} times frac{lambda }{2}, $$ ![]() | (1) |
where t is the thickness of film, L is the mean width of dark fringes, ΔL is the mean width of bright fringes and λ is the wavelength of the monochromatic light.
The current–voltage measurements were carried out using a source measuring unit (Agilent B2900). Silver electrodes were used for taking ohmic contacts from CdS. Silver electrodes 5 mm long and 2 mm in width, separated by 5 mm were pasted on to the CdS layers and cured at 50 °C for 15 min. The photoluminescence measurements were carried out on a spectroflurometer (Jasco FP8600). The excitation wavelength used was 375 nm with the excitation and emission slit width set at 2.5 nm. For the oxygen and hydrogen gas sensing the nano-bulk junction was placed within a stainless-steel gas chamber in which the specific gas flow rates could be controlled. This testing chamber contained a flat plate heater and a stainless-steel cell. The injected gas was allowed to flow into the stainless-steel cell where the flow rate could be controlled by a mass flow controller. Hydrogen and Oxygen gases with different concentrations in an air atmosphere were continuously introduced into the gas testing chamber. Kelvin probe microscopy was carried out using the KP020 Ambient Kelvin Probe System. The sample was enclosed within a Faraday enclosure to avoid stray signals during the work function measurements. The off-null detection method is used by the detection system[21]. Sample measurements were carried out in dark and illuminated conditions within the Faraday enclosure. The contact potential difference between the tip of the probe and the sample was first measured. Using a reference gold test sample the work function for the sample under test was calculated[22].
3.
Results and discussion
X-ray diffraction of the nano-bulk structure was carried out. Analysis of the diffraction data showed the presence of diffraction peaks for both Cu and CdS as shown in Fig. 1. The hexagonal cadmium sulfide phase as per the standard data from the JCPDS card (File No. 41-1049) matched with the CdS planes obtained from the sample. Hexagonal CdSs corresponding to crystal growth along the (110) and (112) planes were identified. Maximum diffraction intensity was recorded from the diffraction plane corresponding to the Cu substrate. The mean crystallite size for the (110) and (112) diffraction peak from the XRD data was calculated using the Debye–Scherrer formula[23]:

class="figure_img" id="Figure1"/>
Download
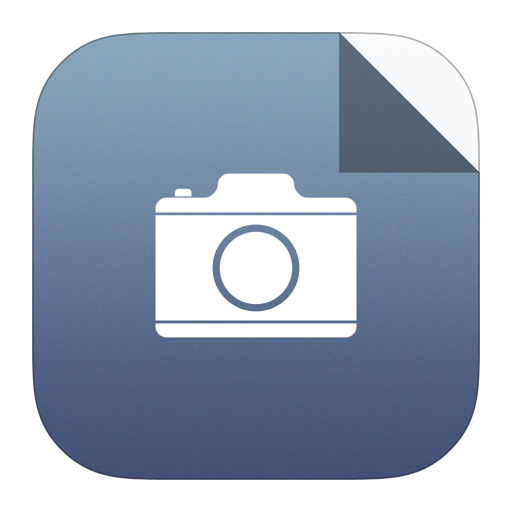
Larger image
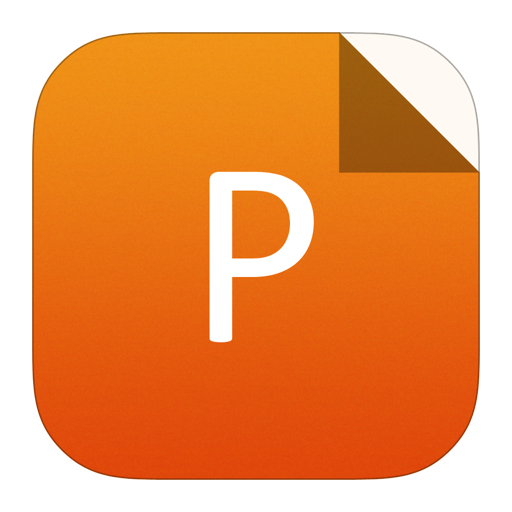
PowerPoint slide
Figure1.
