1.
Introduction
Magnetism, which is the macroscopic spin ordering in spatial space, has attracted many studies in the recently flourishing research of two-dimensional (2D) materials[1-5]. In the cases of one- or two-dimension, magnetism tends to be destroyed by any thermal fluctuation in isotropic spin systems at any non-zero temperature, and this statement was recognized as the Mermin-Wagner theorem[6]. In recent years, thanks to the rapid progress in 2D materials[7, 8], scientists have managed to fabricate ultra-thin candidates hosting detectable ferromagnetic orderings[9, 10] because the 2D ferromagnetic ordering could provide ideal platforms for understanding the low-dimensional magnetism[11] and provide opportunities for realizing atomically thin, flexible magneto-optic or magnetoelectric devices.
Pioneering efforts have been made in exfoliation of monolayer and few-layers of magnetic layered bulk materials, such as NiPS3[12], FePS3[13], and CrSiTe3[14]. However, the macroscopic magnetic orders are only observed in thin films. The atomically-thick CrI3[9] and Cr2Ge2Te6[10] down to the monolayer and bilayer limit have been successfully fabricated and corresponding experiments have demonstrated the existence of the intrinsic ferromagnetism (FM). The numbers of 2D ferromagnets, such as Fe3GeTe2[15], 1T-VSe2[16], MnSex[17], and so on, then mushroomed. All monolayer 2D ferromagnetic materials display significant magnetic anisotropy, which opens a magnon excitation gap and lifts the Wagner-Mermin criteria to finite Tc by suppressing thermal fluctuations[18, 19]. Discoveries of monolayer ferromagnets not only enriched the family of 2D materials but also provide exciting possibilities for device functionalities by the seamless integration of 2D ferromagnets with other nonmagnetic 2D materials via the van der Waals (vdW) heterostructure[20]. Compared to the rapid progress in 2D ferromagnets, the research of low-dimensional antiferromagnets only appear as interlayer couplings in vdW ferromagnetic multilayers[9, 21] but are rarely reported in monolayer limit. This scenario requires precise control of the number of multilayers because even numbers of layers lead to FM and only odd numbers of layers exhibit antiferromagnetism (AFM). Note that most of the discovered magnetic 2D materials possessing ferromagnetic orderings in monolayers show no magnetism in bulk because the interlayer coupling between magnetic monolayers is antiferromagnetic.
2.
Methods
In this paper, we investigate both electronic structures and magnetic orderings of bulk and monolayer forms of chromium diiodides, based on the state-of-the-art first-principles calculations[22, 23]. The first-principles calculations are performed by using the Vienna ab initio simulation package (VASP)[24-26] within the generalized Kohn-Sham scheme. The projector augmented-wave (PAW)[27, 28] pseudopotentials are adopted, and the kinetic cut-off energy is set to be 680 eV for wave-function expansions, a

3.
Results and discussion
3.1
Bulk CrI2
The bulk CrI2 crystallizes in the P63mc space group and is consisting of two CrI2 monolayers in AB stacking. The crystal structure of bulk CrI2 is shown in Fig. 1(a), the chromium and iodine atoms are displayed with blue and purple spheres, respectively. The spin-polarized band structures of bulk CrI2 is shown in Fig. 1(b) (antiferromagnetic) and Fig. 1(c) (ferromagnetic), to compare the total energy difference between ferromagnetic and antiferromagnetic orderings, a 2 × 1 × 1 supercell is used, and the band dispersions of spin majority and spin minority is indicated by red and green lines. The corresponding spin spatial distribution is illustrated in inserts of Figs. 1(b) and 1(c)) with the same color-coding. Since the total energy difference between ferromagnetic and antiferromagentic states is 20 meV/unit cell, one can find out that, bulk CrI2 take a ferromagnetic ground state.