X-ray diffraction spectrum of the Cu/CdS structure.
$$tau = frac{{0.9lambda }}{{beta cos theta }}, $$ ![]() | (2) |
where τ is the average diameter of the crystallite forming the films, λ = 1.5406 ?, β is the broadening of diffraction line measured at the half of its maximum intensity in radians and θ is the half angle between incident and diffracted X-ray. The average diameter using Eq. (1) was found to be ~27 nm. The total thickness of the CdS layer was measured to be around 450 nm.
Fig. 2 shows the scanning electron microscope image of the nano-bulk junction. The nano-bulk junction has a homogenous distribution of spherical crystallites with a clear demarcation between individual crystallites. Also each crystallite appears to have wound nano-wires over the crystallities, with the nano-wire having a diameter of ~70 nm (marked in the inset of the figure). This surface morphology may aid in gas adsorption in the space between the nano-wires. These observations prove that the surface morphological features of the grown nano-bulk junctions are rich of interfaces which may result in intelligent selectivity and sensitivity. XRD provides the mean scattering domain size of the volume, whereas SEM yields the surface morphology and estimate of the size based on the surface only.

class="figure_img" id="Figure2"/>
Download
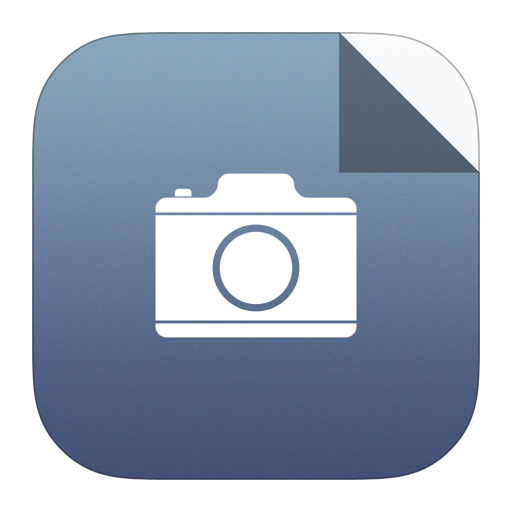
Larger image
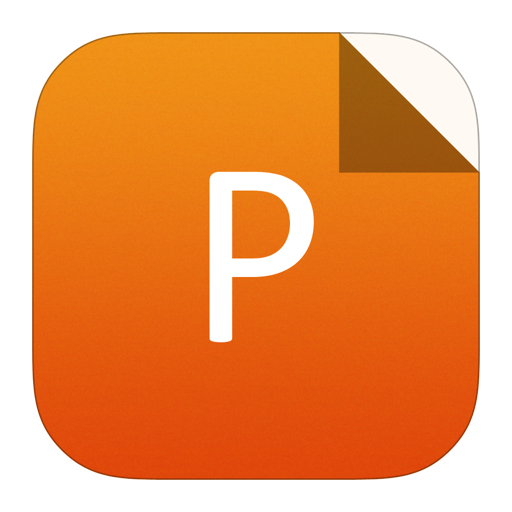
PowerPoint slide
Figure2.
SEM image of Cu/CdS bulk nano-junction.
Fig. 3 shows the photoluminescence spectra of the nano-bulk junction. The spectra clearly show that the emission spectrum is sharper and blue shifted as compared to the emission from bulk CdS. The peak emission for bulk CdS was centered ~600 nm whereas for the nano-bulk CdS it was ~511 nm. The excitation spectrum for the nano-bulk PL emission is also shown in the figure which corresponds to an excitation wavelength of 370 nm. This excitation wavelength corresponds to energy levels much higher than the bulk CdS band gap energy of 2.43 eV. Thus the blue shift in the photoluminescence emission wavelength for the nano-bulk junction relative to the bulk CdS may be the result of multiple factors like the difference in surface states, decrease in defect density, interaction between bulk and nano-particles of CdS etc.