class="figure_img" id="Figure1"/>
Download
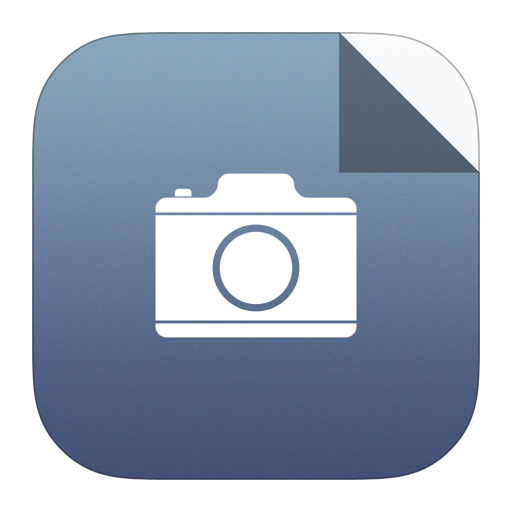
Larger image
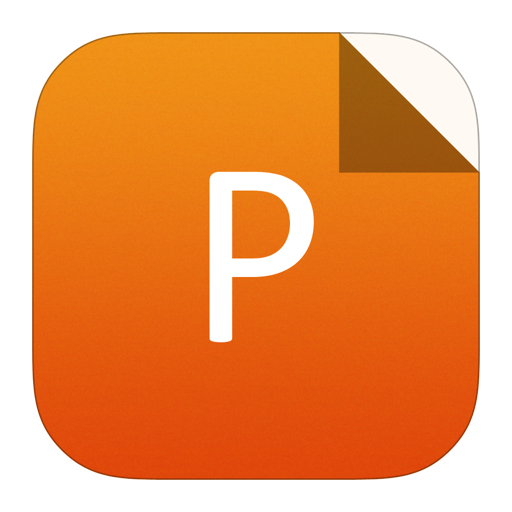
PowerPoint slide
Figure1.
(Color online) (a) Crystal structure of bulk CrI2, and spin-polarized band structure and spin spatial distribution of (b) ferromagnetic and (c) antiferromagnetic orderings.
3.2
CrI2 monolayer
CrI2 monolayer is cleaved from a fully relaxed bulk crystal. Each chromium atom is bonded to six iodine atoms in the monolayer, and all the atoms are arranged in the 1T phase. Noticing the similarity of CrI2 monolayer to the famous transitional metal dichalcogenide monolayers, it is necessary to consider the 2H phase. The 1T and 2H phases of CrI2 monolayers are illustrated in Fig. 2.

class="figure_img" id="Figure2"/>
Download
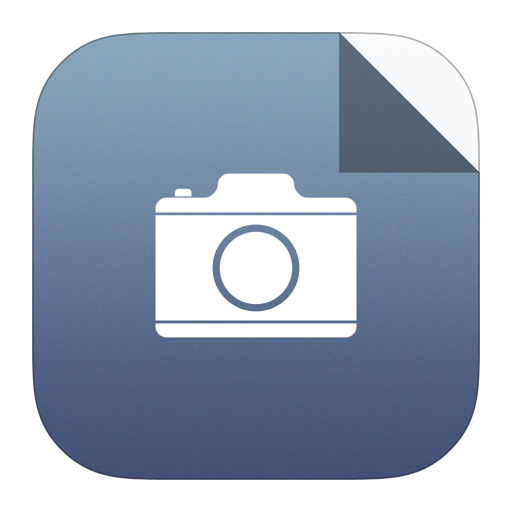
Larger image
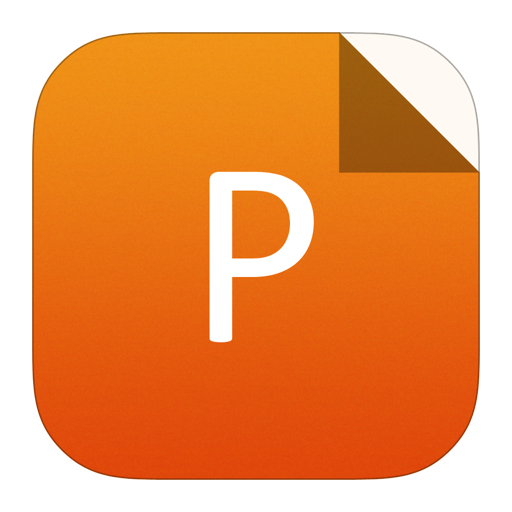
PowerPoint slide
Figure2.
(Color online) (a) 1T and (b) 2H phases of CrI2 monolayers. The chromium and iodine atoms are illustrated with blue and purple spheres, respectively.
In Fig. 3, the spin-resolved band structures, the total spin density of states (DOS) and spin spatial distributions of 1T and 2H CrI2 monolayers are shown. 2 × 1 × 1 supercells are also employed, to determine the magnetic ground states, by performing constrained spin-polarized first-principles calculations. The numerical results for 1T-FM, 1T-AFM, 2H-FM, 2H-AFM are displayed in panels Figs. 3(a)–3(d) accordingly, and the total energies for each configuration are listed in Table 1. One can find from Table 1 the most energy-favored configuration is 1T-AFM, which implies the ground state of monolayer CrI2 is antiferromagnetic.
Parameter | FM (eV) | AFM (eV) |
1T | –26.464 | –26.467 |
2H | –25.065 | –25.148 |
Table1.
The total energies of 1T and 2H phases.