class="figure_img" id="Figure3"/>
Download
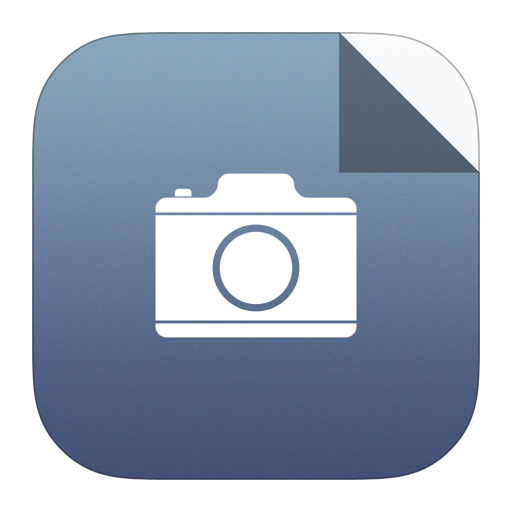
Larger image
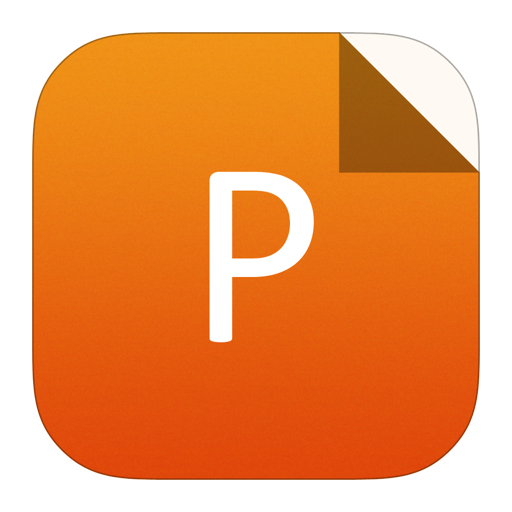
PowerPoint slide
Figure3.
PL spectrum for Cu/CdS nano-bulk junction and bulk CdS.
Fig. 4 shows the current voltage characteristic of the as prepared nano-bulk junction under the vacuum condition of 5 × 10?5 Torr. The rectification behavior indicates the formation of a Schottky barrier contact between Cu and CdS. The inset shows the current voltage relationship across two silver contacts taken from the CdS layer proving that Ag forms an ohmic contact with CdS. The figure also shows the theoretical fit to the experimental curve using Eq. (3) where A is the area of the CdS/Cu junction, A* is a constant = 23.4, q is the electronic charge, k is the Boltzmann constant, ? is the surface potential, T is the temperature in the Kelvin scale, V is the applied bias voltage and n is the diode quality factor[24]. The theoretical fit provided values for n and ? as 1.44 and 0.398 eV respectively.
$$I = A{A^*}{T^2}exp left( {frac{{ - qphi }}{{kT}}} ight)left( {exp frac{{qV}}{{nkT}} - 1} ight).$$ ![]() | (3) |
If we substitute

$$ln frac{J}{{{A^*}{T^2}}} = frac{{beta V}}{n}-beta phi .$$ ![]() | (4) |
Fig. 5 shows the plot of ln J versus voltage for the Schottky diode. As can be seen from the plot the relation between ln J and voltage is linear above 0.35 V (Region 1) where as it is logarithmic for lower bias voltages (Region 2). This indicates that there are two different conduction mechanisms in this Cu?CdS junction. In the higher bias range the ln J versus voltage plot is linear, indicating that the conduction mechanism is predominated by the thermionic emission across the CdS/Cu junction. The observed transition from the non-linear regime at lower bias voltage to the thermionic regime at higher bias voltage suggests a change in transport property. Hence, we theoretically modeled the behavior in these two bias voltage regions separately. In the lower voltage region i.e. from 0 to 0.34 V, we were able to fit the experimental curve using a theoretical relation given by:
$$y = 0.414ln x - 5.101, $$ ![]() | (5) |
and for bias voltage above 0.34 V the experimental curve could be fitted with a straight line given by:
$$y = 27.735x-15.299.$$ ![]() | (6) |
The inset in Fig. 5 shows the experimentally obtained data and the theoretical fit in the two bias voltage regions. From the mathematical fit using Eqs. (5) and (6) in tandem with Eq. (4), we obtained the diode ideality factor as 1.44 and the Schottky barrier height as 0.38 eV.

class="figure_img" id="Figure5"/>
Download
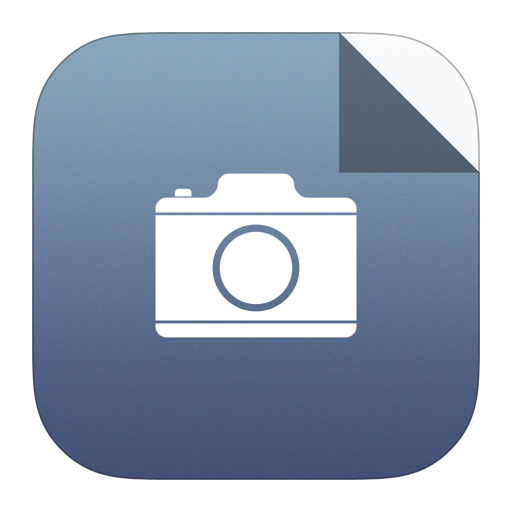
Larger image
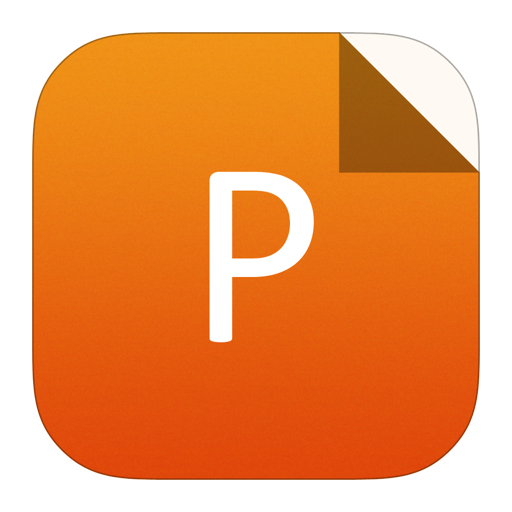
PowerPoint slide
Figure5.
Plot of ln J versus voltage for the grown Cu/CdS structure and inset shows the its theoretical fit in the two bias voltage regions (0?0.35 V and 0.36?0.45 V).
Fig. 6 shows the response to oxygen test gas exposure at 1000 ppm/air for the nano-bulk junction containing nano-CdS of the size ~180, ~100, and ~30 nm. The figure clearly shows the altered response to the same input gas with only a change in Nano-CdS. In this paper we report the results for the nano-CdS @ 30 nm since it demonstrates consistent recovery and its response function has the highest speed relative to other structures grown by us.