Table options
-->
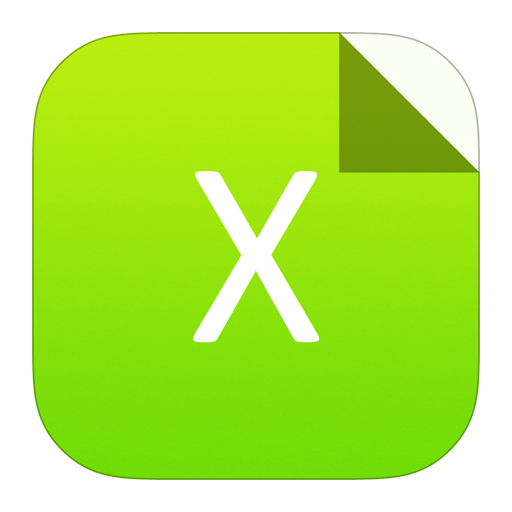
Download as CSV
Parameter | FM (eV) | AFM (eV) |
1T | –26.464 | –26.467 |
2H | –25.065 | –25.148 |

class="figure_img" id="Figure3"/>
Download
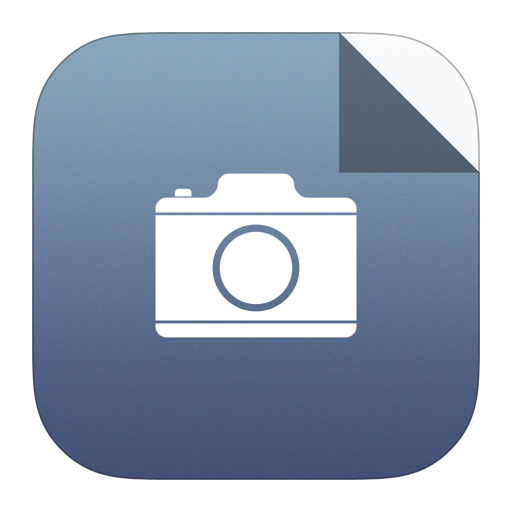
Larger image
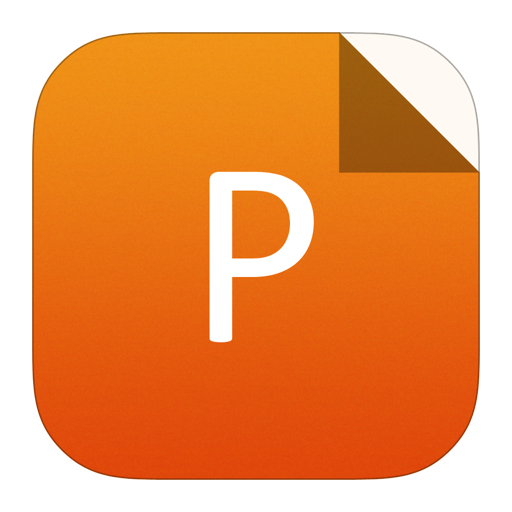
PowerPoint slide
Figure3.
(Color online) The spin-resolved band structures, total spin density of states (DOS) and spin spatial distributions of (a) 1T-FM, (b) 1T-AFM, (c) 2H-FM, and (d) 2H-AFM.
Table 1 shows the total energies of the FM state and the AFM state of the 1T phase, where the energy differences between the FM and the AFM states are obtained by performing DFT+U calculations within Dudarev’s approach. The Ueff (U–J) varies from 1 to 4 eV, and the corresponding total energy differences are of 30 meV, which indicates the AFM state is more energetic favorable.
3.3
CrI2 bilayer
To investigate the magnetic effect of the interlayer interaction, we construct a CrI2 bilayer from 1T CrI2 monolayers, and to clarify the influence of stacking patterns, both AB stacking and AA stacking models are constructed. As shown in Fig. 4, we denote these models, for convenience, as AA-FM, AA-AFM-a, AA-AFM-f, AB-FM, AB-AFM-a and AB-AFM-f, where the appendix a (f) indicates that the intra-layer initial magnetic moment is set antiferromagnetic (ferromagnetic). The interlayer distance between the adjacent chromium layers in equilibrium is obtained by relaxation and reads XX for the AA stacking and XX for the AB stacking, respectively.