class="figure_img" id="Figure6"/>
Download
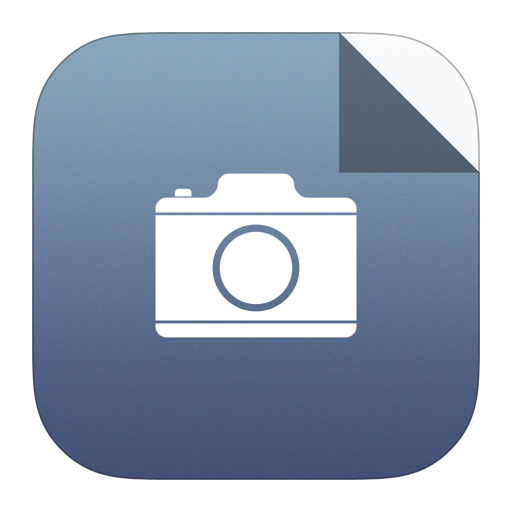
Larger image
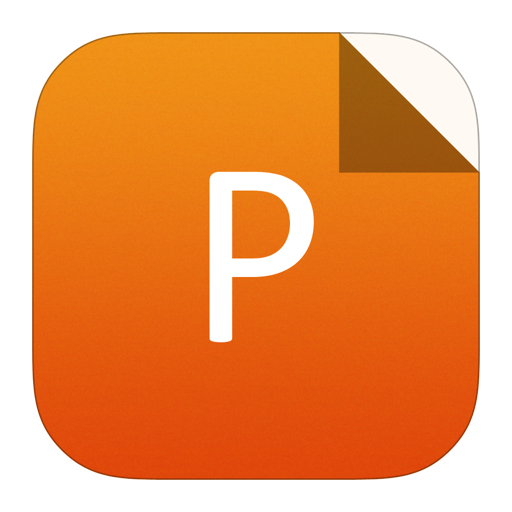
PowerPoint slide
Figure6.
Gas response curves for Cu/CdS structure grown with size controlled CdS layers.
Fig. 7(a) shows the current voltage characteristic for the nano-bulk junction containing CdS @ 30 nm when exposed to specific gas atmospheres with the operating temperature at 300 K. As can been seen from the figure, the negative bias region shows discernible sensitivity compared to the forward bias region. Hence resistance measurements under negative bias (?0.3 V) conditions were used for further characterization. The gas sensitivity defined by (Rgas/Rair) × 100, was found to be 198% for oxygen, and 34% for Hydrogen. Fig. 7(b) shows the current voltage characteristic for the nano-bulk junction when exposed to specific gas atmospheres with the operating temperature at 400 K. The gas sensitivity defined by (Rgas/Rair) × 100, was found to be 48% for oxygen and 33% for hydrogen. Thus, we may conclude that an increase in the operating temperature of the nano-bulk junction results in a rapid decrease in their response to oxygen as compared to its response to hydrogen. It is well established that oxygen sensors are influenced by their operating temperature[25]. The exact mechanism for oxygen and hydrogen sensing by the current nano-bulk junction is not well understood. However, we conclude that the conductive mechanism is altered under exposure of both test gases studied herein. There is an increase in resistance for the nano-bulk junction when exposed to oxygen or hydrogen gas at room temperature. We observe that the conductive mechanism under oxygen gas exposure is altered when the operating temperature is changed to 400 K. We model the mechanism of oxygen sensing as follows: under room temperature operation, the O2 molecules adsorbed on the CdS surface would trap the electron from the conduction band and form O2– ions. This would lead to a band bending and in effect decrease the conductivity. Under elevated operating temperature, the ions on the surface would contribute electrons to the conduction band. This would lead to a depletion of the space charge region and enhance the conductivity.

class="figure_img" id="Figure7"/>
Download
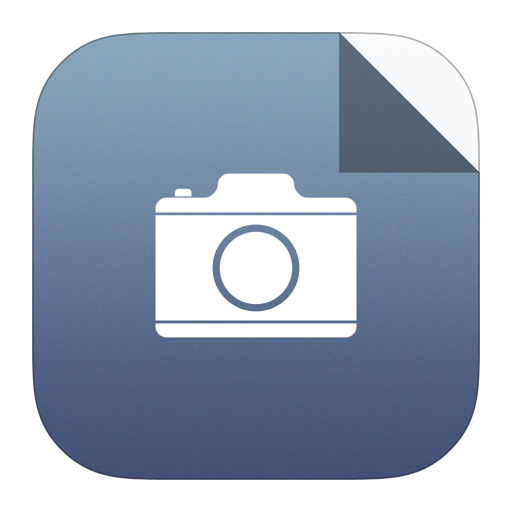
Larger image
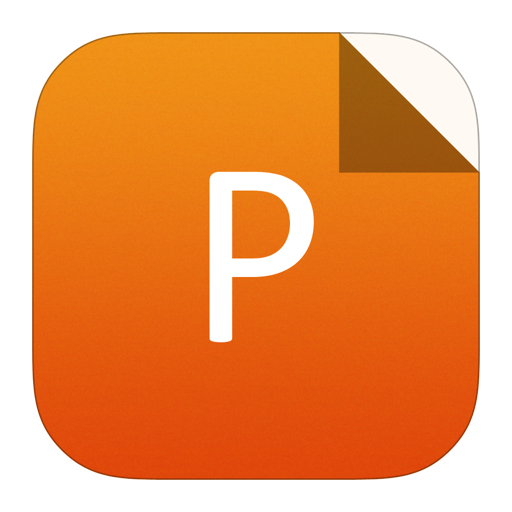
PowerPoint slide
Figure7.
Current voltage characteristics of the nano-bulk structure when exposed to specific gas atmospheres (a) at 300 K and (b) at 400 K.
The conductivity mechanism is unaltered for operation at room temperature and elevated temperature for hydrogen gas exposure where the conductivity is reduced for the device upon gas exposure. When the device is exposed to H2 gas a reaction between the H2 and surface adsorbed oxygen may be initiated, which may lead to the formation of (OH)– ions on the surface. This would lead to a band bending and in effect decrease the conductivity. The same mechanism holds at elevated temperatures also. For a working temperature of 400 K, the conductivity decreases when exposed to H2 gas whereas the conductivity increases when exposed to oxygen gas. Thus, a window to deliver the gas selective response is optimized.
To expand the insight into the fundamental oxygen detection mechanism of the CdS/Cu nano-bulk junction, gas measurements in a vacuum under different partial pressures of oxygen were carried out. The isothermal behavior of the oxygen adsorption was investigated by measuring the dependence of the sensor response on the oxygen partial pressure. The junction was operated at 300 K in a vacuum and was exposed to different partial pressures of oxygen (Fig. 8). A positive bias was applied to the device and the current was electronically held constant, so that a change in the background oxygen concentration would be directly converted into an output voltage. A logarithmic dependence on the oxygen partial pressure over was observed, indicating a Temkin isothermal behavior[25]. According to the IUPAC definition, "sensitivity" is the slope of the calibration curve, that is, sensor response versus concentration. Each isotherm represents the sensor response for different oxygen partial pressure when the CdS nano-bulk junction is maintained at the same bias. The sensitivity was calculated by taking the average of the slopes of the isotherms shown in Fig. 8, and was estimated as 0.09 V/Bar.

class="figure_img" id="Figure8"/>
Download
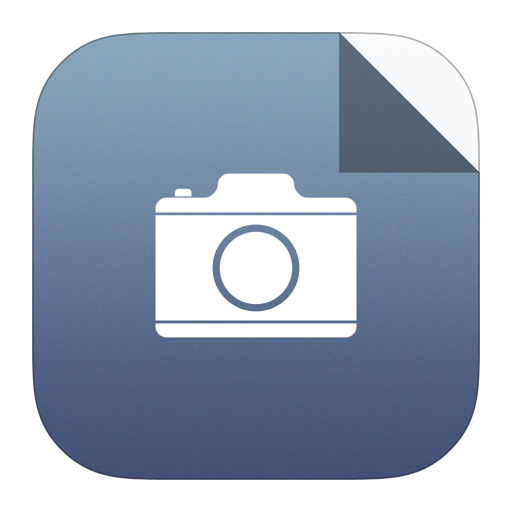
Larger image
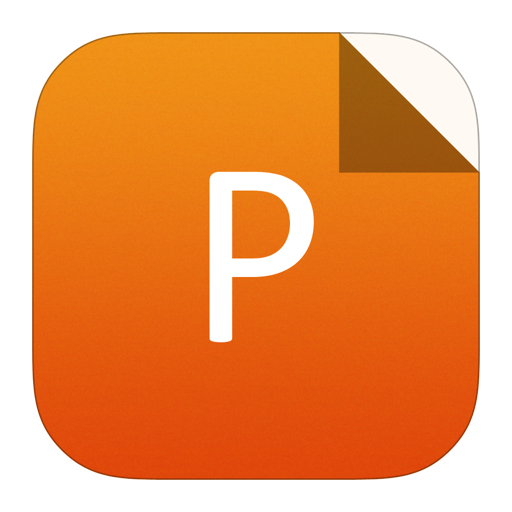
PowerPoint slide
Figure8.