class="figure_img" id="Figure4"/>
Download
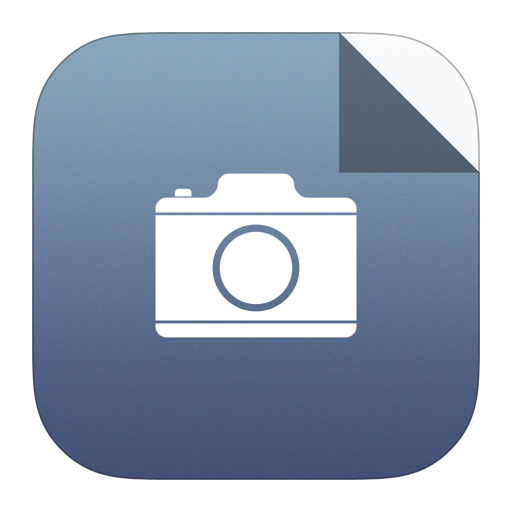
Larger image
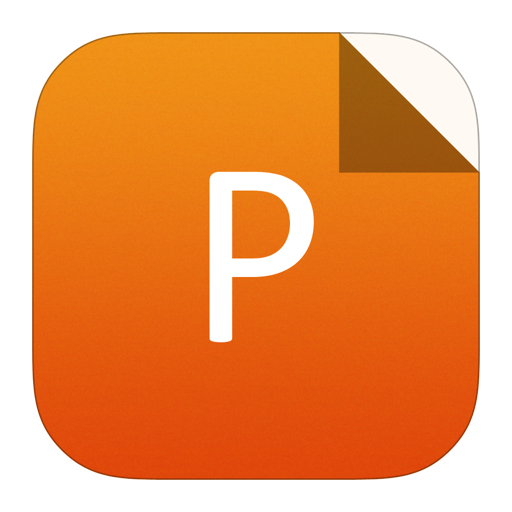
PowerPoint slide
Figure4.
(Color online) Configurations of the model (a) AA-FM, (b) AA-AFM-a, (c) AA-AFM-f, (d) AB-FM, (e) AB-AFM-a, and (f) AB-AFM-f. The up and down arrows indicate the signs of initial magnetic moments in constrained DFT calculations.
The spin-resolved band structures, total spin density of states and spin spatial distributions of each CrI2 bilayers are shown in Fig. 5. The total energies for each configuration are listed in Table 2. From Table 3, one can find the ground states of CrI2 bilayers are always antiferromagnetic independent of the stacking patterns, which implies the interlayer interaction is still antiferromagnetic in CrI2 up to few layers.
J (eV) | U (eV) | |||
2 | 3 | 4 | ||
0.9 | AFM | –25.417 | –24.519 | –23.560 |
FM | –25.361 | –24.416 | –23.525 | |
$left| {Delta E} ight|$ | 0.056 | 0.103 | 0.035 | |
0.5 | AFM | –25.031 | –24.092 | –23.217 |
FM | –24.976 | –24.053 | –23.184 | |
$left| {Delta E} ight|$ | 0.055 | 0.039 | 0.033 | |
0.1 | AFM | –24.679 | –23.735 | –22.883 |
FM | –24.600 | –23.699 | –22.851 | |
$left| {Delta E} ight|$ | 0.079 | 0.036 | 0.032 |
Table2.
The DFT+U total energies and energy difference for the 1T phase AFM and FM state.
Table options
-->
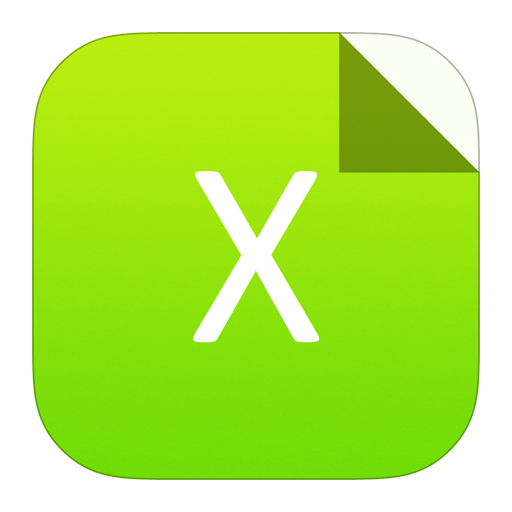
Download as CSV
J (eV) | U (eV) | |||
2 | 3 | 4 | ||
0.9 | AFM | –25.417 | –24.519 | –23.560 |
FM | –25.361 | –24.416 | –23.525 | |
$left| {Delta E} ight|$ | 0.056 | 0.103 | 0.035 | |
0.5 | AFM | –25.031 | –24.092 | –23.217 |
FM | –24.976 | –24.053 | –23.184 | |
$left| {Delta E} ight|$ | 0.055 | 0.039 | 0.033 | |
0.1 | AFM | –24.679 | –23.735 | –22.883 |
FM | –24.600 | –23.699 | –22.851 | |
$left| {Delta E} ight|$ | 0.079 | 0.036 | 0.032 |
Parameter | FM (eV) | AFM-a (eV) | AFM-f (eV) |
AA | –52.277 | –52.333 | –52.271 |
AB | –52.890 | –52.935 | –52.926 |
Table3.