Gas sensitivity in a vacuum under different partial pressures of oxygen for the nano-bulk junction.
Table 1 shows the surface potential and work function measured for the nano-bulk junction before and after soaking in an oxygen gas chamber. After soaking of the nano-bulk junction, the surface potential shifts towards lower values (for both as prepared and annealed nano-bulk junction (473 K for 2 h)), indicating the decrease of electron density and accordingly an increase in hole density. Corresponding to this the work function for the nano-bulk junction increased upon soaking, demonstrating that the exposure to an oxygen atmosphere fails to effectively separate the charges due to the built-in electric field at the interface. The built in potential is the height of the Schottky barrier on the semiconductor side, which is equal to the difference between the work function of the metal and the semiconductor. This is obtained to be 0.43 eV for the as prepared nano-bulk junction and agrees fairly with the value obtained from the theoretical fit of the I?V characteristic.
Sample | Surface potential | Work function calibrated with Au | Surface potential after soaking in Oxygen | Work function after soaking in oxygen calibrated with Au | ||
(mV) | (eV) | (mV) | (eV) | |||
Cu substrate | ?145 ± 4 | 5.105 | ||||
Cu/CdS | ?575 ± 5 | 4.675 | ?544 ± 8 | 4.706 | ||
Annealed Cu/CdS | ?584 ± 5 | 4.656 | ?584 ± 3 | 4666 |
Table1.
Surface potential and work function measured for the nano-bulk junction before and after soaking in oxygen inside a gas chamber.
Table options
-->
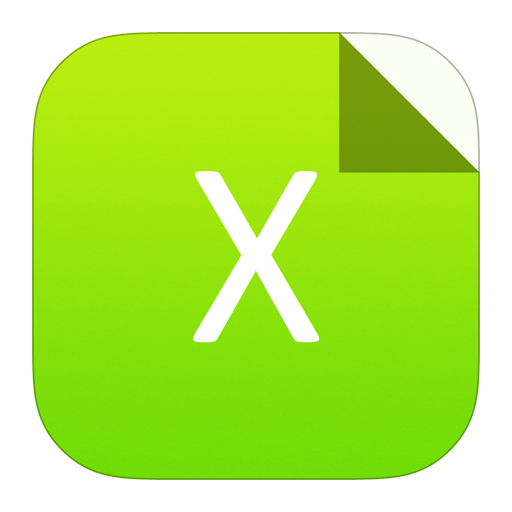
Download as CSV
Sample | Surface potential | Work function calibrated with Au | Surface potential after soaking in Oxygen | Work function after soaking in oxygen calibrated with Au | ||
(mV) | (eV) | (mV) | (eV) | |||
Cu substrate | ?145 ± 4 | 5.105 | ||||
Cu/CdS | ?575 ± 5 | 4.675 | ?544 ± 8 | 4.706 | ||
Annealed Cu/CdS | ?584 ± 5 | 4.656 | ?584 ± 3 | 4666 |
The fundamental mechanism behind the gas response is assumed to be the trapping of electrons at adsorbed molecules and band bending induced by charged molecules. Based on the surface potential and work function measurements, we model the oxygen and hydrogen sensing behavior of the nano-bulk junction as follows: in the I–V curves under different gas exposure conditions, the current saturation under negative bias is significantly deviated from the exponential current of the Schottky barrier obtained for the nano-bulk junction under vacuum conditions as seen from Fig. 4. This behavior can be attributed to the dependence of the barrier height on applied voltage and ambient conditions. Under normal conditions due to the difference in electron affinity between the two materials, electrons are transferred from the Cu side to n-CdS across the interface, and the resultant band bending leads to the formation of a barrier for electrons transport. When gas molecules are adsorbed on the surface, under an applied reverse bias, an accumulation of positive charges at the valance band gives rise to the narrowing and lowering of the barrier and thus enhances the flow of electrons through it[26]. In the forward bias, the barrier height and width are enlarged, and electrons and holes cannot tunnel from it, resulting in no change of forward current under regimes of different gas exposure.

class="figure_img" id="Figure4"/>
Download
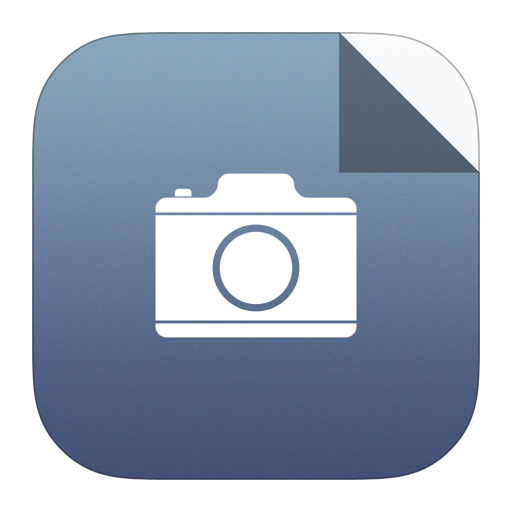
Larger image
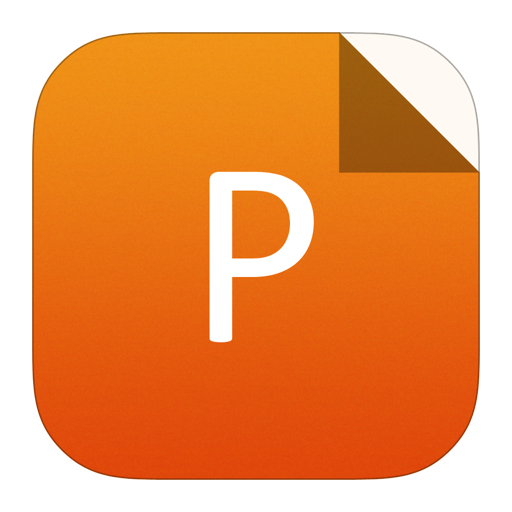
PowerPoint slide
Figure4.
Current–voltage characteristics of grown Cu/CdS structure and its theoretical fit. Inset proves the ohmic nature of the Ag/CdS contact.
4.
Conclusions
We have grown a Cu/CdS nano-bulk junction using the SILAR technique. The junction had an ideality factor of 1.44 and a Schottky barrier height of the order of 0.38?0.43 eV. The nano-bulk junction having nano-particles of CdS with size ~30 nm shows consistent recovery when subjected to gas testing procedures. The nano-bulk junction has an enhanced response to oxygen as compared to hydrogen. The chemo-response is decreased upon increasing the operating temperature indicating the possibility of the realization of an optimal operating point based on application needs. Observation of the Temkin isotherm for the oxygen adsorption processes prompts us to conclude that the heat of oxygen adsorption is a linear function of the adsorbate concentration. Surface potential and work function measurements show that exposure to an oxygen atmosphere fails to effectively separate the charges due to the built-in electric field at the interface.
Acknowledgement
The author would like to extend this gratitude to SERB and KSCSTE for the financial support provided to carry out this work vide SB/FTP/PS-013/2013 and 006/SRSPS/2014/CSTE. The author would also like to thank DST for the FIST grants sanctioned vide SR/FST/College202/2014.