AA and AB total energies.
Table options
-->
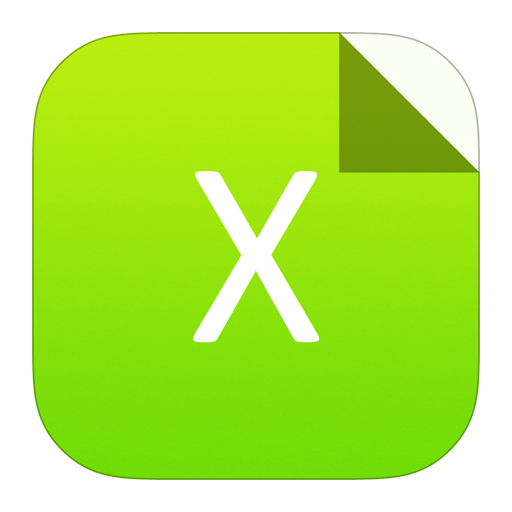
Download as CSV
Parameter | FM (eV) | AFM-a (eV) | AFM-f (eV) |
AA | –52.277 | –52.333 | –52.271 |
AB | –52.890 | –52.935 | –52.926 |

class="figure_img" id="Figure5"/>
Download
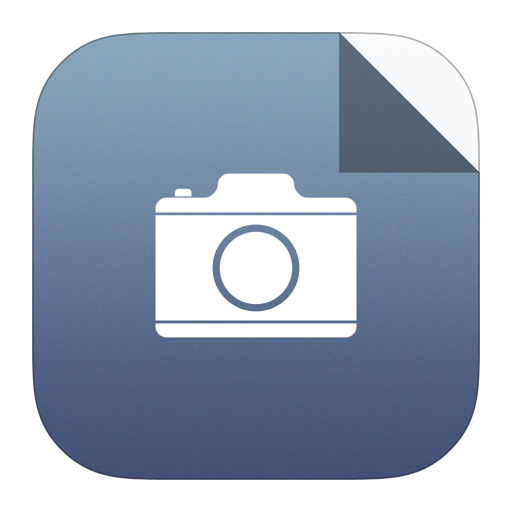
Larger image
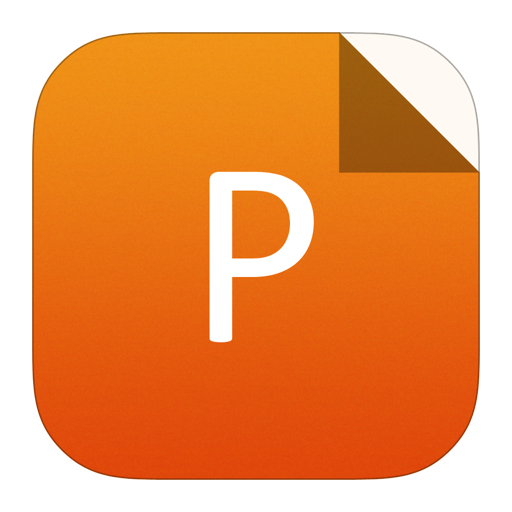
PowerPoint slide
Figure5.
(Color online) (The spin-resolved band structures, total spin density of states (DOS) and spin spatial distributions of model (a) AA-FM, (b) AA-AFM-a, (c) AA-AFM-f, (d) AB-FM, (e) AB-AFM-a, and (f) AB-AFM-f.
4.
Conclusion
In summary, we investigate both electronic structures and magnetic orderings of bulk and monolayer forms of chromium diiodides by first-principles calculations and demonstrate that the ground state of a free-standing monolayer of chromium diiodides is antiferromagnetic, even though the bulk possesses macroscopic ferromagnetic ordering. The stable CrI2 monolayer takes a 1T-phase configuration and the interlayer interaction remains antiferromagnetic up to few-layer scenarios. The robust antiferromagnetic feature makes monolayer or few layers CrI2 possible blocks to build flexible 2D antiferromagnetic spintronic devices. The questions of why the magnetic structures of monolayer and bulk CrI2 are opposite and how dependent antiferromagnetic interlayer interaction depend on the layer numbers are still to be answered.
Acknowledgements
This work was supported by the National Natural Science Foundation of China (No. 11404043) and Graduate Research Innovation Project of Chongqing (No. CYS18253).